Abstract
Analogs of pralidoxime, which is a commercial antidote for intoxication from neurotoxic organophosphorus compounds, were designed, synthesized, characterized, and tested as potential inhibitors or reactivators of acetylcholinesterase (AChE) using the Ellman’s test, nuclear magnetic resonance, and molecular modeling. These analogs include 1-methylpyridine-2-carboxaldehyde hydrazone, 1-methylpyridine-2-carboxaldehyde guanylhydrazone, and six other guanylhydrazones obtained from different benzaldehydes. The results indicate that all compounds are weak AChE reactivators but relatively good AChE inhibitors. The most effective AChE inhibitor discovered was the guanylhydrazone derived from 2,4-dinitrobenzaldehyde and was compared with tacrine, displaying similar activity to this reference material. These results indicate that guanylhydrazones as well as future similar derivatives may function as drugs for the treatment of Alzheimer's disease.
Introduction
Acetylcholinesterase (AChE) is a very important enzyme used to control transmission between neuronsCitation1,Citation2, when the process is either mediated or modulated by the neurotransmitter acetylcholine (ACh). ACh is released by the axon terminal or varicosities of the transmitter neuron into the extracellular spa ce to interact with the receptors of the other neuron. To maintain control of neurotransmission, it is necessary for AChE, after ACh executes its function, to catalyze ACh hydrolysis, converting ACh to choline (Ch) and acetate (Ac). After ACh hydrolysis, Ch is re-absorbed by the axon terminal to produce more ACh. If AChE is inhibited in the central nervous system, the concentration of ACh increases in the synaptic cleft, leading to cholinergic crisis, which affords several dangerous effects, such as convulsion and respiratory problems, which could lead to death.
AChE can be inhibited with reversible and irreversible inhibitors. The irreversible inhibitors are basically neurotoxic organophosphorus compounds, normally used as pesticides, insecticides, and chemical warfare agentsCitation3,Citation4. These agents lead to the phosphorylation of Ser203, which is the most important active amino acid involved in ACh hydrolysis, at the active site of AChE, leading to complete inhibition of this enzymeCitation5. Despite the use of chemical warfare agents being prohibited by the United Nations, these agents constitute one of the greatest threats in the modern worldCitation6. In parallel, many organophosphorus pesticides are used by all countries for agricultural production, a process that sometimes causes several toxic problems. To reactivate inhibited and phosphorylated AChE, it is necessary to use agents with the appropriate capacity to execute a nucleophilic attack on the phosphorus atom bound to Ser203, leading to the reactivation of the function of this serine. The AChE reactivator agents are mainly cationic oximesCitation7, which are effective but, unfortunately, not appropriate against all neurotoxic organophosphorus agentsCitation8, thereby necessitating the development of new and more effective agents for the protection of all humanity.
Some reversible inhibitors of AChE have medical applications and are particularly important for the treatment of Alzheimer’s disease (AD). When people develop AD, their neurons degenerate, leading to the low production of neurotransmitters, a process that induces serious memory problems. In this case, the inhibition of AChE increases the concentration of ACh in synaptic clefts, improving the neurotransmission process and brain function. For this reason, AChE inhibitors are very important agents for the treatment of AD, but some of these inhibitors are toxic, such as tacrine, requiring the development of new agents. Interestingly, some AChE reactivators also display competitive inhibition of the enzymeCitation8, and the reversible inhibitor and AD drug galantamine protects animals from soman, sarin, and paraoxon intoxicationCitation9, suggesting that novel compounds may have dual application, for AD and organophosphorus intoxication.
Therefore, the development of new agents that interact with AChE as potential drugs for the treatment of AD or as reactivators of this enzyme for the detoxification of neurotoxic organophosphorus compounds is very important. This work describes the preparation of compounds with a certain similarity to pralidoxime and their evaluation as AChE inhibitors or reactivators using the Ellman’s test, nuclear magnetic resonance (NMR) and molecular docking.
Materials and methods
Chemistry
Solvents (ethyl alcohol 95%, diethyl ether, methanol, chloroform) were purchased from VETEC (Duque de Caxias, Brazil) (used with further purification), and reagents were purchased from Merck and Sigma-Aldrich (São Paulo, Brazil) (used without further purification). Reactions were monitored by TLC using DC-Alufolien Kieselgel 60 F254 (Merck, Darmstadt, Germany). The NMR spectra were determined on a Varian 600 MHz spectrometer, using dimethyl sulfoxide-d6 (DMSO-d6) as solvent and tetramethylsilane as internal standard. The infrared (IR) spectra were measured using a Spectrum 100 spectrometer. The absorption values are expressed in wave number, using inverse centimeters (cm−1) as units. The mass spectra were obtained on a Waters (Milford, MA) spectrometer, model Q-Tof micro, using positive mode detection (MS ES+) and acetonitrile as solvent. The IR, HRMS and NMR spectra are available in the Supplemental file.
Synthesis of the compounds
N-methylation method for pyridine-2-carboxaldehyde
2-Formyl-1-methylpyridinium iodide was prepared using 5.0 g pyridine-2-carboxaldehyde (0.05 mol) and CH3I (14 g) with stirring and heating under reflux for 2 h. The orange crystals formed were filtered under nitrogen and washed with dry diethyl ether.
Method of synthesis for 2-PAM (1)
The method for the preparation of 1-methylpyridine-2-oxime iodide (1) was developed in two stages. First prepared was the iodide of 2-formyl-1-methylpyridine. Then, the iodide of 2-formyl-1-methylpyridine (1.4 mol) diluted in ethanol was reacted with hydroxylamine (0.0014 mol) in the presence of pyridine (1 mL) as catalyst. This reaction was stirred and heated under reflux for 40 min. Afterwards, distilled water (1 mL) was added, and the product was filtered.
Pralidoxime (1)
Yield 26%; Yellow solid. mp: 212–214 °C (Lit. 224–225 °C)Citation10.
1H NMR (300 MHz, DMSO-d6) δ (ppm): 4.37 (s, 3H, CH3); 8.06 (ddd, J = 1.6; 7.6 e 6.3 Hz, 1H, Ar-H); 8.38 (dd, J = 1.4 e 8.1 Hz, 1H, Ar-H); 8.53 (bt, 7.9 Hz, 1H, Ar-H); 8.67 (s, 1H, H-C = N-OH); 8.97 (bd, 6.3 Hz, 1H, Ar-H); 13.10 (s, 1H, C = N-OH).
13C NMR (75 MHz, DMSO-d6) δ: 46.4 (CH3), 125.1 (CH), 127.2 (CH), 141.8 (CH), 145.0 (CH), 146.6 (CH), 147.5 (C).
For C7H9N2 calculated: (31.7%) C, (10.5%) N, (3.7%) H; found (31.9%) C, (10.4%) N, (3.4%) H.
MS (ESI) m/z 137.0.
