Abstract
A recombinant carbonic anhydrase (CA, EC 4.2.1.1) from the soil-dwelling bacterium Enterobacter sp. B13 was cloned and purified by Co2+ affinity chromatography. Bioinformatic analysis showed that the new enzyme (denominated here B13-CA) belongs to the β-class CAs and to possess 95% homology with the ortholog enzyme from Escherichia coli encoded by the can gene, whereas its sequence homology with the other such enzyme from E. coli (encoded by the cynT gene) was of 33%. B13-CA was characterized kinetically as a catalyst for carbon dioxide hydration to bicarbonate and protons. The enzyme shows a significant catalytic activity, with the following kinetic parameters at 20 °C and pH of 8.3: kcat of 4.8 × 105 s−1 and kcat/Km of 5.6 × 107 M−1 × s−1. This activity was potently inhibited by acetazolamide which showed a KI of 78.9 nM. Although only this compound was investigated for the moment as B13-CA inhibitor, further studies may reveal new classes of inhibitors/activators of this enzyme which may show biomedical or environmental applications, considering the posssible role of this enzyme in CaCO3 biomineralization processes.
Introduction
Carbonic anhydrases (CAs) [EC 4.2.1.1] are Zn(II)-dependent metalloenzymes which catalyze the reversible hydration of CO2 to and H+Citation1. CAs have important functions in the eukaryotes as well as in prokaryotes, such as pH regulation, biosynthetic reactions, respiration, CO2 transport, photosynthesis, among othersCitation2, and are known to be among the fastest enzymes described so far. Bacterial CAs have an essential role in the life cycle of these organisms, as well as in the regulation of calcium carbonate mineralization, at least in some bacterial species. Indeed, CaCO3 mineralization by bacteria is crucial for soil and ground water remediation, sequestration and capture of atmospheric CO2, as well as sand and soil strengthening and consolidationCitation3.
CAs are encoded by six evalutionary unrelated families, the α, β, γ, δ, ζCitation4 and η-CAsCitation5, which are present in organisms all over the phylogenetic tree. Among them, the α-, β-, γ- and δ-CAs are broadly distributed in many microorganismsCitation1, whereas the η-CAs were described only in protozoa for the momentCitation5.
To date, two different β-CAs have been reported in Escherichia coli, the most investigated bacterium for the molecular biology viewpoint. One such enzymes is encoded by the cynT gene and was the first β–CA identified in this bacterium, as a product of the cyn operon, being shown to possess sequence homology with higher plants β-CAs and to function in the process of cyanate utilization as a nitrogen source by this bacteriumCitation6,Citation7. The second one is can (previously called yadF), which has 30% amino acid sequence homology with CynTCitation8. It was reported that can is essential for the growth of E. coli under atmospheric CO2 conditionsCitation2. It has been also shown that expression of can is susceptible to the increase of cell density and to the rise in the medium temperature. In E. coli, CA activity is normally provided by the expression of both these enzymes, although detailed data regarding their localization within the bacterium are missing for the moment. In fact, bicarbonate/CO2 are necessary during the normal growth of this bacterium and for the biosynthesis of several biomolecules involved in vital functions. It has been suggested that can is conserved throughout Enterobacteriaceae genera (the type of bacteria to which E. coli also belongs)Citation9. However, only four β–CA genes have been identified in Enterobacteriaceae so far, i.e. cynT, can (previously yadF), cah and mig-5Citation9. Recently, β–CAs from two Enterobacter species were phylogenetically compared with the E. coli CAsCitation10, but only one of these enzymes has been investigated in detail for the moment, i.e. the one encoded by the cynT gene, for which the X-ray crystal structure was reported by Cronk et al.Citation8
Although ultimately there are many studies on various bacterial β–CAs, the investigation of Enterobacteriaceae is rather limited with only one E. coli enzyme being investigated in some detail, as mentioned above. Here, we describe a new β–CA from a bacterium belonging to this bacteria family, more precisely from an Enterobacter sp. B13 recently isolated from soil in Northern Turkey.
Materials and methods
Sampling site, culture conditions and microorganisms
The bacterial specimens were isolated from soil and mud samples which were collected from a riverside nearby Trabzon, Sürmene, Turkey. The samples were diluted with dH2O and filtrated. The filtrate inoculated in TSA plates and incubated at different temperatures. Selected colonies which grew at 37 °C were then inoculated to modified B-4 agar plates to monitor the CaCO3 decomposition properties of the grown bacteria. Among these isolates, clone B13 showed CO2 hydratase activityCitation11 and was subsequently used as a source of genomic DNA in this study.
