Abstract
An amperometric biosensor based on tricobalt tetraoxide nanoparticles (Co3O4), graphene (GR), and chitosan (CS) nanocomposite modified glassy carbon electrode (GCE) for sensitive determination of glutamate was fabricated. Scanning electron microscopy was implemented to characterize morphology of the nanocomposite. The biosensor showed optimum response within 25 s at pH 7.5 and 37 °C, at +0.70 V. The linear working range of biosensor for glutamate was from 4.0 × 10−6 to 6.0 × 10−4 M with a detection limit of 2.0 × 10−6 M and sensitivity of 0.73 μA/mM or 7.37 μA/mMcm2. The relatively low Michaelis–Menten constant (1.09 mM) suggested enhanced enzyme affinity to glutamate. The glutamate biosensor lost 45% of its initial activity after three weeks.
Introduction
l-Glutamate is an amino acid, which plays an important role in food processing and clinical applications. It is an important neurotransmitter in the central nervous system and neuronal pathways in the brain which is related to several neurological disorders, such as epilepsy, stroke, Alzheimer’s and Parkinson’s diseases (Batra and Pundir Citation2013, Gholizadeh et al. Citation2012, Jamal et al. Citation2010). Moreover, its sodium salt, monosodium glutamate (MSG) is widely used as a flavor enhancing food additive and it has been associated with the Chinese restaurant syndrome. The excessive intake of MSG can cause allergic effects such as headache and stomach pain (Basu et al. Citation2006, Beyene et al. Citation2003). Therefore, developing sensitive and rapid methods is highly demanded and plays an important role in food industry and clinical analysis. Various methods are available for determination of glutamate like chromatography (Acuna and Trias Citation2009, Buck et al. Citation2009, Demirhan et al. Citation2015), spectrophotometry (Acebal et al. Citation2008) and fluorimetry (Tsukatani and Matsumoto Citation2005), electrophoresis (Tucci et al. Citation1998) and potentiometry (Gündüz et al. Citation1988). However, most of these methods are cumbersome, time consuming, and require expensive apparatus and skilled person to operate. Compared with other analytical methods, enzyme-based electrochemical biosensors possess simplicity of operation, high sensitivity, and selectivity. Different types of biosensors have been reported for detection of glutamate (Batra and Pundir Citation2013, Şimşek et al. Citation2016, Tian et al. Citation2009, Varma et al. Citation2006, Yu et al. Citation2011, Yılmaz and Karakuş Citation2011).
Graphene (GR) is a two dimensional layer of graphite with sp2 carbon atoms arranged in a hexagonal lattice (Abbasi et al. Citation2016). Because of the unique properties including fast electron transfer kinetics, excellent thermal conductivity, large surface area and good biocompatibility, GR and its derivatives have demonstrated attractive application in electrochemical sensing and biosensing (Lim et al. Citation2010, Liu et al. Citation2010, Pumera et al. Citation2010, Zhou et al. Citation2009). Metal and metal oxide nanoparticles have recently been investigated intensively in so many fields including the construction of biosensors being mainly due to their large surface-to-volume ratio, good electrical properties, high surface reaction activity, high catalytic efficiency, chemical stability and strong adsorption ability (Casero et al. Citation2014, Devi et al. Citation2013). Among different metal oxides, tricobalt tetraoxide nanoparticles (Co3O4) have been widely used in biosensors as they have advantages such as low cost, high reactivity, wide availability, and excellent electrocatalytic properties (Ali et al. Citation2015, Kaçar et al. Citation2014, Wang et al. Citation2011). Recent research interest has focused on the preparation of nanocomposites involving the combination of GR and metal or metal oxide nanoparticles. Various metal or metal oxide nanoparticles and GR composites such as Pd − graphene (Zeng et al. Citation2011), TiO2–reduced GR (Casero et al. Citation2014), NiO–carboxylic graphene (Yang et al. Citation2013), Au–graphene (Zhou et al. Citation2010) Pt–graphene (Sun et al. Citation2011) have been reported for electrochemical biosensing applications.
The aim of this work was to develop an amperometric biosensor for glutamate measurement that possesses properties such as low cost, good stability, and rapid response. The biosensor was constructed by immobilizing glutamate oxidase (GLOx) on a Co3O4/chitosan (CS)/GR composite film modified glassy carbon electrode (GCE). The experimental conditions related to the construction and the characterization of the biosensor were studied in detail. Optimum parameter studies such as enzyme loading, pH, and working potential were conducted. The analytical performance parameters of the biosensor including the linear range, detection limit, sensitivity, response time, repeatability, and stability were also described.