General procedure for hydrazone (2)
The method for the preparation of 1-methylpyridine-2-hydrazone iodide (2) was developed in two stages. First prepared was the iodide of 2-formyl-1-methylpyridine. Then, the iodide of 2-formyl-1-methylpyridine (0.004 mol) diluted in methanol was reacted with hydrazine hydrate (0.004 mol) in the presence of HCl (0.6 M) as catalyst. This reaction was stirred and heated under reflux for 30 min and then was cooled to 5 °C and filtered. The red liquid filtrate was evaporated, and the product was recrystallized from diethyl ether.
1-Methylpyridine-2-carboxaldehyde hydrazone (2)
Yield 36%. Yellow solid. mp: 174–176 °C.
IR (cm−1): 3291 (amine N-H), 3119 (aromatic C-H), 1628-1505 (C = N and C = C), 1293 and 1247 (C-N), 780 (C-H out of plane (Di-1,2)).
1H NMR (300 MHz, DMSO-d6) δ (ppm): 4.17 (s, 3H, CH3); 7.65 (dt, J = 2.3 and 6.4 Hz, 1H, Ar-H); 7.89 (s, 1H, H-C = N-NH2); 8.22 (m, 2H, Ar-H); 8.67 (d, 6.3 Hz, 1H, Ar-H); 9.16 (s, 2H, C = N-NH2).
13C NMR (75 MHz, DMSO-d6) δ: 44.8 (CH3); 121.9 (CH), 122.6 (CH), 123.4 (CH), 142.5 (CH), 144.2 (CH), 151.2 (C).
For C7H10N3 calculated: (31.9%) C, (15.9%) N, (3.8%) H; found (32.0%) C, (15.4%) N, (3.8%) H.
HRMS (ESI) m/z: Calcd for C7H10N3 [M + H]+ 136.0869; found 136.0868.
General procedure for guanylhydrazones (3–12)
Aminoguanidine hydrochloride (1.4 mmol) dissolved in 20 mL of 95% ethanol, the corresponding aldehyde (1 mmol) and two drops of HCl (0.6 M) were added to a 50.0 mL round-bottom flask. The solution was stirred and heated under reflux. Evaporation of the solvent gave a precipitate that was solubilized in distilled water and extracted with dichloromethane (5 × 20 mL). The aqueous phase was evaporated, and the product was obtained as crystals. The crystals were recrystallized from ethanol.
1-Methylpyridine-2-carboxaldehyde guanylhydrazone (3)
Yield 44%. Yellow solid. mp: 220–221 °C.
IR (cm−1): 3294 (amine N-H), 3086 (aromatic C-H), 1679-1509 (C = N and C = C), 1290 and 1251 (C-N), 782 (C-H out of plane (Di-1,2)).
1H NMR (300 MHz, DMSO-d6) δ (ppm): 4.41 (s, 3H, CH3); 8.19 (t, J = 6.1 Hz, 1H, Ar-H); 8.37 (s, 4H, C = NHCN2H4); 8.70 (t, J = 7.9 Hz, 1H, Ar-H); 8.75 (s, 1H, HC = N); 9.06 (d, J = 7.9 Hz, 1H, Ar-H); 9.13 (d, J = 6.1 Hz, 1H, Ar-H); 12.88 (s, 1H, C = NNH).
13C NMR (75 MHz, DMSO-d6) δ: 44.8 (CH3), 125.3 (CH), 127.5 (CH), 137.1 (CH), 144.6 (CH), 146.5 (CH), 147.6 (C), 155.3 (C).
For C8H12N5 calculated: (30.3%) C, (21.8%) N, (4.3%) H; found (28.1%) C, (20.5%) N, (3.8%) H.
HRMS (ESI) m/z: Calcd for C8H12N5 [M + H]+ 178.1087; found 178.1090.
Pyridine-2-carboxaldehyde guanylhydrazone (4)
Yield 45%. Yellow solid. mp: 213–215 °C (Lit. 190–192 °C)Citation11
IR (cm−1): 3460 (amine N-H); 3047(aromatic C-H), 1662-1465 (C = N and C = C), 1341 and 1174 (C-N), 770 (C-H out of plane (Di-1,2)).
1H NMR (300 MHz, DMSO-d6) δ (ppm): 7.79 (t, J = 5.2 Hz; 1H, Ar-H), 8.19 (s, 4H, C = NH-C-N2H4), 8.36 (m, 3H, HC = N and Ar-H), 8.77 (d, J = 5.2 Hz, 1H, Ar-H), 12.80 (s, 1H, C = NH-C-N2H4).
13C NMR (75 MHz, DMSO-d6) δ: 157.4 (C), 148.5 (CH); 146.8 (C), 144.2 (CH); 138.9 (CH); 128.8 (CH); 128.2 (CH).
For C7H10N5 calculated: (42.1%) C, (35.0%) N, (5.0%) H; found (50.3%) C, (41.7%) N, (5.1%) H.
HRMS (ESI) m/z: Calcd for C7H10N5 [M + H]+ 164.0920; found 164.0931.
Benzaldehyde guanylhydrazone (5)
Yield 65%. White solid. mp: 45–47 °C (Lit. 145–147 °C)Citation12.
IR (cm−1): 3316 (amine N-H), 3167 (aromatic C-H), 1676-1446 (C = N and C = C), 1229; 1156 (C-N), 748 and 686 (C-H out of plane).
1H NMR (300 MHz, DMSO-d6) δ (ppm): 7.43 (m, 3H, Ar-H); 7.85 (m, 5H, Ar-H and C = NH-CN2H3); 8.19 (s, 1H, HC = N); 12.16 (s, 1H, C = NH-CN2H3).
13C NMR (75 MHz, DMSO-d6) δ: 155.5 (C); 146.7 (CH), 133.4 (CH), 130.5 (C), 128.7 (CH), 127.6 (CH).
For C8H11N4 calculated: (48.3%) C, (28.2%) N, (5.6%) H; found (37.2%) C, (26.7%) N, (6.9%) H.
HRMS (ESI) m/z: Calcd for C8H11N4 [M + H]+ 163.0978; found 163.0881.
4-Nitrobenzaldehyde guanylhydrazone (6)
Yield 60%. Yellow solid. mp: 244–245 °C (Lit. 244–245 °C)Citation12.
IR (cm−1): 3272 (amine N-H), 3101 (aromatic C-H), 1676-1583 (C = N and C = C), 1510 and 1339 (NO2), 1231 (C-N), 835 (C-H out of plane (Di-1,4)).
1H NMR (300 MHz, DMSO-d6) δ (ppm): 8.01 (s, 4H, C = NH-CN2H4); 8.16 (d, J = 8.9 Hz, 2H, Ar-H); 8.27 (d, J = 8.9 Hz, 2H, Ar-H); 8.32 (s, 1H, HC = N); 12.48 (s, 1H, C = NH-CN2H4).