Identification of the bacterial strain by 16S rRNA analysis
For identification of the strain, the 16S rRNA gene was amplified via PCR from genomic DNA with eubacterial universal primers and subsequently sequenced (Macrogen Inc., Seoul, Korea).
Cloning of Enterobacter sp. B13 can and construction of the recombinant expression vector
The genomic DNA of Enterobacter sp. was obtained with Wizard Genomic DNA Purification Kit (Promega, Madison, WI) and was used as a template for the CA gene. The amplification process was performed by PCR with two primers in order to amplify the Enterobacter sp. B13 can with NdeI (Biolab) and BamHI (Thermo Scientific, Waltham, MA) recognition sites (CANdeI; 5′-CCA TAT GAA CGA CAT AGA TAC-3″ and BamHI CAR5′-CGG ATC CTT ATT TAT GGT TNA CGT GC-3′) with the following conditions: initial denaturation steps at 95 °C for 3 min, denaturation at 95 °C for 45 s, followed by annealing at 51 °C for 1 min and primer extension at 72 °C for 1 min 20 s, followed by a step at 72 °C for 5 min, for 35 cycles. The PCR products were cloned into pGEM-T Easy vector (Promega) and sequenced (Macrogen Inc., Seoul, Korea). After the sequence verification, both the positive clone and the expression vector pET-15b (Novagen) were digested with NdeI-BamHI, then excised from the agarose gel (Qiaquick Gel Extraction Kit, Qiagen, Venlo, The Netherlands) and ligated (Thermo Scientific T4 Ligase, Thermo Scientific, Waltham, MA).
Construction and cloning of the recombinant plasmid was carried out in E. coli JM101 (Novagen, Podenzano, Italy) as described in the literatureCitation12. Transformants were checked by enzymatic digestion.
Overexpression in E. coli BL21 (DE3) pLysS and purification of the β-CA
The recombinant vector designed as pB13 can was transformed into E. coli BL21 (DE3)pLysS (Novagen) for overexpression and sequenced for correctness. A single colony, harboring the recombinant plasmid was chosen and cultured overnight in Luria Broth (LB) medium containing 50 μg/ml ampicillin. This culture was used to inoculate 200 ml LB-ampicillin medium and incubated at 37 °C with vigorous shaking. When OD600 reached the mid-log phase, ∼0.6–0.8, 0.5 mM ZnSO4 were added to the culture and expression was induced by 0.1 mM isopropyl β-D-thiogalactopyranoside (IPTG) for 24 h at 22 °C, for the production of recombinant B13-CA. Cells were then collected by centrifugation at 9000 rpm for 10 min at 4 °C. The pellet was resuspended in the lysis buffer (30 mM Tris–SO4 (pH 8.0), 0.1% Triton X-100, 200 mM NaCl, 20 mM imidazole and 0.2 mg/ml lysozyme) and sonicated. After centrifugation at 7000 rpm for 20 min at 4 °C, the supernatant containing N-terminally His-tagged protein was purified by Co2+ (TALON Metal Affinity Resin, Clontech), pre-equilibrated with the wash buffer (50 mM NaH2PO4 (pH 8.0), 0.1% Triton X-100, 300 mM NaCl and 20 mM imidazole). After transferring, the lysate was incubated in the His beads in 15 ml falcon tubes overnight at +4 °C. Following the two washing steps at pH 8.0 and one at pH 7.0 by gentle shaking at +4 °C, the CA was eluted with 250 mM imidazole in the same buffer (pH 7.0). SDS-PAGE (15%) was run to evaluate the purity of the protein. Purified B13-CA was dialyzed overnight in dialyis buffer (30 mM Tris–SO4, pH 8.0, 0.5 mM ZnSO4, 1 mM dithiothreitol-DTT) at +4 °C. After dialysis, the concentration of the protein was measured with both NanoDrop Spectrophotometer 2000 and at 595 nm, with bovine serum albumin (BSA) as a standard.
