Abstract
Alzheimer's disease is a very common progressive neurodegenerative disorder affecting the learning and memory centers in the brain. The hallmarks of disease are the accumulation of β-amyloid neuritic plaques and neurofibrillary tangles formed by abnormally phosphorylated tau protein. Alzheimer's disease is currently incurable and there is an intense interest in the development of new potential therapies. Chromatin modifying compounds such as sirtuin modulators and histone deacetylase inhibitors have been evaluated in models of Alzheimer's disease with some promising results. For example, the natural antioxidant and sirtuin 1 activator resveratrol has been shown to have beneficial effects in animal models of disease. Similarly, numerous histone deacetylase inhibitors including Trichostatin A, suberoylanilide hydroxamic acid, valproic acid and phenylbutyrate reduction have shown promising results in models of Alzheimer's disease. These beneficial effects include a reduction of β-amyloid production and stabilization of tau protein. In this review we provide an overview of the histone deacetylase enzymes, with a focus on enzymes that have been identified to have an important role in the pathobiology of Alzheimer's disease. Further, we discuss the potential for pharmacological intervention with chromatin modifying compounds that modulate histone deacetylase enzymes.
Histone deacetylase (HDAC) inhibitors represent a new class of anticancer compounds. To date, suberoylanilide hydroxamic acid (SAHA, Vorinostat, Zolinza™) and depsipeptide (Romidespin, Istodax™) have been approved by the US Food and Drug administration (FDA) for the treatment of cutaneous T-cell lymphoma Citation1 Citation2 Citation3 Citation4 . Numerous clinical trials involving HDAC inhibitors, either as monotherapies or in combination with other anticancer modalities, are currently underway for both hematological and solid malignancies Citation5 Citation6 Citation7 . The anticancer properties of HDAC inhibitors are relatively well known Citation5 Citation6 Citation7 . Although not as thoroughly investigated, it is emerging that HDAC inhibitors may have clinical potential for non-oncological applications, including asthma, cardiac hypertrophy and neurodegenerative conditions Citation8 Citation9 Citation10 Citation11 Citation12 Citation13 Citation14 Citation15 Citation16 .
The aim of this review is to focus on the therapeutic potential of HDAC inhibitors in Alzheimer's disease (AD). Alzheimer's disease is the most common form of dementia and given the aging population in Western societies, prevalence is expected to continue to grow Citation17. It is an age-related progressive neurodegenerative disorder affecting the cortex and hippocampus (learning and memory centers in the brain), and is considered to be a disease of synaptic dysfunction and loss Citation18 Citation19 Citation20 Citation21 . The hallmark features of AD are: 1) the accumulation of β-amyloid neuritic plaques resulting from aberrant cleavage of the amyloid precursor protein (APP); typically APP is cleaved by α-secretase however, it can be cleaved into a soluble and the highly insoluble β-amyloid fragments, by β- and γ-secretases and 2) neurofibrillary tangles formed by abnormally phosphorylated tau protein Citation17. Other characteristics of AD include direct neurotoxic effects by lipid peroxidation, protein oxidation and formation of radical species (oxygen and nitrogen), inflammation arising from microglia surrounding plaques, mitochondrial damage and altered mitochondrial distribution, abnormal calcium regulation and aberrant interaction between metals and β-amyloid Citation22 Citation23 Citation24 . Although, cholinesterase inhibitors and an N-methyl-D-aspartate antagonist are currently approved by the FDA to assist in managing symptoms, the disease is currently untreatable Citation25 Citation26 Citation27 . Therefore, there is intense interest in the investigation of novel compounds as potential therapeutics for AD. Chromatin-modifying compounds such as HDAC inhibitors have been shown to have beneficial effects in experimental models of AD. In this review, we provide an overview of histone deacetylase enzymes and their relevance to AD. Pharmacological modulation of these enzymes in the context of AD is discussed.
Sirtuins in Alzheimer's disease
Histone acetylation is regulated by the opposing actions of HDAC enzymes and histone acetyltransferases (HATs) Citation28 Citation29 Citation30 . Briefly, HATs catalyze the addition of the acetyl group of acetyl-CoA to the ɛ-amino lysine residue of histone lysines resulting in an open, transcriptionally permissive, chromatin architecture Citation31 Citation32. The HDAC enzymes catalyze the opposite (removal of acetyl groups) resulting in a more condensed, transcriptionally repressive chromatin conformation Citation28. In addition, numerous non-histone protein substrates, with key cellular functions (e.g. chaperones, DNA repair proteins, cell motility proteins, transcription factors and co-regulators and signaling mediators) have been identified for HDAC enzymes Citation33 Citation34 Citation35 Citation36 . HDAC enzymes are categorized into two main families; the metal-dependent HDAC1–11 enzymes and the seven mammalian class III sirtuins.