13C NMR (75 MHz, DMSO-d6) δ: 155.6 (C), 148.0 (C), 144.3 (C), 139.7 (CH), 128.5 (2CH), 123.7 (2CH).
For C8H10N5O2 calculated: (39.4%) C, (28.7%) N, (4.1%) H; found (37.6%) C, (27.1%) N, (4.2%) H.
HRMS (ESI) m/z: Calcd for C8H10N5O2 [M + H]+ 208.0834; found 208.0791.
4-Chlorobenzaldehyde guanylhydrazone (7)
Yield 94%. White solid. mp: 70 °C dp (Lit. 156–158 °C)Citation12.
IR (cm−1): 3251 (amine N-H), 3105 (aromatic C-H), 1678-1492 (C = N and C = C), 1276 (C-N), 832 (C-Cl), 750 (C-H out of plane (Di-1,4)).
1H NMR (300 MHz, DMSO-d6) δ (ppm): 7.89 (s, 4H, C = NH-CN2H4); 7.89 (d, J = 8.4 Hz, 2H, Ar-H); 7.48 (d, J = 8.4 Hz, 2H, Ar-H); 8.19 (s, 1H, HC = N); 12.26 (s, 1H, C = NH-CN2H4).
13C NMR (75 MHz, DMSO-d6) δ: 155.5 (C), 148.0 (C), 145.4 (CH), 132.4 (C), 129.2 (2CH), 128.7 (2CH).
For C8H10N4Cl calculated: (41.2%) C, (24.0%) N, (4.3%) H; found (39.5%) C, (23.1%) N, (5.1%) H.
HRMS (ESI) m/z: Calcd for C8H10N4Cl [M + H]+ 197.0594; found 197.0559.
4-Methylbenzaldehyde guanylhydrazone (8)
Yield 40%. White solid. mp: 155–156 °C (Lit. 170–171 °C)Citation13.
IR (cm−1): 3343 (amine N-H); 3153 (aromatic C-H), 1682-1483 (C = N and C = C), 1231 and 1178 (C-N), 810 (C-H out of plane (Di-1,4)).
1H NMR (300 MHz, DMSO-d6) δ (ppm): 2.34 (s, 3H, CH3); 7.25 (d, J = 8.4 Hz, 2H, Ar-H); 7.74 (d, J = 8.0 Hz, 2H, Ar-H); 7.76 (s, 4H, C = NH-CN2H4); 8.14 (s, 1H, HC = N); 12.06 (s, 1H, C = NH-CN2H4).
13C NMR (75 MHz, DMSO-d6) δ: 155.4 (C), 146.7 (CH), 140.3 (C), 130.7 (C), 129.2 (2CH), 127.5 (2CH), 21.0 (CH3).
For C9H13N4 calculated: (50.8%) C, (26.3%) N, (6.1%) H; found (52.0%) C, (27.1%) N, (6.5%) H.
HRMS (ESI) m/z: Calcd for C9H13N4 [M + H]+ 177.1140; found 177.1058.
4-Dimethylaminobenzaldehyde guanylhydrazone (9)
Yield 85%. Pale pink solid. mp: 212–214 °C (Lit. 179–182 °C)Citation13.
IR (cm−1): 3438 (amine N-H), 3100 (aromatic C-H), 1675-1530 (C = N and C = C), 1233 and 1181 (C-N), 818 (C-H out of plane (Di-1,4)).
1H NMR (300 MHz, DMSO-d6) δ (ppm): 2.98 (s, 6H, N(CH3)2); 7.68 (d, J = 8.8 Hz, 2H, Ar-H); 7.68 (s, 4H, C = NH-CN2H4); 6.86 (d, J = 8.8 Hz, 2H, Ar-H); 8.04 (s, 1H, HC = N); 11.89 (s, 1H, C = NH-CN2H4).
13C NMR (75 MHz, DMSO-d6) δ: 155.2 (C), 150.8 (C), 147.1 (CH), 128.9 (2CH), 122.4 (C), 112.8 (2CH), 40.4 (2CH3).
For C10H16N5 calculated: (49.7%) C, (28.9%) N, (6.7%) H; found (48.0%) C, (28.3%) N, (6.8%) H.
HRMS (ESI) m/z: Calcd for C10H16N5 [M + H]+ 206.1406; found 206.1357.
4-Hydroxybenzaldehyde guanylhydrazone (10)
Yield 70%. White solid. mp: 245–246 °C (Lit. 245–247 °C)Citation14.
IR (cm−1): 3425 (amine N-H), 3259 (O-H) 3102 (aromatic C-H), 1682-1515 (C = N and C = C), 1268 (C-O) 1216 (C-N), 832 (C-H out of plane (Di-1,4)).
1H NMR (300 MHz, DMSO-d6) δ (ppm): 6.83 (d, J = 8.6 Hz, 2H, Ar-H); 7.67 (s, 4H, C = NH-CN2H4); 7.67 (d, J = 8.6 Hz, 2H, Ar-H); 8.05 (s, 1H, HC = N); 10.09 (s, 1H, C = NH-CN2H4), 11.85 (s, 1H, OH).
13C NMR (75 MHz, DMSO-d6) δ: 159.8 (C), 155.2 (C), 146.9 (CH), 129.3 (CH), 124.3 (C), 115.5 (CH).
For C8H11N4O calculated: (44.7%) C, (26.1%) N, (5.1%) H; found (44.7%) C, (26.0%) N, (4.8%) H.
HRMS (ESI) m/z: Calcd for C8H11N4O [M + H] + 179.0933; found 179.0865.
2,4-Dinitrobenzaldehyde guanylhydrazone (11)
Yield 80%. White solid. mp: 190–191 °C.
IR (cm−1): 3386 (amine N-H), 3107 (aromatic C-H), 1685-1558 (C = N and C = C), 773 (NO2), 728 (C-H out of plane).
1H NMR (300 MHz, DMSO-d6) δ (ppm): 8.12 (s, 4H, C = NH-CN2H4); 8.50 (s, 1H, HC = N); 8.51 (d, J = 2,3 Hz; 1H, Ar-H); 8.73 (dd, J = 2.3 e 8.8 Hz; 1H, Ar-H); 8.76 (d, J = 8.8 Hz; 1H, Ar-H); 12.76 (s, 1H, C = NH-CN2H4).
13C NMR (75 MHz, DMSO-d6) δ: 155.5 (C), 147.8 (C), 147.5 (C), 140.6 (CH), 133.3 (C), 130.0 (CH), 127.2 (CH), 120.3 (CH).
MS (ESI) m/z 253.0.
2,4-Dichlorobenzaldehyde guanylhydrazone (12)
Yield 50%. White solid. mp: 246–248 °C (Lit. 222–223 °C)Citation13.
IR (cm−1): 3308 (amine N-H), 3143 (aromatic C-H), 1677-1519 (C = N and C = C), 823 (C-Cl), 775(C-H out of plane).