Zymography
Zymography analysis of the CA activity was performed as described in the literatureCitation13. To determine the esterase activity, zymogram staining was performed with minor modifications as described previouslyCitation14 at two different temperatures (+4 °C and also at RT) on SDS-PAGE (15%). In brief, followed by electrophoresis the gels were incubated in 100 mM Tris–HCl (pH 7.5) including 0.5% Triton X-100, for 6 h at +4 °C and for 4 h at RT. After incubation, the gels were rinsed in 100 mM Tris–HCl, containing 100 mM α-naphthyl acetate (α-NAc), dissolved in 2 ml acetone, and 100 mM Tris–HCl (pH 7.5) buffer containing 20 mg Fast Red Salt (two solutions were added to the gels at the same time) until the bands become visible.
Bioinformatic analysis
The nucleotide sequence of Enterobacter sp. B13 can was submitted to GeneBank under accession number KT184504. The identification of 16S rDNA sequence was determined by the EzTaxon identification tool (http://www.ezbiocloud.net/eztaxon) against 16S rRNA sequences found in the database, i.e. strains with validly published prokaryotic namesCitation15. Haemophilus influenzae ATCC was selected as outgroup member. Aamino acid resemblance was determined by using the DELTA-BLAST server (http://blast.ncbi.nlm.nih.gov/Blast.cgi).
For predicting the estimated molecular weight and to perform the multiple amino acid sequence alignment, Compute pI/Mw tool of ExPASyCitation16 and ClustalWCitation17 were used, respectively. Protein family and conserved domains were identified by Conserved Domain Search Database (http://www.ncbi.nlm.nih.gov/cdd). Determination of evolutionary familiarities was performed by Mega 6Citation18.
CO2 hydrase activity assay of B13-CA
An Applied Photophysics stopped-flow instrument was used for assaying the CA catalyzed CO2 hydration activityCitation19. Phenol red (at 0.2 mM) was used as indicator, working at the absorbance maximum of 557 nm with 10 mM TRIS (pH 8.3) as buffer and 0.1 M NaClO4 (for maintaining constant ionic strength), at 20 °C, following the CA-catalyzed CO2 hydration reaction for a period of 10–100 s (the uncatalyzed reaction needs around 60–100 s in the assay conditions, whereas the catalyzed ones are of around 6–10 s). The CO2 concentrations ranged from 1.7 to 17 mM for the determination of the kinetic parameters. The uncatalyzed rates were determined in the same manner and subtracted from the total observed rates. Enzyme concentrations in the assay system were about 16 nM for all the enzymes considered in the present study.
Results and discussion
16S rDNAsequence analysis and characterization of the bacteria
The result of the microbial growth on the B-4 agar plates led to the observation that the B13 bacterial isolate was effectively promoting CaCO3 precipitation, presumably due to the CA activity present in the bacterium (data not shown). The EzTaxon identification results of 16S rDNA sequence of the B13 strain showed that it possesses a high percentage similarity with bacteria belonging to Enterobacteriaceae. Among various such bacteria, strain B13 was 99.04% identical to Enterobacter xiangfangensis strain 10–17, 98.63% to Enterobacter asburiae strain JCM6051, 98.56% to Enterobacter cloacae strain ATCC 13047, 98.45% to Enterobacter cowanii CIP 107300, 98.41% to Klebsiella michiganensis W14, 98.23% to Escherichia hermannii GTC 347, 97.90% to Escherichia vulneris ATCC 33821, to 97.89% Citrobacter youngae CECT 5335, to 97.77% Enterobacter ludwigii EN-119T, 97.73% to Enterobacter soli LF7a, 97.67% to Cedecea davisae DSM 4568, 97.63% Cedecea neteri, 97.60% to Erwinia aphidicola GTC 1688, 97.59% to Enterobacter aerogenes KCTC 2190 ().
Purification of Enterobacter sp. B13-CA
The recombinant B13-CA was purified in concentrations ranging between 0.04 and 2.4 mg/ml from 250 ml cultures. The purified enzyme was subjected to SDS-PAGE analysis () which led to the observation of a distinct single band, with the predicted molecular mass of 24.95 kDa (deduced from the amino acid sequence). Data of the SDS-PAGE (, lane 2) showed that the purified B13-CA had indeed a molecular weight (for the monomer) of 25 kDa.