Experimental
Materials
GR solution (DRP-GPHSOL) (2 mg/mL) was obtained from Dropsense (Llanera, Spain). GLOx (E.C. 1.4.3.11 from Streptomyces sp.), Co3O4 nanoparticles (<50 nm particle size), potassium hexacyanoferrate(III), potassium hexacyanoferrate(II) trihydrate, l-glutamic acid monosodium salt, lysine, methionine, and glutaraldehyde were purchased from Sigma (St. Louis, MO, USA). Sodium monohydrogenphosphate and sodium dihydrogenphosphate were supplied from Riedel-de Haën (Seelze, Germany). CS (90% deacetylation, medium molecular weight) and aspartic acid were from Aldrich (Steinhem, Germany). Glucose was from Fluka (Buchs, Switzerland). All other chemicals were obtained from Merck (Darmstadt, Germany). Standard solutions of glutamate were prepared by dissolving glutamate in phosphate buffer solution.
Apparatus and measurements
Electrochemical measurements were performed with IVIUM electrochemical analyzer (Ivium Technologies, the Netherlands). A conventional three electrode system was used for all electrochemical measurements. Ag/AgCl electrode (BAS MF 2052) and platinium wire (BAS MW 1034) electrode were used as reference electrode and counter electrode, respectively. The working electrode was a modified GCE (diameter 3 mm). All measurements were carried out in a thermostated cell at 37 °C, containing 0.05 M phosphate buffer solution (PBS pH 7.5) under stirring at an applied potential of +0.70 V. The morphology of the modified electrodes was observed using scanning electron microscope (SEM) from Carl Zeiss AG, EVO® 50 Series. pH measurements were performed using a ORION Model 720A pH/ion meter and ORION combined pH electrode (Thermo Scientific, Waltham, MA). Double-distilled water from a Human Power I+, Ultra Pure Water System (ELGA PURELAB Option-S, London, UK) was used for the preparation of all aqueous solutions.
Biosensor preparation and modification
Prior to coating, the bare GCE was carefully polished with 0.05 μm alumina slurry, then rinsed thoroughly with double distillated water, followed by ultrasonication in ethanol and double distilled water for 5 min, respectively and dried an air before use. About 0.025 g of CS was dissolved in 5.0 mL of acetate buffer solution (pH 5.0) via magnetic stirring at room temperature for 4 h. Co3O4 nanoparticles/CS suspension (1 mg mL −1) was prepared by dispersing Co3O4 nanoparticles in CS solution with ultrasonication for 4 h. About 5 μL of GR solution (2 mg/mL) was dropped on the cleaned surface of GCE and dried in air at room temperature. About 5 μL of Co3O4/CS solution was dropped on the surface of GR modified GCE to prepare the Co3O4/CS/GR/GCE electrode. 10 μL of GLOx solution (0.025 Units/μL) was dropped on the surface of Co3O4/CS/GR/GCE and the resulting electrode was placed in 2.5% glutaraldehyte vapor for 15 min for the crosslinking of the enzymes. 7.5 μL of nafion solution (0.5%) was casted and allowed to dry on the enzyme electrode. Finally, the biosensor was immersed in 0.05 M PBS (pH 7.5) to wash out the unbound components from the electrode surface. The glutamate biosensor was stored at +4 °C in a refrigerator when not in use. The fabrication steps of the biosensor are presented in Scheme 1.
Results and discussion
Morphology of composite electrode
The surface morphologies of Co3O4/CS/GR/GCE and GLOx/Co3O4/CS/GR/GCE were investigated by SEM (). Image a shows that the Co3O4 nanoparticles are uniformly embedded in the porous CS network. The porous nature of the resulting film is suitable for the immobilization of enzymes. The surface of GLOx/Co3O4/CS/GR/GCE (image b) shows globular structures on Co3O4/CS/GR composite indicating that enzymes were successfully immobilized on the surface of Co3O4/CS/GR/GCE.