The class III HDAC enzymes consist of the sirtuins (SIRTs) 1–7 which are homologous to the Saccharomyces cerevisiae silent information regulator 2 (Sir2) () Citation37 Citation38. The sirtuins are nicotinamide adenine dinucleotide (NAD+)-dependent enzymes. They deacetylate substrates via the consumption of NAD+ releasing nicotinamide, O-acetyl-ADP-ribose and the deacetylated substrate Citation29. The sirtuins contain a 257 amino acid catalytic core domain and have differing N- and C-terminal tails and zinc-binding domains Citation37. Phylogenetically the sirtuins can be further sub-classified into four distinct classes Citation37 Citation38. Class I consists of SIRTs 1–3 and those found in yeast. SIRT 4 is the sole member of class II enzymes with homology to enzymes found in bacteria, insects, nematodes and protozoans Citation37. Class III consists of SIRT 5, with homology to prokaryotic enzymes. Class IV includes SIRTs 6 and 7 which have homologous enzymes distributed in plants, vertebrates and metazoans Citation37 Citation38. The sirtuins have differing subcellular localizations with SIRTs 3, 4 and 5 found in the mitochondria, SIRT 2 is primarily cytoplasmic and SIRTs 1, 6 and 7 are found predominantly in the nucleus Citation39 Citation40. SIRT 1 is mainly associated with euchromatin but also shares a degree of cytoplasmic localization Citation39 Citation40. SIRT 6 is associated predominantly with heterochromatin and SIRT 7 is localized in the nucleolus Citation39 Citation40. SIRTs 1, 3 and 5 are NAD+ -dependent deacetylases. They catalyze the deacetylation of histone and non-histone substrates. SIRT 6 is an NAD+-dependent ADP ribosyltransferase (ART) and catalyzes the ribosylation of mitochondrial proteins. SIRTs 2 and 4 are both NAD+-dependent and ART enzymes. The properties of SIRT 7 are not well-defined Citation39.
Fig. 1. Schematic representation of the class III sirtuin (SIRT) deacetylases. The sirtuins are highly conserved nicotinamide adenine dinucleotide (NAD + ) dependent protein deacetylases (DAC) or ADP-ribosyltransferases (ART) which can be subdivided into four classes based on their phylogenetic lineage. The subcellular localization, DAC or ART binding domains (dark blue) and zinc binding domains (black) are depicted.
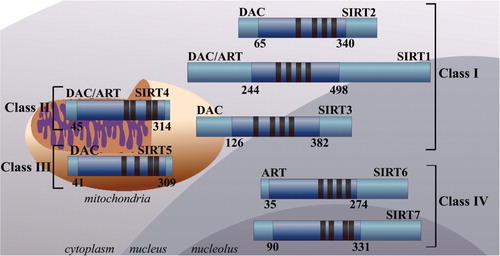
To date, SIRT 1 has been the most extensively investigated of the sirtuin enzymes. It has been shown to modulate metabolism (e.g. via modulation of peroxisome proliferator-activated receptor gamma coactivator-1α [PGC-1α]), cellular stress resistance (e.g. by interaction with forkhead box class O (FOXO) transcription factors) and genomic integrity (e.g. by interaction with p53 and Ku70), which have been the subject of recent reviews Citation41 Citation42 Citation43 . The functions of SIRT 1 in AD are summarized in . SIRT 1 has been shown to increase production of α-secretase, via deacetylation and activation of the retinoic acid receptor-β protein, which stimulates transcription of the ADAM10 gene Citation44 Citation45. This results in the increases in ADAM10 drive alpha-secretase cleavage of APP within the amyloid peptide region, resulting in the reduction of the β-amyloid peptide which gives rise to the characteristic amyloid plaques found in AD Citation44 Citation45 Citation46 . ADAM10 also cleaves the cell-surface Notch receptor initiating the Notch signaling pathway which results in the upregulation of genes involved in neurogenesis Citation47.