Biochemical characterization of Enterobacter sp. B13-CA
According to the zymogram analysis, Enterobacter sp. B13 CA did not reveal any esterase activity. Furthermore, no esterase activity was observed by using a spectrophotometric assay with α-naphthyl acetate as substrate (data not shown). This is to be expected, since esterase activity was not reported for any β-class CAs, although many putative activated esters have been investigated as possible substratesCitation20. In fact only the α-Citation20,Citation21 and η-CAsCitation22 possess esterase activity with activated esters (such as 4-nitrophenyl- or α-naphthyl acetate) as substrates.
Bioinformatic analysis
Considering the sequence of the can gene of Enterobacter sp. B13, which contains 663 bp, it encodes a protein of 220 amino acid residues. Based on DELTA-BLAST results of this amino acid sequence, Enterobacter sp. B13 can shows 33% homology with E. coli cynT and 95% with can (from the same bacterium, i.e. E. coli). Phylogenetic analysis of amino acid resemblance revealed that B13-CA is a member of the β-CA class and has a close relationship with can genes identified in other bacteria belonging to Enterobacteriaceae (). A conserved domain database (CDD) (http://www.ncbi.nlm.nih.gov/Structure/cdd/wrpsb.cgi) search of Enterobacter sp. B13 can sequences showed that the active and ion binding site residues of B13-CA are well conserved, as in all β-CAs investigated in detail, such as among others the enzymes from H. influenzae, Citrobacter freundii, Pseudomonas aeruginosa, Synechococcus elongatus, Brucella suis, E. coli, Methanothermobacter thermautotrophicus, Salmonella enterica, Pectobacterium carotovorum, Helicobacter pylori, Porphyromonas gingivalis, Vibrio cholerae, Streptococcus pneumoniae, S. mutans and Mycobacterium tuberculosis Rv1284Citation23–42 ().
Figure 3. Evolutionary relationship analysis, by the neighbor-joining method, of 21 known CAs from prokaryotes, in comparison to Enterobacter sp. B13-CA (accession numbers are given in parenthesis). Scale bar represents 0.05 substitutions per nucleotide position.
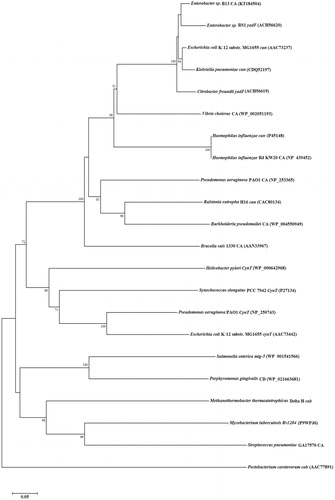
Figure 4. Multiple amino acid sequence alignment of 21 known β-class CAs: Enterobacter sp. B13-CA, E. coli str. K-12 substr. MG1655can, Ralstonia eutropha can, Enterobacter sp. RS1 yadF, Klebsiella pneumoniae can, H. influenzae can, C. freundii yadF, P. aeruginosa PAO1 cynT, P. aeruginosa PAO1 CA, Synechococcus elongates PCC 7942 icfA, B. suis 1330 CA, E. coli str. K-12 substr. MG1655 cynT, M. thermautotrophicus cab, S. enterica mig-5, P. carotovorum cah, H. pylori cynT, H. influenzae Rd KW20 CA, Burkholderia pseudomallei CA, P. gingivalis CD, V. cholerae CA, S. pneumoniae GA17570 CA and M. tuberculosis Rv1284. Triangles indicates the zinc ion-binding residues. Other active side conserved residues were shaded in blue (red letters). The figure was drawn with ESPript.
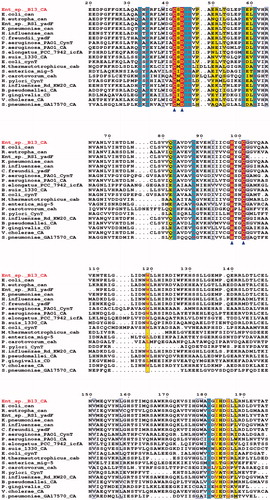
Catalytic activity of B13-CA
In fact there are two types of β-CAs in organisms all over the phylogenetic tree, which are defined by their pH–catalytic activity profile as well as active site structural configurationCitation41–52. Type I β-CAs display catalytic activity over a broad pH range (6.5–9.0) with the active site zinc tetrahedrally coordinated by three amino acid residues (two Cys and one His) and a hydroxide/water. In contrast, type II β-CAs are catalytically active only at a pH 8 and higher where they adopt a functional active site configuration like that of type I. In fact, below pH 8 they are conformationally self-inactivated by the addition of a fourth amino acid (an Asp residue) which coordinates to the Zn(II) ion as the fourth ligand, and displacing the zinc bound solventCitation39,Citation52. At pH >8, the Asp copordinated to Zn(II) makes a salt pair interaction with a conserved Arg residue, which “opens” the active site in the sense that a water molecule/hydroxide ion takes the place of the Asp residue, generating thus the nucleophile responsible for the catalytic activity of these enzymesCitation39,48–52.