Electrochemical characteristics of the electrodes
CV of the ferricyanide system is a convenient and valuable tool to monitor the characteristics of the surface modified electrodes. The electron transfer properties of bare GCE, and Co3O4/CS/GR/GCE were investigated in 0.1 M KCl solution containing 5 mM Fe(CN)63−/4− at 50 mVs−1. As shown in well-defined redox peaks of Fe(CN)63−/4− were observed for bare GCE. The modification of the bare GCE with Co3O4/CS/GR resulted in an increase in peak currents and a decrease in peak separation which can be attributed to the high electric conductivity and large surface area of GR and Co3O4 nanoparticles (Dalkıran et al. Citation2014, Kuila et al. Citation2011, Pérez-López and Merkoçi Citation2012). shows cyclic voltammograms (CVs) of the Co3O4/CS/GR/GCE electrode in 5 mM [Fe(CN)6]3−/4− solution containing 0.1 M KCl over the potential range from −0.1 to 0.6 V at various scan rates. As shown in inset, the relationship between the peak current (Ip) and the square root of the scan rate (v1/2) is linear for the Co3O4/CS/GR/GCE. This suggests that the mass transfer phenomenon in the double layer region of the electrodes is mainly controlled by diffusion (Qiu et al. Citation2011). The average electroactive surface area could be calculated according to the Randles–Sevcik equation (Bard and Faulkner Citation2001):
where n is the number of electrons participating in the redox reaction, A is the area of the electroactive surface area (cm2), D is the diffusion coefficient of the molecule in solution (cm2 s−1), C corresponds to the bulk concentration of the redox probe (mol cm3), and v is the scan rate (V s − 1). The [Fe(CN)6]3−/4− redox couple used in this study exhibits a heterogeneous one-electron transfer (n = 1). C is equal to 5 mM, and D is (6.70 × 10−6 cm2 s−1) (Zeng et al. Citation2006). According to the equation, the average value of the electroactive surface area for Co3O4/CS/GR/GCE is 0.238 cm2. The effective electrochemical surface area of Co3O4/CS/GR/GCE (0.238 cm2) is 3.35 times higher than the surface area of bare GCE (0.071 cm2). This result revealed that the Co3O4/CS/GR composite is more suitable to facilitate electron transfer between redox probe and working electrode. This may result from the high electron transfer kinetics and the large surface area of Co3O4/CS/GR/GCE and synergistic effects of GR and Co3O4.
Figure 2. (A) Cyclic voltammograms of (a) GCE and (b) Co3O4/CS/GR/GCE at 50 mV s−1 (B) The Nyquist curves of (a) GCE and (b) Co3O4/CS/GR/GCE in 0.1 M KCl solution containing 5.0 mM Fe(CN)63−/4− (C) Peak currents of Co3O4/CS/GR/GCE as a function of scan rate for the determination of the effective working surface area. Inset: Cyclic voltammograms of the Co3O4/CS/GR/GCE at scan rates of 10, 20, 50, 75, 100, 200, and 300 mV s−1 in a 0.1 M KCl solution containing 5.0 mM Fe(CN)63−/4−.
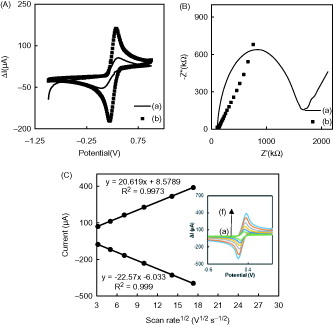
EIS, a powerful tool for studying the interface characteristics of the modified electrodes, was employed to probe the electron transfer kinetics at the bare GCE and Co3O4/CS/GR/GCE. shows the Nyquist plots of the EIS of (a) bare GCE and (b) Co3O4/CS/GR/GCE in 0.10 M KCl solution containing 5.0 mM [Fe(CN)6]3−/4−. In Nyquist plots the imaginary impedance (Z″) is plotted against the real impedance (Z′). The typical impedance spectrum includes a semicircle portion and a linear portion. The semicircle portion at higher frequencies corresponds to the electron-transfer limited process, and the linear portion at lower frequencies represents the diffusion-limited process. The diameter of the semicircle in the impedance spectrum is equal to the electron-transfer resistance, Rct, which controls the electron-transfer kinetics of the redox probe at the electrode interface (Li et al. Citation2014). It can be seen from the that a well-defined semicircle was obtained with the bare GCE (curve a). When the electrode was modified with Co3O4/CS/GR composite (curve b), smaller semicircle was obtained which indicated that the electron transfer resistance of [Fe(CN)6]3−/4− on Co3O4/CS/GR/GCE was greatly decreased as compared with that on the bare GCE. The impedance results were consistent with the conclusion from the CV experiments, indicating that the nanocomposite was successfully coated on electrode surface.
Optimization of experimental parameters
Zhang et al. (Citation2006) have reported the high affinity of CS to GLOx postulated by strong electrostatic interactions between the polycationic CS (pKa = 6.5) and polyanionic GLOx (pI = 6.2). To investigate the effect of the enzyme amount on the response current, the enzyme electrodes were prepared with three different GLOx amounts as 0.125, 0.25, and 0.375 Units (U). With the increase of the GLOx concentration from 0.125 U to 0.25 U, the response current of the glutamate biosensor in the presence of 0.2 mM glutamate increased accordingly, which implies that higher enzyme amount resulted in higher sensitivity (). The decrease in the response current of the biosensor at high enzyme amount may be attributed to the increase of the diffusional resistance for the substrate to arrive at the electrode surface. The optimal amount of 0.25 U of GLOx was found for the immobilization on the working electrode. This amount was consequently used for all biosensors in further experiments. About 0.23 U (Batra and Pundir Citation2013), 0.27 U (Chakraborty and Raj Citation2007) 0.1–0.2 U (Backer et al. Citation2013), 0.40 U (Cui et al. Citation2007), 0.50 U (Tseng et al. Citation2013), 0.58 U (Jamal et al. Citation2010) were reported as optimum enzyme amount for glutamate biosensors in the literature. It can be concluded that the result of optimum enzyme amounts study is consistent with the results reported in the literature.