Fig. 2. Identified roles of sirtuin (SIRT) 1 in Alzheimer's disease. Although there a still controversies surrounding its precise mechanism of action, activation of SIRT 1 by the natural antioxidant resveratrol, may lead to the molecular effects depicted.
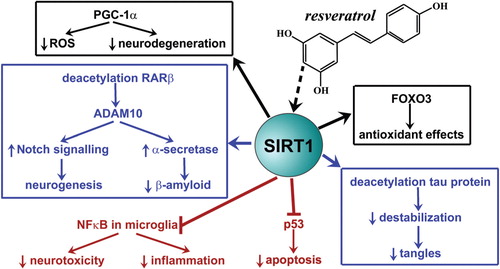
Further, SIRT 1 has been shown to deacetylate the tau protein resulting in destabilization and proteolysis Citation48. This reduces neurofibrillary tangles in neurons Citation48. Another effect of SIRT 1 in AD is mediated by inhibition of NFκB signaling in microglia resulting in the decrease of β-amyloid-induced release of neurotoxic chemokines, cytokines and nitric oxide Citation47 Citation49. Anti-apoptotic effects are mediated by interaction with p53 and antioxidant effects of SIRT 1 are mediated by activation of FOXO3 and regulation of PGC-1α Citation42 Citation47. Resveratrol, a natural polyphenol abundant in the skins of red grapes and putative SIRT 1 activator, has been shown to have efficacy in relevant models of AD () Citation50 Citation51 Citation52 Citation53 Citation54 Citation55 Citation56 . Previous studies have found protective effects of resveratrol on beta-amyloid-induced toxicity in cultured rat hippocampal cells Citation57 Citation58 Citation59 . Supplemental forms of resveratrol are undergoing evaluation in clinical trials for the disease. However, there are still question marks over its precise mechanism of action and whether the effects are mediated through activation of SIRT 1. Further, bioavailability of both oral resveratrol and its active metabolites remains controversial and it will be important to determine whether these reach biologically relevant concentrations to affect either sirtuin-dependent and/or independent pathways. Paradoxically, nicotinamide, a competitive sirtuin inhibitor has also shown beneficial effects in an animal model of AD, attenuating cognitive deficit Citation60. The mechanism was ascribed, at least in part, to reduced phosphorylation of tau protein at threonine-231 (T231) Citation60. Hyperacetylation of α-tubulin was also observed Citation60. These findings highlight the need for further clarification of the function of sirtuins in AD.
Metal-dependent histone deacetylases
The remaining 11 HDACs are typically referred to as the classical metal-dependent enzymes which require coordination of a divalent metal ion (zinc) for their catalytic activity () Citation6 Citation7 Citation61 Citation62. These HDAC enzymes are divided into class I (HDAC1, 2, 3 and 8), class IIa (4, 5, 7 and 9), class IIb (HDAC6 and 10) and class IV (HDAC11) on the basis of their homology to yeast proteins Citation6 Citation7 Citation61 Citation62. Class I enzymes share homology with Saccharomyces cerevisiae transcriptional regulator RDP3 whereas class II enzymes are homologous with yeast Hda1 Citation62. HDAC11 shares homology with both class I and II enzymes and is the sole member of class IV Citation62. Class 1 enzymes are expressed ubiquitously, primarily localized in the nucleus and have important roles in cellular proliferation and survival Citation63. Class IIa enzymes shuttle between the nucleus and cytoplasm and have more restricted tissue distributions and functions Citation6 Citation7 Citation35 Citation36 Citation61 Citation62 Citation64 Citation65. Little is known about the class IIb HDAC10. However, HDAC6, another class IIb member, is a major cytoplasmic protein with numerous identified non-histone substrates and important roles in aggresome formation and growth signaling Citation66 Citation67 Citation68 .
Fig. 3. Schematic representation of metal–dependent histone deacetylase (HDAC) enzymes. The classical HDACs are categorized into class 1 (HDAC1, 2, 3 and 8), class IIa (HDAC4, 5, 7 and 9), class IIb (HDAC6 and 10) and class IV (HDAC11) on the basis of their homology to yeast proteins. The deacetylase catalytic domain (pink), nuclear localization signal (purple), myocyte enhancer factor 2 binding domain (light blue), serine binding motif (orange). SE14 = serine–glutamate tetradecapeptide, ZnF = zinc finger protein binding domain and leucine rich domain are depicted. Subcellular localization is shown.