1H NMR (300 MHz, DMSO-d6) δ (ppm): 7.95 (s, 4H, C = NH-CN2H4); 8.50 (s, 1H, HC = N); 7.70 (d, J = 2.0 Hz; 1H, Ar-H); 7.51 (dd, J = 2.0 e 8.6 Hz; 1H, Ar-H); 8.33 (d, J = 8.6 Hz; 1H, Ar-H); 11.83 (s, 1H, C = NH-CN2H4).
13C NMR (75 MHz, DMSO-d6) δ: 155.3 (C), 141.5 (CH), 135.5 (C), 133.9 (C), 129.7 (C), 129.3 (CH), 128.9 (CH), 127.7 (CH).
For C8H9N4 calculated: (35.9%) C, (20.9%) N, (3.4%) H; found (33.0%) C, (19.1%) N, (3.9%) H.
MS (ESI) m/z 230.9.
Biological evaluation
AChE inhibition assay by Ellman’s method
Inhibition of AChE by guanylhydrazones and hydrazone was performed by Ellman’s colorimetric method modified for reading in a microplate spectrophotometer (SpectraMax 250, Molecular Devices, Sunnyvale, CA, USA) and at pH 7.4Citation15,Citation16. In this study, instead of using the homogenate of rat brain, enzyme purified from Electrophorus electricus was used. Equine serum butyrylcholinesterase was used to evaluate selectivity among the enzymes. To evaluate the inhibitory capacity of the compounds, a final volume of 200 µL was used and to a microplate were added 20 µL of cholinesterase (1 IU/mL), 5 μL of 5,5′-dithiobis-(2-nitrobenzoic acid) (DTNB) (0.01 M), 100 μL of inhibitor (200 μM) (final concentration of 100 μM), and 55 μL of pH 7.4 phosphate buffer (0.1 M). Inhibitors, DTNB, and acetylthiocholine iodide were dissolved in phosphate buffer, pH 7.4. After 10 min, 20 μL of acetylthiocholine iodide (0.005 M) was added. This analysis was performed at room temperature (22–25 °C) and followed at 412 nm for 5 min to determine reaction velocities, in triplicate. The compounds that showed inhibition above 50% were reevaluated at final concentrations of 3, 10, 30, 100, 300, and 1000 μM. The mean inhibitory concentration (IC50) was obtained by nonlinear regression with GraphPad Prism software.
AChE reactivation assay by Ellman’s method
For evaluating the reactivation of AChE by hydrazones, we used a final volume of 200 μL. To a microplate were added 20 μL of E. electricus AChE (1 IU/mL), 5 μL of DTNB solution, 100 μL of a solution of ethyl paraoxon 200 nM (final concentration of 100 nM) and 35 μL of pH 7.4 phosphate buffer. After 1 h, the time required for enzyme inhibition (established in preliminary experiments), 20 μL of hydrazones (final concentration in plate 10 µM, 100 µM, and 500 µM) were added, and after 20, 30, and 60 min (to allow the reactivation of the enzyme), 20 μL of acetylthiocholine iodide (0.005 M) was added at the moment that sample was read in the spectrophotometer, i.e. when then the enzymatic reaction is triggered. The analysis was performed in a spectrophotometer at 412 nm in triplicate and reagents were used at room temperature.
AChE inhibition assay by the NMR method
The EeAChE activity and inhibition determination by NMR was performed on a 600 MHz spectrometer using 5-mm NMR tubes, as described in our previous article on this topicCitation17. To determine EeAChE activity, 2 µL of its solution of 0.2 µM concentration in pH 7.4 phosphate buffer with D2O and in the presence of 1% bovine serum albumin was used. At the starting time of the reaction, this solution was mixed with 30 µL of acetylcholine (0.1 M) in the same solvent, and the complete mixture was diluted to a volume of 600 µL with the D2O pH 7.4 phosphate buffer in the 5-mm NMR tube. This sample was immediately introduced to the magnet for locking and shimming, allowing for the observation of the first 1H spectra exactly 5 min after the introduction of acetylcholine. All of the next 1H spectra were obtained every 5 min with a single scan over 80 min. The intensity of the methyl signals of acetylcholine (2.24 ppm) and acetic acid (2.16 ppm) was determined by scanning, affording information about EeAChE kinetics. The AChE inhibition test was executed with the same procedure, including the addition of 5 µL of each potential EeAChE inhibitor (12 mmol), always before the addition of acetylcholine, using exactly the same time periods. The concentration of acetylcholine and Ac from each 1H spectrum was determined by the integration of their methyl signals, using exactly the same integration length in all cases. The time used for the pure enzyme to afford 50% of Ac was also used to determine the respective Ac production (%) in the presence of the tested inhibitors. The comparison of the Ac concentration at this time with the pure AChE allows the determination of the percent inhibition of each tested compound. These results were obtained in triplicate.
Molecular modeling
The structure conformation, dipole moment, and determination of atomic electronic charges were obtained with Spartan’06 using the B3LYP system and the 6–311++G** basis setCitation18.
Docking
The ligands’ three-dimensional structures were built using the Spartan’10 programCitation19, and the conformer distribution with molecular mechanics using 100 conformers were examined. Their partial atomic charges were calculated using the RM1 semi-empirical method, and the molecular energies were calculated using Hartree-Fock 6–31 G*. The ligands’ rotatable bonds and atomic charges were defined. The 3D coordinates of TcAchE (PDB Code: 1ACJ) were obtained from the Protein Data BankCitation20. AutoDock 4.2Citation21 was used for the docking study of the ligands in the active site of TcAchE. To validate the docking protocol, we first performed the docking simulation of tacrine against the active site of TcAchE and compared it to the crystallographic structure. The interactions of ligands with the TcAChE active site were performed using AutoDock 4.2, using each ligand with 50 poses and grid points of 48 × 38 × 42 with 0.375 Å spacing. The best conformation of each ligand was selected according to the evaluation of the lowest energy of interaction with the enzyme. The figures were generated using a PyMOL Molecular Graphics SystemCitation22, AutoDock Tools and Python Molecular Viewer (ADT/PMV/Viewer)Citation23.
Results and discussion
Synthetic aspects
One previous study using molecular modeling with ab initio methods suggested the evaluation of anionic nucleophiles different from oximates, for example hydrazonates, as AChE reactivatorsCitation24. According to this molecular modeling study, we decided to prepare a hydrazone with a structure similar to pralidoxime to test its capacity as an AChE reactivator or inhibitor. Because hydrazones normally display low acidity, the binding of hydrazonates in the active site of AChE may be relatively difficult, indicating that these compounds would not be very effective reactivators of this enzyme when inhibited by organophosphorus agents. However, other hydrazones could display more acidity than the NH2 group but certainly would be less acidic than the respective oximes. To test this possibility, the only hydrazone selected for preparation and evaluation was 1-methylpyridine-2-carboxaldehyde hydrazone (2) (), with a structure very similar to pralidoxime (2-PAM, 1), which is one of the simplest cationic oximes used commercially for the reactivation of phosphorylated AChE and which certainly interacts with the active site of this enzyme.