As seen from , where an alignment of the amino acid sequence of B13-CA with that of other 20 bacterial β-CAs is presented, the new enzyme described here has all the features of a catalytically effective β-CA: (i) the putative Zn(II) ligands, Cys42, Asp44 (in case B13-CA is a type II β-CA), His 98 and Cys101; (ii) The catalytic dyad involved in activation of the zinc-coordinated water molecule/hydroxide ion for catalysisCitation53, represented by the Asp44–Arg46 residues, which resembles in a way the activation of the water molecule in aspartic proteasesCitation53.
As seen from , in which we compared the catalytic activity of the new enzyme reported here, B13-CA with that of other α- and β-class CAs from various organisms, B13-CA shows a significant activity as catalyst for the hydration of CO2 with formation of protons and bicarbonate. Indeed, B13-CA possesses the following kinetic paramaters at 20 °C and pH of 8.3: kcat of 4.8 × 105 s−Citation1 and kcat/Km of 5.6 × 107 M−Citation1 × s−Citation1. In fact the catalytic activity of the new enzyme is in the same range as those of other β-CAs, such as those from P. gingivalis (PgiCAb)Citation28, H. pylori (HpyCA)Citation35, or Legionella pneumophila (LpCA1 and LpCA2)Citation24, recently characterized by one of our groups. Furthermore, the enzyme was inhibited by the clinically usedCitation54–57 sulfonamide CA inhibitor (CAI) acetazolamide (AAZ) with an inhibition constant of 78.9 nM, in the same range as the human (h) hCA I isoform, HpyCA, LpCA1 and LpCA2 (which were inhibited with inhibition constants ranging between 40 and 250 nM). Only the physiologically dominant isoform hCA II was better inhibited by this drug, with a KI of 12 nM ().
Table 1. Kinetic parameters for the CO2 hydration reaction catalyzed by the human cytosolic isozymes hCA I and II (α-class CAs)Citation48 and the bacterial β-CAs: PgiCAb (from P. gingivalis)Citation28, HpyCA (from H. pylori)Citation35, LpCA1 and LpCA2 (from L. pneumophila)Citation24 and B13-CA (from Enterobacter sp. B13).
Conclusions
A recombinant CA (EC 4.2.1.1) from the soil-dwelling bacterium Enterobacter sp. B13 was cloned and purified by Co2+ affinity chromatography. Bioinformatic analysis showed the new enzyme (denominated here B13-CA) to belong to the β- CA class and to possess 95% homology with the ortholog enzyme from E. coli encoded by the can gene, whereas its sequence homology with the other such enzyme from E. coli (encoded by the cynT gene) was of 33%. B13-CA was characterized kinetically as a catalyst for carbon dioxide hydration to bicarbonate and protons. The enzyme showed a significant catalytic activity, with the following kinetic paramaters at 20 °C and pH of 8.3: kcat of 4.8 × 105 s−Citation1 and kcat/Km of 5.6 × 107 M−Citation1 × s−Citation1. This activity was potently inhibited by acetazolamide which showed a KI of 78.9 nM. Although only this compound was investigated for the moment as B13-CAI, further studies may reveal new classes of inhibitors/activators of this enzymeCitation58–60, which may show biomedical or environmental applications.
Declaration of interest
The authors do not have any conflicts of interest.