Figure 3. The effects of (a) enzyme amount (+0.70 V; pH 7.5), (b) buffer pH (+0.70 V), (c) applied potential (pH 7.5), and (d) temperature (+0.70 V; pH 7.5) on the response of GLOx/Co3O4/CS/GR/GCE (0.05 M PBS containing 0.2 mM glutamate).
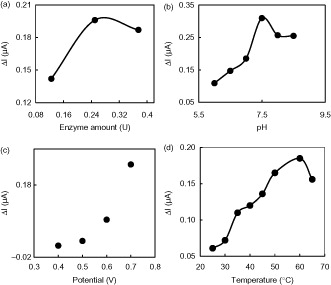
The influence of solution pH was investigated to obtain the optimum conditions for the determination of glutamate. This study was conducted in 0.05 M PBS containing 0.2 mM glutamate between pH 6.0 and 8.5. As can be seen from the response increases significantly from pH 6.0 to 7.5. The best amperometric response of the biosensor was obtained at pH 7.5. At pH values higher than 7.5, a decrease of the response was observed. Therefore, PBS with pH 7.5 was considered as the optimal electrolyte. This pH is in good agreement with the data reported in the literature (Batra and Pundir Citation2013). This optimum value is also compatible with the pH range (7.0–7.5) reported for the free GLOx (Chang et al. Citation2003, Kwong et al. Citation2000; Villarta et al. Citation1991). This study indicates that the immobilization procedure has no significant effect on the optimum pH of GLOx. Different pH values such as 6.5 (Cui et al. Citation2007), 7.0 (Basu et al. Citation2006, Chang and Chen Citation2007, Pauliukaite et al. Citation2006) 7.4 (Claussen et al. Citation2011, Jamal et al., Citation2010, Ryan et al. Citation1997, Tian, et al. Citation2009, Zhang et al. Citation2006), 8.0 (Backer et al. Citation2013) were also reported for glutamate enzyme electrodes.
The effect of applied potential on the response currents of GLOx/Co3O4/CS/GR/GCE in the presence of 0.2 mM glutamate was examined at different potentials between +0.40 and +0.70 V. With the increase of the applied potential from +0.40 V to +0.70 V, the response current increased regularly and a maximum response current was observed at +0.70 V (). Thus, a potential of 0.70 V was chosen for glutamate detection in the following experiments. GLOx catalyzes the oxidation reaction of l-glutamate to α-ketoglutarate according to EquationEq. (1)(1) (Pauliukaite et al. Citation2006). The electron transfer mechanism of the presented biosensor is based on the formation of H2O2 from glutamate by GLOx and its oxidation at an applied potential of +0.70 V according to EquationEq. (2)
(2) .
(1)
(2)
The influence of temperature on the electrochemical response of biosensor was investigated over the range of 25–65 °C in the presence of 0.2 mM glutamate. shows that the response current of the biosensor increased up to 60 °C. Further increase in temperature caused a decrease in the response current of the electrode, which is caused by the denaturation of the enzyme. The best current response obtained at 60 °C glutamate measurements were performed at body temperature (37 °C) because the response current was stable between 35 and 40 °C. Moreover, the repeatability of the calibration curves obtained over 40 °C was not satisfactory. Different temperatures such as 37 °C (Chang and Chen Citation2007), 35 °C (Basu et al. Citation2006, Batra and Pundir Citation2013, Rahman et al. Citation2005), 42 °C (Gholizadeh et al. Citation2012), 25 °C (Maalouf et al. Citation2007, Tang et al. Citation2007, Tian et al. Citation2009) were selected as optimum for glutamate biosensors.
Performance parameters of glutamate biosensor
The amperometric response of the presented biosensor was given in on successive injection of glutamate into the stirring 0.05 M PBS (pH 7.5) at an applied potential of +0.70 V. Immediately after the addition of glutamate, the anodic current increased and reached a steady state within 25 s. This response time is quite fast and highly suitable for biosensor response. There are longer; 2 min (Almeida and Mulchandani Citation1993), 120 s (Basu et al. Citation2006), 20–50 s (Pauliukaite et al. Citation2006), 20–40 s (Kulagina et al. Citation1999), and shorter response times; 10 s (Frey et al. Citation2010; Rahman et al. Citation2005, Ryan et al. Citation1997), 5 s (Jamal et al. Citation2010), 2 s (Batra and Pundir Citation2013) reported for glutamate biosensors.