Figure 1. Preparation of pralidoxime (2-PAM) (1); 1-methylpyridine-2-carboxaldehyde hydrazone (2); 1-methylpyridine-2-carboxaldehyde guanylhydrazone (3), pyridine-2-carboxaldehyde guanylhydrazone (4), benzaldehyde guanylhydrazone (5), 4-nitrobenzaldehyde guanylhydrazone (6), 4-chlorobenzaldehyde guanylhydrazone (7), 4-methylbenzaldehyde guanylhydrazone (8), 4-dimethylaminobenzaldehyde guanylhydrazone (9), 4-hydroxybenzaldehyde guanylhydrazone (10).
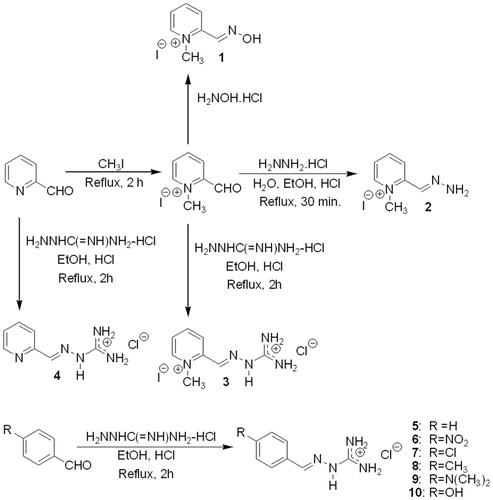
Considering the possibility that other cationic or neutral compounds similar to pralidoxime could interact well with the AChE active site and with inhibition capacity, we decided to prepare and test the compounds 1-methylpyridine-2-carboxaldehyde guanylhydrazone (3), pyridine-2-carboxaldehyde guanylhydrazone (4), benzaldehyde guanylhydrazone (5), 4-nitrobenzaldehyde guanylhydrazone (6), 4-chlorobenzaldehyde guanylhydrazone (7), 4-methylbenzaldehyde guanylhydrazone (8), 4-dimethylaminobenzaldehyde guanylhydrazone (9), and 4-hydroxybenzaldehyde guanylhydrazone (10), as shown in . Guanylhydrazones were selected because they contain one cationic site and therefore are appropriate to interact with the anionic region of the AChE active site and compete with acetylcholine. Several guanylhydrazones have been used as pharmacological agents for diverse diseases, such as cancerCitation25,Citation26, Chagas diseaseCitation27,Citation28, malaria and othersCitation29. These compounds were also studied by NMR and theoretical chemistryCitation30.
To determine if hydrazones could act as inhibitors or reactivators of AChE, the first step was the N-methylation of pyridine-2-carboxaldehyde using methyl iodide as solvent with stirring and under reflux for 2 h. This compound was used to prepare 2-PAM (1) in 26% yield by reaction with hydroxylamine hydrochloride in ethanol with pyridine under reflux for 40 min. The cationic hydrazone 2 was prepared by the reaction of N-methylpyridine-2-carboxaldehyde with hydrazine hydrochloride under reflux in 95% ethanol for 2 h, affording the compound in 36% yield. The N-methylpyridine-2-carboxaldehyde was also used to prepare the bis-cationic guanylhydrazone (3) by reaction with aminoguanidine hydrochloride in ethanol and HCl under reflux for 2 h, affording the compound in 44% yield. All other guanylhydrazones (4–10) were prepared by reaction of the respective aldehydes with aminoguanidine hydrochloride using 95% ethanol as solvent and heating under reflux for 2–7 h, leading to mono-cationic compounds obtained in 40–94% yields. All compounds were characterized by IR, NMR and mass spectrometry.
The only previous attempt to synthesize compound 2 was made in 1961 by Poziomek, who reported that at the end of the synthesis procedure, they obtained incomplete dehydration of the molecule and not the desired productCitation31. Our procedure led to the desired hydrazone after recrystallization from diethyl ether, and this compound has not been described in the literature. Compound 3 is a new unpublished agent. Compounds 4–10 have been described in the literatureCitation11,Citation12,Citation32,Citation33, but never for their application in AChE inhibition or reactivation, and thus, this is a new type of application for guanylhydrazones.
Assessment of inhibition of EeAChE
The tests of AChE inhibition with these compounds were carried out with two methods – the Ellman’s testCitation15,Citation16 and the recently published NMR methodCitation16 using tacrine as a reference standard. The selected AChE was from E. electricus (EeAChE), which displays an identical active site and very similar activity to that of the human enzymeCitation34,Citation35. We have also used the Ellman’s assay for EqBuChE inhibition, to assess the compounds’ selectivity. All results obtained for cholinesterase inhibition using the Ellman’s test and NMR are shown in .
Table 1. Results of inhibition of EeAChE and EqBuChE.
Analysis of the Ellman’s test indicates that all compounds display EeAChE inhibition activity, confirming that guanylhydrazones and hydrazones are potential inhibitors, especially because all contain cationic groups that are capable of interacting with the active site of this enzyme.
The most effective EeAChE inhibitors were 1-methylpyridine-2-carboxaldehyde hydrazone (2) (55.35%) and 4-nitrobenzaldehyde guanylhydrazone (6) (52.99%). It is observed that the two compounds containing a N-methylpyridine ring are effective (2 and 3), but compound 2, with a single cationic group (N-methylpyridine), is more effective than 3, which contains two cationic groups (guanidine and N-methylpyridine). However, pyridine-2-carboxaldehyde guanylhydrazone (4) is less effective (23.56%); its only cationic group is the guanidine. Furthermore, 2-PAM (1) displays a low effect (24.34%) similar to compound 4, despite containing an N-methylpyridine ring as a single cationic group. These results indicate that for the pyridine derivatives (1, 2, 3, and 4), the best situation is where the cationic N-methylpyridine ring is associated with a hydrazone group (2) and not with a guanylhydrazone group. However, as 4-nitrobenzaldehyde guanylhydrazone (6), the second most effective compound, shows, it is clear that guanylhydrazones not associated with pyridine rings could be effective.
The IC50 of the most active compounds at EeAChE from were 49.55 µM (compound 2) and 45.64 µM (compound 6). The inhibition curves are available in the Supplemental file. The EqBuChE assay showed that most compounds were not very selective, except for 2-PAM (1) and 3, which did not inhibit the enzyme at 100 µM.