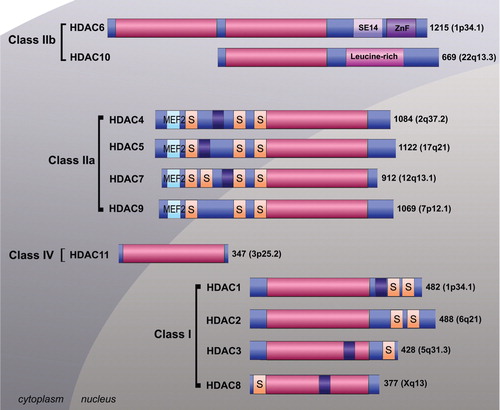
With respect to AD, the class I HDAC2 and class IIb HDAC6 enzymes, have been associated with the pathobiology of the disease. Firstly, a seminal study has indicated that over-expressing HDAC2 in neurons in mice results in decreased synaptic plasticity and memory formation that modifying HDAC2, indicating that modification of HDAC2 could be a beneficial treatment for the memory impairment that occurs in AD Citation69. In the same study it was shown that HDAC2 deficiency exhibits the opposite effects indicating that the enzyme has an important role in negatively modulating synaptic plasticity, learning and memory Citation69. A solid body of evidence has accumulated for the role of HDAC6 in various neurodegenerative conditions including AD Citation70. Firstly, HDAC6 has been shown to be over-expressed in the brains of AD patients (by 52% in cortices and 91% in hippocampi) Citation71. In the same study it was shown that HDAC6 binds with tau protein both in vitro and in human brain tissues Citation71. Tau was identified as a HDAC6 deacetylase inhibitor Citation72. A further study, using the HDAC6 selective inhibitor, tubacin, has indicated that inhibition of the enzyme results in attenuation of tau phosphorylation at T231, which is important for the regulation of the stability of the cytoskeleton; this may decrease neurofibrillary tangle formation in AD Citation71 Citation73. Additionally, abnormal mitochondrial transport is a feature of AD, and it has been identified that HDAC6 has an important function in the modulation of mitochondrial transport through an association with glycogen synthase kinase-3β GSK3β Citation17 Citation74.
Histone deacetylase inhibitors in Alzheimer's disease
A structurally disparate group of compounds have been identified to possess HDAC inhibition activity. The prototypical Trichostatin A and the clinically approved SAHA are part of the hydroxamic acid class of HDAC inhibitors with HDAC inhibition activity in the nanomolar to low micromolar range Citation2 Citation6 Citation7 Citation33 Citation61 Citation75. The cyclic peptides, which include trapoxin and clinically approved depsipeptide, are also potent HDAC inhibitors. Similarly, the benzamides such as entinostat and electrophilic ketones such α-ketomide are potent HDAC inhibitors Citation2 Citation6 Citation7 Citation33 Citation61 Citation75. Aliphatic acids which include valproic acid, butyrate and phenylbutyrate are the least potent group of HDAC inhibitors possessing inhibition activity in the millimolar range Citation75 Citation76 Citation77 Citation78 . These compounds are typically referred to as broad-spectrum (pan-) HDAC inhibitors. Although they inhibit multiple HDAC1–11 enzymes they do possess some selectivity for HDAC isoforms. It is becoming apparent that selectivity or isoform-specificity is important particularly when considering non-oncological applications. Therefore, there is an intense effort aimed at development of such compounds, the HDAC6 specific, tubacin and the HDAC8 selective PC-34051 being pertinent examples Citation79 Citation80 Citation81 Citation82 .