Figure 4. (a) Calibration curve of the GLOx/Co3O4/CS/GR/GCE, (b) Typical current–time response of the on successive injection of glutamate into a stirred solution of 0.05 M PBS (pH 7.5) at applied potential +0.70 V, and (c) The effect of glutamate concentration upon the amperometric response of the biosensor (0.05 M pH 7.5 PBS, 37 °C).
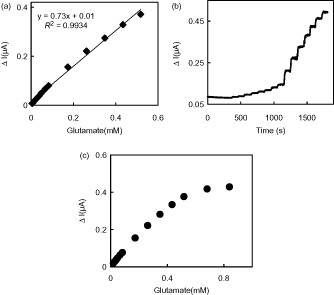
The response current of GLOx/Co3O4/CS/GR/GCE was linear in the range from 4.0 × 10−6 M to 6.0 × 10−4 M with a correlation coefficient of 0.9934. The sensitivity obtained from calibration curve was 0.73 μA/mM for glutamate. Also, the sensitivity calculated by considering the effective electrochemical surface area of GLOx/Co3O4/CS/GR/GCE (0.099 cm2) was found as 7.37 μA/mMcm2 for glutamate. The limit of detection (LOD) of the biosensor, which is the minimum concentration at which the ratio of signal to noise is not less than 3, is 2.0 × 10−6 M (S/N = 3). Compared with some previously reported glutamate biosensors this detection limit is lower than that of poly(carbamoyl) sulfonate hydrogel modified bienzymatic Clark electrode (5.0 × 10−6 M) (Cui et al. Citation2007) and electrodeposited CS modified microelectrodes (2.5 ± 1.2 × 10−6 M) (Tseng et al. Citation2013) but comparable to carboxylated multiwalled carbon nanotubes (c-MWCNT), gold nanoparticles (AuNPs) and CS modified Au electrode (1.6 × 10−6 M) (Batra and Pundir Citation2013). The apparent Michaelis–Menten constant (Kmapp) was calculated to evaluate the catalytic activity of immobilized enzyme by using the Lineweaver–Burk equation:
where Iss is the steady current after the addition of substrate, C is the bulk concentration of the substrate, and Imax is the maximum current measured under the saturated substrate condition (Xu et al. Citation2003). The apparent Michaelis–Menten constant, in this study was calculated to be 1.09 mM for the GLOx/Co3O4/CS/GR/GCE. This value was smaller than those of a GLOx immobilized polycarbonate membrane based biosensor (1.92 mM) (Basu et al. Citation2006), and nafion and methyl viologen modified GCE based biosensor (2.84 mM) (Maalouf et al. Citation2007). These results show that the current biosensor possesses higher affinity to glutamate compared with some of the earlier biosensors.
The repeatability and long-term stability of our proposed biosensor have also been studied. During the repeatability study, five calibration curves were plotted by the use of the same enzyme electrode sequentially (). The relative standard deviation (RSD) of the sensitivities was calculated to be 1.0% which confirms the reliable repeatability of GLOx/Co3O4/CS/GR/GCE.
The long-term stability of the GLOx/Co3O4/CS/GR/GCE was determined by measuring response current of 0.2 mM glutamate during three weeks. The electrode was stored in dry atmosphere at +4 °C when not in use. There were not obvious changes of amperometric response during the first five days and then an activity loss of 45% was observed after three weeks. The satisfactory stability of this biosensor may be attributed to the chemical and mechanical stability of Co3O4/CS/GR nanocomposite and thus it can be used as a useful platform for immobilizing enzymes to enhance biosensor performances. The effect of possible interfering substances on GLOx/Co3O4/CS/GR/GCE was investigated at +0.70 V in 0.05 M PBS (pH 7.5) by injection of 0.1 mM glutamate and 0.01 mM interfering species. The interference caused by lysine, glucose, methionine, aspartic acid, valine, glutamine, serine, leucine, isoleucine, phenylalaline, arginine to 0.1 mM glutamate was <5%. This result indicated that the presented biosensor has acceptable anti-interferent ability. The performance parameters and the optimum working conditions of the presented biosensor are summarized in .
Table 1. The performance parameters and the optimum working conditions of the biosensor.
A comparison of the performance of different glutamate biosensors was summarized in . The results presented in show that the different characteristics of the proposed glutamate biosensor are better in some cases or comparable with the other glutamate biosensors reported so far.
Table 2. Characteristics of various amperometric glutamate biosensors.
Conclusion
In this paper, a novel amperometric biosensor based on GR/Co3O4/CS nanocomposite for monitoring glutamate was described. The use of a GR and Co3O4 nanoparticles resulted in the improved analytical performance of the glutamate biosensor in terms of low response time (25 s), wide linear range (4.0 × 10−6 M − 6.0 × 10−4 M), good repeatability (1.0%) and no interference by common interfering substances. Moreover, the cost of the presented biosensor is lower than that of the conventional techniques and the preparation procedure of the biosensor is very simple. The purposed strategy can be extended for the development of other enzyme-based biosensors. It can be concluded that the presented biosensor can be used for glutamate determination in food samples.