To confirm the results obtained by the Ellman’s test, 1H NMR experiments were also carried outCitation17. The NMR method required a low concentration of enzyme (1.0 pM) in phosphate buffer at pH 7.4 and with the presence of 5% albumin to maintain the enzyme activity for a long time because EeAChE is a very fast enzyme in its hydrolysis of ACh. To perfectly control the time for each 1H NMR spectrum, it was also necessary to introduce the ACh into the 5-mm NMR tube as the last ingredient and then take 5 min to lock, gradient auto-shim and obtain the first spectra with one single transient. Continuing in this manner with additional spectra taken every 5.0 min led to the acquisition of 20 spectra during 100 min. The substrate (ACh) and product (Ac) concentrations from each spectrum were obtained from the integration of the corresponding methyl signals at 2.15 and 1.91 ppm, respectively. These experiments, which were executed first with pure EeAChE and followed with compounds 2–10, are shown in . In general terms, despite the results of both methods being similar, there are also important differences with some compounds. For example, the results for compound 5 are very similar (14.06 by Ellman’s and 13.9 by NMR), but for compound 9, the results are different (16.59 by Ellman and 10.4 by NMR). However, because the NMR test allows for perfect signal determination of the AChE substrate (ACh) and products (Ch and HOAc), as well as their correct concentration by integration, this method is as appropriate as the Ellman’s test; because the NMR method includes the structural determination of all agents, substrates and products, it is also a very good method.
Comparing the effects on AChE for the guanylhydrazones prepared from benzyl aldehydes in , it is observed that the compound containing one para-NO2 group (6) is the most effective, and the second most effective is compound 7, which is para-chlorinated. The third most effective compound is the para-methylated compound (8), and the less effective compounds are 5, which does not contain an R group, and 9 and 10, whose para-R groups, Me2N and HO, respectively, are electropositive. This information indicates that the best benzylidene guanylhydrazones for the inhibition of AChE should contain strong electron withdrawing R groups. To confirm this effect, all guanylhydrazones were studied by molecular modeling (B3LYP 6–311 + G**) to determine the correlation between some calculated properties of each compound and their percentage of AChE inhibition. In this case, the correlation of their dipole moment (Debye) with inhibition afforded an R2 of 0.9361, as shown in .
Figure 2. Correlation of the dipole moment calculated by molecular modeling with the AChE inhibition of compounds 5–10.
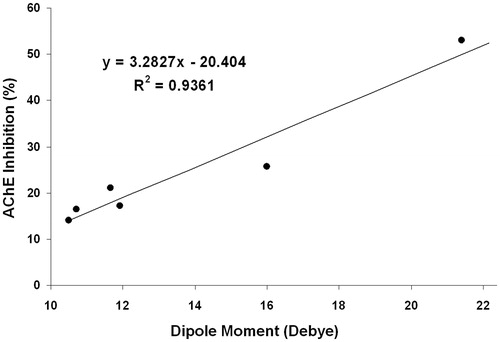
The correlation of the electronic charge of the nitrogen atom bound to the carbonyl aldehydes with AChE inhibition also afforded an R2 = 0.8310. These results confirm that the electron withdrawing characteristics of the R groups at the para-position on the benzene ring affect the AChE-inhibiting capacity of these guanylhydrazones.
To test this possibility after obtaining the previous results, the two compounds with more electronegative groups on the benzylidene ring, 2,4-dinitrobenzaldehyde guanylhydrazone (11) and 2,4-dichlorobenzaldehyde guanylhydrazone (12), were prepared and tested (). The most electronegative compound, 11, displayed EeAChE inhibition of 63.79% by the Ellman’s test, with an IC50 of 11.25 µM, and 75.6% by NMR, thus being the most effective compound, being superior to compound 2. Compound 11 was also more selective for EeAChE over EqBuChE. However, the bis-chlorinated compound, (12), which is less electronegative, affords 45.49% inhibition by the Ellman’s test and 41.3% inhibition by NMR, therefore being the fourth most effective inhibitor. In this case, tacrine (13) was used as the reference AChE inhibition agent.
Figure 3. Structure of the two selected more electronegative guanylhydrazones, 11 and 12, and tacrine (13).
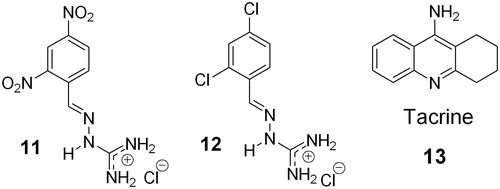
The results are shown in , and is the graphic of their NMR tests. The inclusion of the dipole moments of two additional compounds 11 and 12 with their AChE activities in the correlation graph of compounds 5–10 afforded an R2 of 0.9119. These results confirm that including more electronegative groups on the benzylidene ring increases the EeAChE inhibition capacity.
Figure 4. Kinetics and inhibition of EeAChE with benzaldehyde guanylhydrazone (5), 2,4-dinitrobenzaldehyde guanylhydrazone (11) and tacrine (13) by NMR.
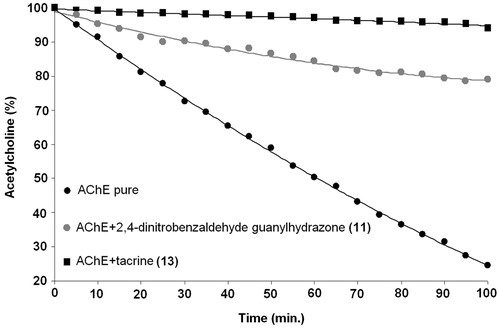
Table 2. Results of EeAChE inhibition with compounds 11, 12, and 13 by NMR and the Ellman’s test.
These results obtained by NMR are very similar to those obtained by the Ellman’s test, confirming their capacity for EeAChE inhibition. It is observed that 2,4-dinitrobenzaldehyde guanylhydrazone (11) displays a very efficient capacity to inhibit EeAChE (75.6%), indicating that other similar derivatives could be more effective and that guanylhydrazones and their analogs can be used as prototypes in the preparation of new drugs.
The correlation of AChE inhibition by the Ellman’s test with that using NMR, as shown in , indicate that these methods afford relatively similar results (R2 = 0.8914).
Molecular docking studies
To confirm this information, the interaction of compounds 2 and 6 in comparison with tacrine (13) was studied using AutoDock 4.2 softwareCitation21. Because the structure of E. electricus AChE (EeAChE) has not been reported as a complex with any ligand, we used the structure of Torpedo californica AChE (TcAChE), obtained from the Protein Data Bank under the code PDB ID:1ACJCitation20, for docking. To determine the effectiveness of AutoDock 4.2, the re-docking of tacrine in the TcAChE site was executed to compare with the reported X-ray crystallographic structure of this enzyme-tacrine complexCitation36. The root mean square deviation obtained for the re-docking of tacrine inside of the 3D structure of TcAChE was 0.53, confirming the effectiveness of AutoDock 4.2. After this, the docking of compounds 2, 6, 11, and 12 was performed, and their intermolecular binding energies were determined. The data obtained, in comparison with tacrine, are shown in .