There is accumulating evidence indicating the potential benefits of classical metal-dependent HDAC inhibitors in models of AD. For example, Trichostatin A has been shown to increase diminished H4 acetylation and improve contextual performance in a mouse model of AD Citation83. Similarly, the clinical hydroxamic acid, SAHA, has been shown to rescue contextual memory in a transgenic mouse model of AD Citation84. However, most studies to date have focused on the aliphatic acid group of HDAC inhibitors. Although histone acetylation was not considered, valproic acid has been shown to inhibit the production of β-amyloid in cells (HEK293) transfected with the Swedish APP isoform (APP751) Citation85. Further, using the PDAPP (APP (V717F)) transgenic model of AD, valproic acid was shown to inhibit the production of β-amyloid in the brains of mice at biologically relevant doses of 400 mg/kg Citation85. Similarly, valproic acid has been shown to decrease β-amyloid production and to attenuate behavioral deficits in APP23 transgenic mice Citation86. Inhibition of GSK3β was suggested as a mechanism of action of valproic acid in AD Citation85. In another study, the beneficial effects of valproic acid in models of AD have been linked with histone acetylation (H4) Citation84. Valproic acid is particularly interesting given that it is relatively well-tolerated and has a very long history of clinical use as an anti-epileptic Citation87 Citation88 Citation89 . However, the findings from a recent clinical trial indicate potential contraindications with the use of valproic acid in Alzheimer's disease highlighting the need for further research with this commonly used compound Citation90.
The aliphatic acid HDAC inhibitor, phenylbutyrate, has also been investigated in models of AD. Several groups have shown beneficial effects upon AD pathology and memory performance with no signs of toxicity in AD transgenic mouse models Citation84 Citation91 Citation92 Citation93 Citation94 . Further, phenylbutyrate specifically represses apoptosis in stressed neuronal systems Citation95 Citation96 Citation97 . Findings have indicated that the beneficial effects of phenylbutyrate (increased synaptic plasticity, improved learning and memory and attenuation of spatial memory deficits) may be attributed to restored acetylation of histone H4 and to the clearance of intraneuronal Aβ accumulation Citation91 Citation92.
Although acetylation of histone H4 appears to be important in AD, the potential use of classical HDAC inhibitors in neurodegeneration remains controversial. Broad-spectrum HDAC inhibitors have cell-specific effects and are well-known for their potential to induce cell-death, apoptosis and cell-cycle arrest in malignant and transformed cells Citation7 Citation75 Citation98 Citation99. However, similar effects have been observed in neuronal cells Citation100. In this context, evaluation of more selective or isoform-specific compounds is important. In particular, HDAC2 and HDAC6 have been shown to have important roles in the pathobiology of AD Citation69 Citation70. Although there is no specific inhibitor of HDAC2 available, tubacin is highly selective for HDAC6 Citation79. As described earlier, tubacin has been shown to interact with tau protein Citation71.
Conclusions
Overall, the class III sirtuin deacetylases, in particular SIRT 1, have been shown to be important potential targets in AD. Numerous clinical trials, using resveratrol to target SIRT 1 are ongoing. The findings from clinical studies and further characterization of the sirtuins in relevant model systems are anticipated to improve our understanding of the therapeutic promise of targeting this class of enzymes in AD. Similarly, classical metal-dependent HDAC inhibitors have been shown to have beneficial effects of models of AD. While most studies have used relatively broad-spectrum inhibitors, it is becoming apparent that more selective or isoform-specific compounds may be more applicable. Evaluation of HDAC expression in animal models of disease akin to the atlas of the HDAC1–11 expression produced in normal rat brain will assist identifying relevant targets Citation101. Further genetic studies and experiments with more selective compounds (e.g. tubacin for HDAC6 and a selective HDAC8 inhibitor is available) are also required to clarify the roles of HDAC1–11 in the pathobiology of AD.
Conflict of interest and funding
TCK was the recipient of AINSE awards. Epigenomic Medicine is supported in part by the Victorian Government's Operational Infrastructure Support program. The authors (TCK and KV) declare that they have no direct financial relation with the commercial identities mentioned in this manuscript that might lead to a conflict of interest.
Acknowledgements
The support of the Australian Institute of Nuclear Science and Engineering (AINSE) is acknowledged.
References
- Campas-Moya C. Romidepsin for the treatment of cutaneous T-cell lymphoma. 2009; 45: 787-95.
- Marks PA, Breslow R. Dimethyl sulfoxide to vorinostat: development of this histone deacetylase inhibitor as an anticancer drug. 2007; 25: 84-90.
- Duvic M, Vu J. Vorinostat: a new oral histone deacetylase inhibitor approved for cutaneous T-cell lymphoma. 2007; 16: 1111-20.
- Grant C, Rahman F, Piekarz R, Peer C, Frye R, Robey RW et al. Romidepsin: a new therapy for cutaneous T-cell lymphoma and a potential therapy for solid tumors. 2010; 10: 997-1008.