References
- Smith KS, Ferry JG. Prokaryotic carbonic anhydrases. FEMS Microbiol Rev 2000;24:335–66
- Hashimoto M, Kato JI. Indispensability of the Escherichia coli carbonic anhydrases YadF and CynT in cell proliferation at a low CO2 partial pressure. Biosci Biotechnol Biochem 2003;67:919–22
- Rodriguez-Navarro C, Jroundi F, Schiro M, et al. Influence of substrate mineralogy on bacterial mineralization of calcium carbonate: implications for stone conservation. Appl Environm Microbiol 2012;78:4017–29
- Capasso C, De Luca V, Carginale V, et al. Biochemical properties of a novel and highly thermostable bacterial α-carbonic anhydrase from Sulfurihydrogenibium yellowstonense YO3AOP1. J Enzyme Inhib Med Chem 2012;27:892–7
- Del Prete S, Vullo D, Fisher GM, et al. Discovery of a new family of carbonic anhydrases in the malaria pathogen Plasmodium falciparum—the η-carbonic anhydrases. Bioorg Med Chem Lett 2014;24:4389–96
- Sung YC, Fuchs JA. Characterization of the cyn operon in Escherichia coli K12. J Biol Chem 1988;263:14769–75
- Guilloton MB, Korte JJ, Lamblin AF, et al. Carbonic anhydrase in Escherichia coli. A product of the cyn operon. J Biol Chem 1992;267:3731–4
- Cronk JD, Endrizzi JA, Cronk MR, et al. Crystal structure of E. coli beta-carbonic anhydrase, an enzyme with an unusual pH-dependent activity. Protein Sci 2001;10:911–22
- Merlin C, Masters M, McAteer S, Coulson A. Why is carbonic anhydrase essential to Escherichia coli? J Bacteriol 2003;185:6415–24
- Ramanan R, Kannan K, Sivanesan SD, Chakrabarti T. Prevalence and phylogenetic relationship of two β-carbonic anhydrases in affiliates of Enterobacteriaceae. Ann Microbiol 2013;63:1275–82
- Chirică LC, Elleby B, Jonsson BH, Lindskog S. The complete sequence, expression in Escherichia coli, purification and some properties of carbonic anhydrase from Neisseria gonorrhoeae. Eur J Biochem 1997;244:755–60
- Joseph S, David WR. Molecular cloning: a laboratory manual. Cold Spring Harbor (NY): Cold Spring Harbor Laboratory Press; 2001:2–25
- Kotwica J, Ciuk MA, Joachimiak E, et al. Carbonic anhydrase activity in the vas deferens of the cotton leafworm–Spodoptera littoralis (Lepidoptera: Noctuidae) controlled by circadian clock. J Physiol Pharmacol 2006;57:107–23
- Eminoğlu A, Ülker S, Sandallı C. Cloning, purification and characterization of acetyl xylane esterase from Anoxybacillus flavithermus DSM 2641(T) with activity on low molecular-weight acetates. Protein J 2015;34:237–42
- Kim OS, Cho YJ, Lee K, et al. Introducing EzTaxon-e: a prokaryotic 16S rRNA gene sequence database with phylotypes that represent uncultured species. Int J Syst Evol Microbiol 2012;62:716–21
- Bjellqvist B, Hughes GJ, Pasquali C, et al. The focusing positions of polypeptides in immobilized pH gradients can be predicted from their amino acid sequences. Electrophoresis 1993;14:1023–31
- Thompson JD, Higgins DG, Gibson TJ. CLUSTAL W: improving the sensitivity of progressive multiple sequence alignment through sequence weighting, position-specific gap penalties and weight matrix choice. Nucleic Acids Res 1994;22:4673–80
- Tamura K, Stecher G, Peterson D, et al. MEGA6: molecular evolutionary genetics analysis version 6.0. Mol Biol Evol 2013;30:2725–9
- Khalifah RG. The carbon dioxide hydration activity of carbonic anhydrase. I. Stop-flow kinetic studies on the native human isoenzymes B and C. J Biol Chem 1971;246:2561–73
- Innocenti A, Scozzafava A, Parkkila S, et al. Investigations of the esterase, phosphatase and sulfatase activities of the cytosolic mammalian carbonic anhydrase isoforms I, II and XIII with 4-nitrophenyl esters as substrates. Bioorg Med Chem Lett 2008;18:2267–71