Funding information
We gratefully acknowledge the financial support of Ankara University Research Fund (Project No: 13L4240002) and a scholarship for B. DALKIRAN of The Scientific and Technological Research Council of Turkey.
Disclosure statement
The authors report no conflicts of interest. The authors alone are responsible for the content and writing of this article.
References
- Abbasi E, Akbarzadeh A, Kouhi M, Milani M. 2016. Graphene: synthesis, bio-applications, and properties. Artif Cells Nanomed Biotechnol. 44:150–156.
- Acebal CC, Lista AG, Band BSF. 2008. Simultaneous determination of flavor enhancers in stock cube samples by using spectrophotometric data and multivariate calibration. Food Chem. 106:811–815.
- Acuna AAM, Trias JF. 2009. A high performance liquid chromatography method with electrochemical detection of gamma-aminobutyric acid, glutamate and glutamine in rat brain homogenates. J Neurosci Methods. 183:176–181.
- Ali A, Israr-Qadir M, Wazir Z, Tufail M, Ibupoto ZH, Jamil-Rana S, Willander M. 2015. Cobalt oxide magnetic nanoparticles–chitosan nanocomposite based electrochemical urea biosensor. Ind J Phys. 89:331–336.
- Almeida NF, Mulchandani AK. 1993. A mediated amperometric enzyme electrode using tetrathiafulvalene and l-glutamate oxidase for the determination of l-glutamic acid. Anal Chim Acta. 282:353–361.
- Backer M, Rakowski D, Poghossian A, Biselli M, Wagner P, Schöning MJ. 2013. Chip-based amperometric enzyme sensor system for monitoring of bioprocesses by flow-injection analysis. J Biotechnol. 163:371–376.
- Bard AJ, Faulkner LR. 2001. Electrochemical Methods: Fundamentals and Applications, 2nd ed. New York: John Wiley & Sons Inc.
- Basu AK, Chattopadhyay P, Roychudhuri U, Chakraborty R. 2006. A biosensor based on co-immobilized l-glutamate oxidase and l-glutamate dehydrogenase for analysis of monosodium glutamate in food. Biosens Bioelectron. 21:1968–1972.
- Batra B, Pundir CS. 2013. An amperometric glutamate biosensor based on immobilization of glutamate oxidase on to carboxylated multiwalled carbon nanotubes/gold nanoparticles/chitosan composite film modified Au electrode. Biosens Bioelectron. 47:496–501.
- Beyene NW, Moderegger H, Kalcher K. 2003. A stable glutamate biosensor based on mno2 bulk-modified screen-printed carbon electrode and nafion® film-immobilized glutamate oxidase. S Afr J. Chem. 56:54–59.
- Buck K, Voehringer P, Ferger B. 2009. Rapid analysis of GABA and glutamate in microdialysis samples using high performance liquid chromatography and tandem mass spectrometry. J Neurosci Methods. 182:78–84.
- Casero E, Alonso C, Petit-Domínguez MD, Vázquez L, Parra-Alfambra AM, Merino P, Lorenzo E. 2014. Lactate biosensor based on a bionanocomposite composed of titanium oxide nanoparticles, photocatalytically reduced graphene, and lactate oxidase. Microchim Acta. 181:79–87.
- Chakraborty S, Raj CR. 2007. Amperometric biosensing of glutamate using carbon nanotube based electrode. Electrochem Commun. 9:1323–1330.
- Chang KS, Chen CY. 2007. Effect of l-aspartate concentration on the response of the amperometric l-glutamate.sensor for the measurement of l-glutamate and aspartate aminotransferase activity in serum. Anal Lett. 40:933–945.
- Chang KS, Hsu WL, Chen HY, Chang CK, Chen CY. 2003. Determination of glutamate pyruvate transaminase activity in clinical specimens using a biosensor composed of immobilized l-glutamate oxidase in a photo-crosslinkable polymer membrane on a palladium-deposited screen-printed carbon electrode. Anal Chim Acta. 481:199–208.
- Claussen JC, Artiles MS, McLamore ES, Mohanty S, Shi J, Rickus JL, Fisher TS, Porterfield MD. 2011. Electrochemical glutamate biosensing with nanocube and nanosphere augmented single-walled carbon nanotube networks: a comparative study. J Mater Chem. 21:11224–11231.
- Cui Y, Barford JP, Renneberg R. 2007. Development of an l-glutamate biosensor using the coimmobilization of l-glutamate dehydrogenase and p-hydroxybenzoate hydroxylase on a Clark-type electrode. Sens Actuat B. 127:358–361.