- Kwa FA, Balcerczyk A, Licciardi P, El-Osta A, Karagiannis TC. Chromatin modifying agents – the cutting edge of anticancer therapy. 2011; 16: 543-7.
- Marks PA. Histone deacetylase inhibitors: a chemical genetics approach to understanding cellular functions. 2010; 1799: 717-25.
- Marks PA, Xu WS. Histone deacetylase inhibitors: potential in cancer therapy. 2009; 107: 600-8.
- Banerjee A, Trivedi CM, Damera G, Jiang M, Jester W, Hoshi T, et al.. Trichostatin A abrogates airway constriction, but not inflammation in mouse and human asthma models. . 2012feb; 46: 132–8.
- Choi JH, Oh SW, Kang MS, Kwon HJ, Oh GT, Kim DY. Trichostatin A attenuates airway inflammation in mouse asthma model. 2005; 35: 89-96.
- Antos CL, McKinsey TA, Dreitz M, Hollingsworth LM, Zhang CL, Schreiber K et al. Dose-dependent blockade to cardiomyocyte hypertrophy by histone deacetylase inhibitors. 2003; 278: 28930-7.
- Backs J, Olson EN. Control of cardiac growth by histone acetylation/deacetylation. 2006; 98: 15-24.
- Haberland M, Montgomery RL, Olson EN. The many roles of histone deacetylases in development and physiology: implications for disease and therapy. 2009; 10: 32-42.
- Kong Y, Tannous P, Lu G, Berenji K, Rothermel BA, Olson EN et al. Suppression of class I and II histone deacetylases blunts pressure-overload cardiac hypertrophy. 2006; 113: 2579-88.
- McKinsey TA, Olson EN. Dual roles of histone deacetylases in the control of cardiac growth. . 2004; 259: 132–41; discussion 141–5, 163–9..
- Chuang DM, Leng Y, Marinova Z, Kim HJ, Chiu CT. Multiple roles of HDAC inhibition in neurodegenerative conditions. 2009; 32: 591-601.
- Royce SG, Dang W, Ververis K, De Sampayo N, El-Osta A, Tang MLK, et al.. Protective effects of valproic acid against airway hyperresponsiveness and airway remodeling in a mouse model of allergic airways disease. . 2011Dec 1; 6: 1463–70.
- Querfurth HW, LaFerla FM. Alzheimer's disease. 2010; 362: 329-44.
- Davies CA, Mann DM, Sumpter PQ, Yates PO. A quantitative morphometric analysis of the neuronal and synaptic content of the frontal and temporal cortex in patients with Alzheimer's disease. 1987; 78: 151-64.
- Selkoe DJ. Alzheimer's disease is a synaptic failure. 2002; 298: 789-91.
- Masliah E, Mallory M, Alford M, DeTeresa R, Hansen LA, McKeel DWJr et al. Altered expression of synaptic proteins occurs early during progression of Alzheimer's disease. 2001; 56: 127-9.
- Terry RD, Masliah E, Salmon DP, Butters N, DeTeresa R, Hill R et al. Physical basis of cognitive alterations in Alzheimer's disease: synapse loss is the major correlate of cognitive impairment. 1991; 30: 572-80.
- Bamberger ME, Landreth GE. Inflammation, apoptosis, and Alzheimer's disease. 2002; 8: 276-83.
- Castellani RJ, Rolston RK, Smith MA. Alzheimer disease. 2010; 56: 484-546.
- Butterfield DA, Griffin S, Munch G, Pasinetti GM. Amyloid beta-peptide and amyloid pathology are central to the oxidative stress and inflammatory cascades under which Alzheimer's disease brain exists. 2002; 4: 193-201.
- DeKosky ST. Pathology and pathways of Alzheimer's disease with an update on new developments in treatment. 2003; 51Suppl 1314-20.
- Doraiswamy PM. Alzheimer's disease and the glutamate NMDA receptor. 2003; 37: 41-9.
- Doraiswamy PM. The role of the N-methyl-D-aspartate receptor in Alzheimer's disease: therapeutic potential. 2003; 3: 373-8.
- Kuo MH, Allis CD. Roles of histone acetyltransferases and deacetylases in gene regulation. 1998; 20: 615-26.
- Cyr AR, Domann FE. The redox basis of epigenetic modifications: from mechanisms to functional consequences. 2011; 15: 551-89.
- Wade PA, Pruss D, Wolffe AP. Histone acetylation: chromatin in action. 1997; 22: 128-32.