- Del Prete S, Vullo D, De Luca V, et al. Biochemical characterization of the δ-carbonic anhydrase from the marine diatom Thalassiosira weissflogii, TweCA. J Enzyme Inhib Med Chem 2014;29:906–11
- Krungkrai J, Krungkrai SR, Supuran CT. Carbonic anhydrase inhibitors: inhibition of Plasmodium falciparum carbonic anhydrase with aromatic/heterocyclic sulfonamides—in vitro and in vivo studies. Bioorg Med Chem Lett 2008;18:5466–71
- Vullo D, Del Prete S, Osman SM, et al. Sulfonamide inhibition studies of the gamma-carbonic anhydrase from the oral pathogen Porphyromonas gingivalis. Bioorg Med Chem Lett 2014;24:240–4
- Nishimori I, Vullo D, Minakuchi T, et al. Anion inhibition studies of two new β-carbonic anhydrases from the bacterial pathogen Legionella pneumophila. Bioorg Med Chem Lett 2014;24:1127–32
- Del Prete S, De Luca V, Scozzafava A, et al. Biochemical properties of a new α-carbonic anhydrase from the human pathogenic bacterium, Vibrio cholerae. J Enzyme Inhib Med Chem 2014;29:23–7
- Del Prete S, De Luca V, Vullo D, et al. Biochemical characterization of the γ-carbonic anhydrase from the oral pathogen Porphyromonas gingivalis, PgiCA. J Enzyme Inhib Med Chem 2014;29:532–7
- Del Prete S, Isik S, Vullo D, et al. DNA cloning, characterization, and inhibition studies of an alpha-carbonic anhydrase from the pathogenic bacterium Vibrio cholerae. J Med Chem 2012;55:10742–8
- Del Prete S, Vullo D, De Luca V, et al. Biochemical characterization of recombinant beta-carbonic anhydrase (PgiCAb) identified in the genome of the oral pathogenic bacterium Porphyromonas gingivalis. J Enzyme Inhib Med Chem 2015;30:366–70
- Supuran CT. Bacterial carbonic anhydrases as drug targets: toward novel antibiotics? Front Pharmacol 2011;2:34
- Vullo D, Nishimori I, Minakuchi T, et al. Inhibition studies with anions and small molecules of two novel beta-carbonic anhydrases from the bacterial pathogen Salmonella enterica serovar Typhimurium. Bioorg Med Chem Lett 2011;21:3591–5
- Joseph P, Ouahrani-Bettache S, Montero JL, et al. A new beta-carbonic anhydrase from Brucella suis, its cloning, characterization, and inhibition with sulfonamides and sulfamates, leading to impaired pathogen growth. Bioorg Med Chem 2011;19:1172–8
- Joseph P, Turtaut F, Ouahrani-Bettache S, et al. Cloning, characterization, and inhibition studies of a beta-carbonic anhydrase from Brucella suis. J Med Chem 2010;53:2277–85
- Nishimori I, Minakuchi T, Morimoto K, et al. Carbonic anhydrase inhibitors: DNA cloning and inhibition studies of the alpha-carbonic anhydrase from Helicobacter pylori, a new target for developing sulfonamide and sulfamate gastric drugs. J Med Chem 2006;49:2117–26
- Nishimori I, Onishi S, Takeuchi H, et al. The alpha and beta classes carbonic anhydrases from Helicobacter pylori as novel drug targets. Curr Pharm Des 2008;14:622–30
- Nishimori I, Minakuchi T, Kohsaki T, et al. Carbonic anhydrase inhibitors: the beta-carbonic anhydrase from Helicobacter pylori is a new target for sulfonamide and sulfamate inhibitors. Bioorg Med Chem Lett 2007;17:3585–94
- Burghout P, Vullo D, Scozzafava A, et al. Inhibition of the β-carbonic anhydrase from Streptococcus pneumoniae by inorganic anions and small molecules: toward innovative drug design of antiinfectives? Bioorg Med Chem 2011;19:243–8
- Guzel O, Maresca A, Scozzafava A, et al. Discovery of low nanomolar and subnanomolar inhibitors of the mycobacterial beta-carbonic anhydrases Rv1284 and Rv3273. J Med Chem 2009;52:4063–7
- Nishimori I, Minakuchi T, Maresca A, et al. The β-carbonic anhydrases from Mycobacterium tuberculosis as drug targets. Curr Pharm Des 2010;16:3300–9
- Suarez Covarrubias A, Larsson AM, Hogbom M, et al. Structure and function of carbonic anhydrases from Mycobacterium tuberculosis. J Biol Chem 2005;280:18782–9