- Dalkıran B, Kaçar C, Erden PE, Kılıc E. 2014. Amperometric xanthine biosensors based on chitosan–Co3O4–multiwall carbon nanotube modified glassy carbon electrode. Sens Actuat B. 200:83–91.
- Demirhan BE, Demirhan B, Sönmez C, Torul H, Tamer U, Yentür G. 2015. Monosodium glutamate in chicken and beef stock cubes using high-performance liquid chromatography. Food Addit Contam Part B. 8:63–66.
- Devi R, Yadav S, Nehra R, Yadav S, Pundir CS. 2013. Electrochemical biosensor based on gold coated iron nanoparticles/chitosan composite bound xanthine oxidase for detection of xanthine in fish meat. J Food Eng. 115:207–214.
- Frey O, Holtzmanb T, McNamarab RM, Theobaldb DEH, van der Wala PD, de Rooija NF, et al. 2010. Enzyme-based choline and l-glutamate biosensor electrodes on silicon microprobe arrays. Biosens Bioelectron. 26:477–484.
- Gholizadeh A, Shahrokhiana S, Zada AI, Mohajerzadeh S, Vosoughia M, Darbari S, Sanaee Z. 2012. Mediator-less highly sensitive voltammetric detection of glutamate using glutamate dehydrogenase/vertically aligned CNTs grown on silicon substrate. Biosens Bioelectron. 31:110–115.
- Gündüz T, Gündüz N, Kiliç E, Köseoğlu F, Oztas GS. 1988. Titrations in non aqueous media Part X. Potentiometric and conductimetric titrations of amino acids with tetrabutylammonium hydroxide in pyridine and acetonitrile solvents. Analyst. 113:715–719.
- Jamal M, Xu J, Razeeb KM. 2010. Disposable biosensor based on immobilisation of glutamate oxidase on Pt nanoparticles modified Au nanowire array electrode. Biosens Bioelectron. 26:1420–1424.
- Kaçar C, Dalkiran B, Erden PE, Kiliç E. 2014. An amperometric hydrogen peroxide biosensor based on Co3O4 nanoparticles and multiwalled carbon nanotube modified glassy carbon electrode. Appl Surf Sci. 311:139–146.
- Kuila T, Bose S, Khanra P, Mishra AK, Kim NH, Lee JH. 2011. Recent advances in graphene-based biosensors. Biosens Bioelectron. 26:4637–4648.
- Kulagina NV, Shankar L, Michael AC. 1999. Monitoring glutamate and ascorbate in the extracellular space of brain tissue with electrochemical microsensors. Anal Chem. 71:5093–5100.
- Kwong AWK, Gründig B, Hu J, Renneberg R. 2000. Comparative study of hydrogel-immobilized l-glutamate oxidases for a novel thick-film biosensor and its application in food samples. Biotechnol Lett. 22:267–272.
- Li X, Liu X, Wang W, Li L, Lu X. 2014. High loading Pt nanoparticles on functionalization of carbon nanotubes for fabricating nonenzyme hydrogen peroxide sensor. Biosens Bioelectron. 59:221–226.
- Lim CX, Hoh HY, Ang PK, Loh KP. 2010. Direct voltammetric detection of DNA and pH sensing on epitaxial graphene: an insight into the role of oxygenated defects. Anal Chem. 82:7387–7393.
- Liu Y, Yu D, Zeng C, Miao Z, Dai L. 2010. Biocompatible graphene oxide-based glucose biosensors. Langmuir. 26:6158–6160.
- Maalouf R, Chebib H, Saikali Y, Vittori O, Sigaud M, Jaffrezic-Renault N. 2007. Amperometric and impedimetric characterization of a glutamate biosensor based on nafion and a methyl viologen modified glassy carbon electrode. Biosens Bioelectron. 22:2682–2688.
- Pauliukaite R, Zhylyak G, Citterio D, Spichiger-Keller UE. 2006. l-Glutamate biosensor for estimation of the taste of tomato specimens. Anal Bioanal Chem. 386:220–227.
- Pérez-López B, Merkoçi A. 2012. Carbon nanotubes and graphene in analytical sciences. Microchim Acta. 179:1–16.
- Pumera M, Ambrosi A, Bonanni A, Chng ELK, Poh HL. 2010. Graphene for electrochemical sensing and biosensing. Trends Anal Chem. 29:954–965.
- Qiu JD, Huang J, Liang RP. 2011. Nanocomposite film based on graphene oxide for high performance flexible glucose biosensor. Sens Actuat B: Chem. 160:287–294.
- Rahman MA, Kwon NH, Won MS, Choe ES, Shim YB. 2005. Functionalized conducting polymer as an enzyme-immobilizing substrate: an amperometric glutamate microbiosensor for in vivo measurements. Anal Chem. 77:4854–4860.