- Roth SY, Denu JM, Allis CD. Histone acetyltransferases. 2001; 70: 81-120.
- Smith BC, Denu JM. Chemical mechanisms of histone lysine and arginine modifications. 2009; 1789: 45-57.
- Dokmanovic M, Marks PA. Prospects: histone deacetylase inhibitors. 2005; 96: 293-304.
- Rosato RR, Grant S. Histone deacetylase inhibitors: insights into mechanisms of lethality. 2005; 9: 809-24.
- Minucci S, Pelicci PG. Histone deacetylase inhibitors and the promise of epigenetic (and more) treatments for cancer. 2006; 6: 38-51.
- Xu WS, Parmigiani RB, Marks PA. Histone deacetylase inhibitors: molecular mechanisms of action. 2007; 26: 5541-52.
- Michan S, Sinclair D. Sirtuins in mammals: insights into their biological function. 2007; 404: 1-13.
- Frye RA. Phylogenetic classification of prokaryotic and eukaryotic Sir2-like proteins. 2000; 273: 793-8.
- Rajendran R, Garva R, Krstic-Demonacos M, Demonacos C. Sirtuins: molecular traffic lights in the crossroad of oxidative stress, chromatin remodeling, and transcription. . 2011; 2011: 368276 Epub 2011..
- Li X, Kazgan N. Mammalian sirtuins and energy metabolism. 2011; 7: 575-87.
- Finkel T, Deng CX, Mostoslavsky R. Recent progress in the biology and physiology of sirtuins. 2009; 460: 587-91.
- Anekonda TS, Reddy PH. Neuronal protection by sirtuins in Alzheimer's disease. 2006; 96: 305-13.
- Guarente L., Franklin H. Epstein Lecture: sirtuins, aging, and medicine. 2011; 364: 2235-44.
- Tippmann F, Hundt J, Schneider A, Endres K, Fahrenholz F. Up-regulation of the alpha-secretase ADAM10 by retinoic acid receptors and acitretin. 2009; 23: 1643-54.
- Donmez G, Wang D, Cohen DE, Guarente L. SIRT1 suppresses beta-amyloid production by activating the alpha-secretase gene ADAM10. 2010; 142: 320-32.
- Vingtdeux V, Marambaud P. Identification and biology of alpha-secretase. 2012; 120Suppl 134-45.
- Bonda DJ, Lee HG, Camins A, Pallas M, Casadesus G, Smith MA et al. The sirtuin pathway in ageing and Alzheimer disease: mechanistic and therapeutic considerations. 2011; 10: 275-9.
- Min SW, Cho SH, Zhou Y, Schroeder S, Haroutunian V, Seeley WW et al. Acetylation of tau inhibits its degradation and contributes to tauopathy. 2010; 67: 953-66.
- Heneka MT, O'Banion MK, Terwel D, Kummer MP. Neuroinflammatory processes in Alzheimer's disease. 2010; 117: 919-47.
- Yang F, Zhang T, Ito Y. Large-scale separation of resveratrol, anthraglycoside A and anthraglycoside B from Polygonum cuspidatum Sieb. et Zucc by high-speed counter-current chromatography. 2001; 919: 443-8.
- Yadav M, Jain S, Bhardwaj A, Nagpal R, Puniya M, Tomar R et al. Biological and medicinal properties of grapes and their bioactive constituents: an update. 2009; 12: 473-84.
- Leifert WR, Abeywardena MY. Cardioprotective actions of grape polyphenols. 2008; 28: 729-37.
- Soleas GJ, Diamandis EP, Goldberg DM. Resveratrol: a molecule whose time has come? And gone?. 1997; 30: 91-113.
- Pervaiz S, Holme AL. Resveratrol: its biologic targets and functional activity. 2009; 11: 2851-97.
- Anekonda TS. Resveratrol – a boon for treating Alzheimer's disease?. 2006; 52: 316-26.
- Richard T, Pawlus AD, Iglesias ML, Pedrot E, Waffo-Teguo P, Merillon JM et al. Neuroprotective properties of resveratrol and derivatives. 2011; 1215: 103-8.
- Jang JH, Surh YJ. Protective effect of resveratrol on beta-amyloid-induced oxidative PC12 cell death. 2003; 34: 1100-10.