- Carta F, Maresca A, Covarrubias AS, et al. Carbonic anhydrase inhibitors. Characterization and inhibition studies of the most active beta-carbonic anhydrase from Mycobacterium tuberculosis, Rv3588c. Bioorg Med Chem Lett 2009;19:6649–54
- Minakuchi T, Nishimori I, Vullo D, et al. Molecular cloning, characterization, and inhibition studies of the Rv1284 beta-carbonic anhydrase from Mycobacterium tuberculosis with sulfonamides and a sulfamate. J Med Chem 2009;52:2226–32
- Capasso C, Supuran CT. Sulfa and trimethoprim-like drugs—antimetabolites acting as carbonic anhydrase, dihydropteroate synthase and dihydrofolate reductase inhibitors. J Enzyme Inhib Med Chem 2014;29:379–87
- Migliardini F, De Luca V, Carginale V, et al. Biomimetic CO2 capture using a highly thermostable bacterial α-carbonic anhydrase immobilized on a polyurethane foam. J Enzyme Inhib Med Chem 2014;29:146–50
- Supuran CT, Capasso C. The η-class carbonic anhydrases as drug targets for antimalarial agents. Expert Opin Ther Targets 2015;19:551–63
- Capasso C, Supuran CT. An overview of the alpha-, beta- and gamma-carbonic anhydrases from Bacteria: can bacterial carbonic anhydrases shed new light on evolution of bacteria? J Enzyme Inhib Med Chem 2015;30:325–32
- Supuran CT. Carbonic anhydrase inhibitors: an editorial. Expert Opin Ther Pat 2013;23:677–79
- Capasso C, Supuran CT. Anti-infective carbonic anhydrase inhibitors: a patent and literature review. Expert Opin Ther Pat 2013;23:693–704
- Supuran CT. Carbonic anhydrases: novel therapeutic applications for inhibitors and activators. Nat Rev Drug Discov 2008;7:168–81
- De Luca V, Del Prete S, Supuran CT, Capasso C. Protonography, a new technique for the analysis of carbonic anhydrase activity. J Enzyme Inhib Med Chem 2015;30:277–82
- Supuran CT. Structure-based drug discovery of carbonic anhydrase inhibitors. J Enzyme Inhib Med Chem 2012;27:759–72
- Supuran CT. Carbonic anhydrases: from biomedical applications of the inhibitors and activators to biotechnological use for CO(2) capture. J Enzyme Inhib Med Chem 2013;28:229–30
- Pinard MA, Lotlikar SR, Boone CD, et al. Structure and inhibition studies of a type II beta-carbonic anhydrase psCA3 from Pseudomonas aeruginosa. Bioorg Med Chem 2015;23:4831–8
- Mastrolorenzo A, Rusconi S, Scozzafava A, et al. Inhibitors of HIV-1 protease: current state of the art 10 years after their introduction. From antiretroviral drugs to antifungal, antibacterial and antitumor agents based on aspartic protease inhibitors. Curr Med Chem 2007;14:2734–48
- Supuran CT. Carbonic anhydrase inhibition/activation: trip of a scientist around the world in the search of novel chemotypes and drug targets. Curr Pharm Des 2010;16:3233–45
- Supuran CT. Carbonic anhydrase inhibitors. Bioorg Med Chem Lett 2010;20:3467–74
- De Simone G, Alterio V, Supuran CT. Exploiting the hydrophobic and hydrophilic binding sites for designing carbonic anhydrase inhibitors. Expert Opin Drug Discov 2013;8:793–810
- Alterio V, Di Fiore A, D'Ambrosio K, et al. Multiple binding modes of inhibitors to carbonic anhydrases: how to design specific drugs targeting 15 different isoforms? Chem Rev 2012;112:4421–68
- Maresca A, Carta F, Vullo D, et al. Dithiocarbamates strongly inhibit the β-class carbonic anhydrases from Mycobacterium tuberculosis. J Enzyme Inhib Med Chem 2013;28:407–11
- Scozzafava A, Passaponti M, Supuran CT, Gulcin I. Carbonic anhydrase inhibitors: guaiacol and catechol derivatives effectively inhibit certain human carbonic anhydrase isoenzymes (hCA I, II, IX and XII). J Enzyme Inhib Med Chem 2015;30:586–91
- Alp C, Maresca A, Alp NA, et al. Secondary/tertiary benzenesulfonamides with inhibitory action against the cytosolic human carbonic anhydrase isoforms I and II. J Enzyme Inhib Med Chem 2013;28:294–8