Table 3. Docking results of compounds 2, 6, 11, 12, and tacrine with TcAChE.
These docking results indicate that these compounds are relatively effective as AChE inhibitors and demonstrate that compounds 2 and 12 have similar action but are less effective than tacrine. Despite the docking results being relatively different from the experimental results obtained by the Ellman and NMR tests ( and ), these results confirm that one hydrazone and several guanylhydrazones are potential AChE inhibitors. The superposition of the lowest energy poses of tacrine and compound 11 interacting in the active site of TcAChE is shown in . These results show that tacrine and compound 11, which is the most effective experimental AChE inhibitor of the tested compounds, interact with the active site of this enzyme, indicating that both display a very similar inhibitory capacity.
Figure 6. Interaction superposition of tacrine (13, green) and compound 11 (blue) in the active site of TcAChE. For references to color, see the online version of the article.
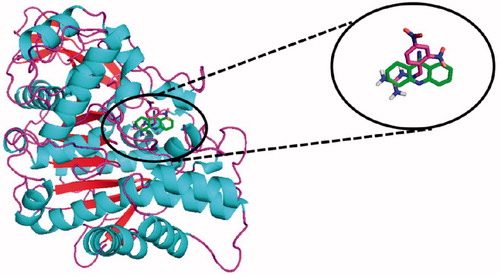
Despite the importance of docking studies, these results indicate that theoretical calculations usually are not in parallel with experimental information. The interaction of compounds 2, 6, 11, and tacrine (13) are shown in .
Figure 7. Docking interaction of tacrine (A), compounds 2 (B), 6 (C), and 11 (D) in the active site of TcAChE using carbon in magenta. H-bond distances in angstroms. For references to color, see the online version of the article.
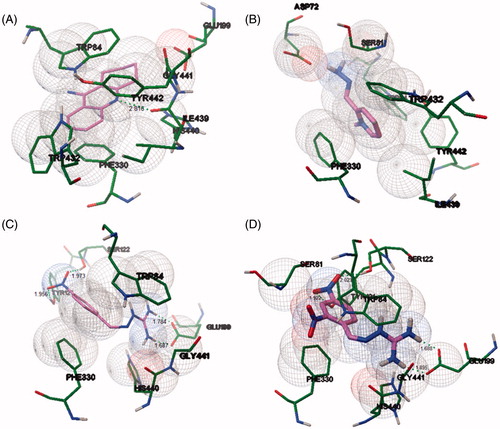
We have also performed a preliminary in silico screening of drug properties of the most active compounds, using the web tools Osiris Property Predictor (http://www.organic-chemistry.org/prog/peo/) and lazar (http://lazar.in-silico.ch/predict). Compounds 2, 6, and 11 all obey the rule-of-five principle and showed low toxicity risk, with confidence values below 0.25 in all assessments of carcinogenicity and mutagenicity. The pyridinium compounds, including pralidoxime (1) and 2, are not expected to permeate the blood-brain-barrier efficiently. However, compounds 6 and 11 showed CLogP of 0.11 and −0.81, respectively, which is compatible with brain penetration and eventual application in AD.
EeAChE reactivation
To perform the AChE reactivation tests, EeAChE was inhibited with paraoxon (100 nM) by the phosphorylation of Ser200. The complete inhibition of this enzyme was also confirmed by the Ellman’s test. To test the activity of these compounds, pralidoxime (2-PAM, 1) was used as a reference material. The Ellman’s tests for the reactivation of EeAChE inhibited with paraoxon confirmed that pralidoxime (1) is the only good reactivator, with the guanylhydrazones being ineffective, as shown in . As expected, 1 completely reactivated EeAChE, considering that it directly inhibited the enzyme by 24.3% at 100 µM (), so that the maximum expected activity in the reactivation assay would be 75.7%. Likewise, for compounds 2–12, only partial recovery of activity should be expected after reactivation, due to their own inhibitory properties.
Table 4. Ellman’s test results for the reactivation of AChE inhibited with paraoxon and incubated with the compounds for 20 min.
The reactivation of EeAChE inhibited with paraoxon was also assayed with incubation times of 30 and 60 min but did not show significant alteration of the results.
The structural modeling () indicated that the compounds can interact with the EeAChE active site. Thus, we expected that the nucleophilic groups NH2 of 2 and the substituent OH of 10 could be deprotonated by active site residues to attack the phosphate group bound to Ser200, leading to enzyme reactivation. Hydrazones are usually not acidic compounds, but compound 2, which contains the N-methyl group on the pyridine ring, is more acidic than average. Compound 3 also failed to reactivate, in spite of the cationic pyridine ring, which would also increase the acidity and nucleophilicity of the NH group. The expected reactivation mechanism for the cationic compounds 4–12 relied on deprotonation of their guanidine groups by active site anionic amino acid residues. This would lead to neutral intermediates with some nucleophilicity at the nitrogen atoms; however, the mechanism was not effective. These negative results are relevant for the design of new agents with more activity for defense against neurotoxic organophosphorus compounds.
Conclusions
Our test for the reactivation of paraoxon-inhibited AChE with 1-methylpyridine-2-carboxaldehyde hydrazone (2) indicates that in general hydrazones may be ineffective. This result suggests that for the reactivation of phosphorylated AChE, it is necessary to use better nucleophiles, especially compounds that could be easily deprotonated by the diverse basic groups of the amino acids in this enzyme.
The analysis of guanylhydrazones by the Ellman’s test indicates that they are also very poor AChE reactivators. However, these agents display interesting AChE inhibition capacity, being more effective when they possess electronegative groups on their benzylidene ring. The polarization of guanylhydrazones increases in the presence of electronegative groups, with the most effective being nitro groups, an effect that was confirmed by the Ellman’s test, NMR and molecular modeling. This condition indicates that guanylhydrazones with high polarization interact better with the active site of AChE. With these results, more effective AChE inhibitors are being designed as new potential agents for the treatment of AD, indicating that guanylhydrazones and analogs could be good effective agents for AD.
Supplementary material available online
Supplementaryinformation.pdf
Download PDF (1.4 MB)Acknowledgements
We would like to thank the following Brazilian funding agencies for financial support: INBEB, CAPES, CNPq and FAPERJfor the financial support.
Declaration of interest
The authors report no conflict of interest.