- Ryan MR, Lowry JP, O'Neill RD. 1997. Biosensor for neurotransmitter l-glutamic acid designed for efficient use of l-glutamate oxidase and effective rejection of interference. Analyst. 122:1419–1424.
- Şimşek Ş, Aynacı E, Arslan F. 2016. An amperometric biosensor for l-glutamate determination prepared from l-glutamate oxidase immobilized in polypyrrole–polyvinylsulphonate film. Artif Cells Nanomed Biotechnol. 44:356–361.
- Sun CL, Lee HH, Yang JM, Wu CC. 2011. The simultaneous electrochemical detection of ascorbic acid, dopamine, and uric acid using graphene/size-selected Pt nanocomposites. Biosens Bioelectron. 26:3450–3455.
- Tang L, Zhu Y, Xu L, Yang X, Li C. 2007. Amperometric glutamate biosensor based on self-assembling glutamate dehydrogenase and dendrimer-encapsulated platinum nanoparticles onto carbon nanotubes. Talanta. 73:438–443.
- Tian F, Gourine AV, Huckstepp RTR, Dale N. 2009. A microelectrode biosensor for real time monitoring of l-glutamate release. Anal Chim Acta. 645:86–91.
- Tseng T, Yao J, Chan WC. 2013. Selective enzyme immobilization on arrayed microelectrodes for the application of sensing neurotransmitters. Biochem Eng J. 78:146–153.
- Tsukatani T, Matsumoto K. 2005. Sequential fluorometric quantification of γ-aminobutyrate and l-glutamate using a single line flow-injection system with immobilized-enzyme reactors. Anal Chim Acta. 546:154–160.
- Tucci S, Pinto C, Goyo J, Rada P, Hernández L. 1998. Measurement of glutamine and glutamate by capillary electrophoresis and laser induced fluorescence detection in cerebrospinal fluid of meningitis sick children. Clin Biochem. 31:143–150.
- Varma S, Yigzaw Y, Gorton L. 2006. Prussian blue-glutamate oxidase modified glassy carbon electrode: a sensitive l-glutamate and β-N-oxalyl-α,β-diaminopropionic acid (β-ODAP) sensor. Anal Chim Acta. 556:319–325.
- Villarta RL, Cunningham DD, Guilbault GG. 1991. Amperometric enzyme electrodes for the determination of l-glutamate. Talanta. 38:49–55.
- Wang M, Zhang D, Tong Z, Xu X, Yang X. 2011. Voltammetric behavior and the determination of quercetin at a flowerlike Co3O4 nanoparticles modified glassy carbon electrode. J Appl Electrochem. 41:189–196.
- Xu JZ, Zhu JJ, Wu Q, Hu Z, Chen HY. 2003. An amperometric biosensor based on the coimmobilization of horseradish peroxidase and methylene blue on a carbon nanotubes modified electrode. Electroanalysis. 15:219–224.
- Yang L, Wang G, Liu Y, Wang M. 2013. Development of a biosensor based on immobilization of acetylcholinesterase on NiO nanoparticles– carboxylic graphene–nafion modified electrode for detection of pesticides. Talanta. 113:135–141.
- Yılmaz D, Karakuş E. 2011. Construction of a potentiometric glutamate biosensor for determination of glutamate in some real samples. Artif Cells Blood Substit Biotechnol. 39:385–391.
- Yu Y, Sun Q, Zhou T, Zhu M, Jin L, Shi G. 2011. On-line microdialysis system with poly(amidoamine)-encapsulated Pt nanoparticles biosensor for glutamate sensing in vivo. Bioelectrochemistry. 81:53–57.
- Zeng J, Wei W, Wu L, Liu X, Liu K, Li Y. 2006. Fabrication of poly(toluidine blue O)/carbon nanotube composite nanowires and its stable low-potential detection of NADH. J Electroanal Chem. 595:152–160.
- Zeng Q, Cheng JS, Liu XF, Bai HT, Jiang JH. 2011. Palladium nanoparticle/chitosan-grafted graphene nanocomposites for construction of a glucose biosensor. Biosens Bioelectron. 26:3456–3463.
- Zhang M, Mullens C, Gorski W. 2006. Amperometric glutamate biosensor based on chitosan enzyme film. Electrochim Acta. 51:14528–14532.
- Zhou K, Zhua Y, Yang X, Luo J, Li C, Luan S. 2010. A novel hydrogen peroxide biosensor based on Au–graphene–HRP–chitosan biocomposites. Electrochim Acta. 55:3055–3060.
- Zhou M, Zhai Y, Dong S. 2009. Electrochemical sensing and biosensing platform based on chemically reduced graphene oxide. Anal Chem. 81:5603–5613.