References
- Tang H, Wei Y-B, Zhang C, et al. Synthesis, biological evaluation and molecular modeling of oxoisoaporphine and oxoaporphine derivatives as new dual inhibitors of acetylcholinesterase/butyrylcholinesterase. Eur J Med Chem 2009;44:2523–32
- Taylor P, Radic Z. The cholinesterases: from genes to proteins. Annu Rev Pharmacol Toxicol 1994;34:281–320
- Chambers JE, Chambers HW, Meek EC, Pringle RB. Testing of novel brain-penetrating oxime reactivators of acetylcholinesterase inhibited by nerve agent surrogates. Chem Biol Interact 2013;203:135–8
- Delfino RT, Ribeiro TS, Figueroa-Villar JD. Organophosphorus compounds as chemical warfare agents: a review. J Braz Chem Soc 2009;20:407–28
- Gonçalves AS, França TCC, Figueroa-Villar JD, Pascutti PG. Molecular dynamics simulations and QM/MM studies of the reactivation by 2-PAM of tabun inhibited human acethylcolinesterase. J Braz Chem Soc 2011;22:155–65
- Ribeiro TS, Prates A, Alves SR, et al. The effect of neutral oximes on the reactivation of human acetylcholinesterase inhibited with paraoxon. J Braz Chem Soc 2012;23:1216–25
- Musilek K, Dolezal M, Gunn-Moore F, Kuca K. Design, evaluation and structure-activity relationship studies of the AChE reactivators against organophosphorus pesticides. Med Res Rev 2011;31:548–75
- Sepsova V, Karasova JZ, Korabecny J, et al. Oximes: inhibitors of human recombinant acetylcholinesterase. A structure-activity relationship (SAR) study. Int J Mol Sci 2013;14:16882–900
- Albuquerque EX, Pereira EFR, Aracava Y, et al. Effective countermeasure against poisoning by organophosphorus insecticides and nerve agents. Proc Natl Acad Sci USA 2006;103:13220–5
- Ginsburg S, Wilson IB. Oximes of pyridines in series. J Am Chem Soc 1957;79:481–5
- Valdés-Martínez J, Alstrum-Acevedo JH, Toscano RA, et al. Tetrachlorocuprate (II) salts of heterocyclic aminoguanidonium (guanylhydrazonium) íons. Polyhedron 2002;21:409–16
- Martins TLC, França TCC, Ramalho TC, Figueroa-Villar JD. Synthesis of guanylhydrazones under microwave irradiation. Synth Commun 2004;34:3891–9
- Ring JR, Zheng F, Haubner AJ, et al. Improving the inhibitory activity of arylidenaminoguanidine compounds at the N-methyl-D-aspartate receptor complex from a recursive computational-experimental structure–activity relationship study. Bioorg Med Chem 2013;21:1764–74
- Runti C. Stereoisomers and derivatives of dulcin. Annali Di Chimica 1956;46:417–27
- Ellman GL, Courtney KD, Andres V Jr, Featherstone RM. A new and rapid colorimetric determination of acetylcholinesterase activity. Biochem Pharmacol 1961;7:88–90
- Castro NG, Costa RS, Pimentel LSB, et al. CNS-selective noncompetitive cholinesterase inhibitors derived from the natural piperidine alkaloid (-)-spectaline. Eur J Pharmacol 2008;580:339–49
- Soares SFCX, Vieira AA, Delfino RT, Figueroa-Villar JD. NMR determination of Electrophorus electricus acetylcholinesterase inhibition and reactivation by neutral oximes. Bioorg Med Chem 2013;21:5923–30
- Shao Y, Molnar LF, Jung Y, et al. Advances in methods and algorithms in a modern quantum chemistry program package. Phys Chem Chem Phys 2006;8:3172–91
- Wavefuntion, Inc. Spartan’10, Irvine, CA, 2010
- Harel M, Schalk I, Ehret-Sabatier L, et al. Quaternary ligand binding to aromatic residues in the active-site gorge of acetylcholinesterase. Proc Natl Acad Sci USA 1993;90:9031–5
- Morris GM, Huey R, Lindstrom W, et al. AutoDock4 and autoDockTools4: automated docking with selective receptor flexibility. J Comput Chem 2009;30:2785–91
- Delano WL. DeLano Scientific, San Carlos, CA, 2008
- Sanner MF. Python: a programming language for software integration and development. J Mol Graph Model 1999;17:57–61
- Delfino RT, Figueroa-Villar JD. Nucleophilic reactivation of sarin-inhibited acetylcholinesterase: a molecular modeling study. J Phys Chem B 2009;113:8402–11
- LaFrate AL, Gunther JR, Carlson KE, Katzenellenbogen JA. Synthesis and biological evaluation of guanylhydrazone coactivator binding inhibitors for the estrogen receptor. Bioorg Med Chem 2008;16:10075–84
- Andreani A, Burnelli S, Granaiola M, et al. Synthesis and antitumor activity of guanylhydrazones from 6-(2,4-dichloro-5-nitrophenyl)imidazo[2,1-b]thiazoles and 6-pyridylimidazo[2,1-b]thiazoles(1). J Med Chem 2006;49:7897–901
- Borges MN, Messeder JC, Figueroa-Villar JD. Synthesis, anti-Trypanosoma cruzi activity and micelle interaction studies of bisguanylhydrazones analogous to pentamidine. Eur J Med Chem 2004;39:925–9
- Pacheco MGO, Silva CF, Souza EM, et al. Trypanosoma cruzi: activity of heterocyclic cationic molecules in vitro. Exp Parasitol 2009;123:73–80
- Specht S, Sarite SR, Hauber I, et al. The guanylhydrazone CNI-1493: an inhibitor with dual activity against malaria-inhibition of host cell pro-inflammatory cytokine release and parasitic deoxyhypusine synthase. Parasitol Res 2008;102:1177–84
- Martins TLC, Ramalho TC, Figueroa-Villar JD, et al. Theoretical and experimental 13C and 15N NMR investigation of guanylhydrazones in solution. Magn Reson Chem 2003;41:983–8
- Poziomek EJ, Kramer DN, Fromm BW, Mosher WAJ. Observations on the geometrical isomerism of formyl-1-methylpyridinium iodide oximes; carbinolamine intermediates. Org Chem 1961;26:423–7
- Foye WO, Almassian B, Eisenberg MS, Maher TJ. Synthesis and biological activity of guanylhydrazones of 2- and 4-pyridine and 4-quinoline carboxaldehydes. J Pharm Sci 1990;79:527–30
- Erkin AV, Krutikov VI. Modified synthesis of some 1-(pyrimidin-2-yl)-3-methyl-4-arylidenepyrazol-5(4H)-ones. Russ J Gen Chem 2008;78:301–4
- Ziemianin A, Ronco C, Dolé R, et al. Screening of new huprines-inhibitors of acetylcholinesterases by electrospray ionization ion trap mass spectrometry. J Pharm Biomed Anal 2012;70:1–5
- Simon S, Massoulié J. Cloning and Expression of acetylcholinesterase from Electrophorus. Splicing pattern of the 3′ exons in vivo and in transfected mammalian cells. J Biol Chem 1997;272:33045–55
- Leon R, de los Rios C, Marco-Contelles J, et al. New tacrine-dihydropyridine hybrids that inhibit acetylcholinesterase, calcium entry, and exhibit neuroprotection properties. Bioorg Med Chem 2008;16:7759–69