- Han YS, Zheng WH, Bastianetto S, Chabot JG, Quirion R. Neuroprotective effects of resveratrol against beta-amyloid-induced neurotoxicity in rat hippocampal neurons: involvement of protein kinase C. 2004; 141: 997-1005.
- Marambaud P, Zhao H, Davies P. Resveratrol promotes clearance of Alzheimer's disease amyloid-beta peptides. 2005; 280: 37377-82.
- Green KN, Steffan JS, Martinez-Coria H, Sun X, Schreiber SS, Thompson LM et al. Nicotinamide restores cognition in Alzheimer's disease transgenic mice via a mechanism involving sirtuin inhibition and selective reduction of Thr231-phosphotau. 2008; 28: 11500-10.
- Dokmanovic M, Clarke C, Marks PA. Histone deacetylase inhibitors: overview and perspectives. 2007; 5: 981-9.
- de Ruijter AJ, van Gennip AH, Caron HN, Kemp S, van Kuilenburg AB. Histone deacetylases (HDACs): characterization of the classical HDAC family. 2003; 370: 737-49.
- Yang XJ, Seto E. Collaborative spirit of histone deacetylases in regulating chromatin structure and gene expression. 2003; 13: 143-53.
- Bolden JE, Peart MJ, Johnstone RW. Anticancer activities of histone deacetylase inhibitors. 2006; 5: 769-84.
- Mai A, Rotili D, Valente S, Kazantsev AG. Histone deacetylase inhibitors and neurodegenerative disorders: holding the promise. 2009; 15: 3940-57.
- Gao YS, Hubbert CC, Yao TP. The microtubule-associated histone deacetylase 6 (HDAC6) regulates epidermal growth factor receptor (EGFR) endocytic trafficking and degradation. 2010; 285: 11219-26.
- Hubbert C, Guardiola A, Shao R, Kawaguchi Y, Ito A, Nixon A et al. HDAC6 is a microtubule-associated deacetylase. 2002; 417: 455-8.
- Kawaguchi Y, Kovacs JJ, McLaurin A, Vance JM, Ito A, Yao TP. The deacetylase HDAC6 regulates aggresome formation and cell viability in response to misfolded protein stress. 2003; 115: 727-38.
- Guan JS, Haggarty SJ, Giacometti E, Dannenberg JH, Joseph N, Gao J et al. HDAC2 negatively regulates memory formation and synaptic plasticity. 2009; 459: 55-60.
- Li G, Jiang H, Chang M, Xie H, Hu L. HDAC6 alpha-tubulin deacetylase: a potential therapeutic target in neurodegenerative diseases. 2011; 304: 1-8.
- Ding H, Dolan PJ, Johnson GV. Histone deacetylase 6 interacts with the microtubule-associated protein tau. 2008; 106: 2119-30.
- Perez M, Santa-Maria I, Gomez de Barreda E, Zhu X, Cuadros R, Cabrero JR et al. Tau – an inhibitor of deacetylase HDAC6 function. 2009; 109: 1756-66.
- Hanger DP, Anderton BH, Noble W. Tau phosphorylation: the therapeutic challenge for neurodegenerative disease. 2009; 15: 112-9.
- Chen S, Owens GC, Makarenkova H, Edelman DB. HDAC6 regulates mitochondrial transport in hippocampal neurons. 2010; 5: e10848.
- Marks P, Rifkind RA, Richon VM, Breslow R, Miller T, Kelly WK. Histone deacetylases and cancer: causes and therapies. 2001; 1: 194-202.
- Gottlicher M, Minucci S, Zhu P, Kramer OH, Schimpf A, Giavara S et al. Valproic acid defines a novel class of HDAC inhibitors inducing differentiation of transformed cells. 2001; 20: 6969-78.
- Kramer OH, Zhu P, Ostendorff HP, Golebiewski M, Tiefenbach J, Peters MA et al. The histone deacetylase inhibitor valproic acid selectively induces proteasomal degradation of HDAC2. 2003; 22: 3411-20.
- Phiel CJ, Zhang F, Huang EY, Guenther MG, Lazar MA, Klein PS. Histone deacetylase is a direct target of valproic acid, a potent anticonvulsant, mood stabilizer, and teratogen. 2001; 276: 36734-41.
- Haggarty SJ, Koeller KM, Wong JC, Grozinger CM, Schreiber SL. Domain-selective small-molecule inhibitor of histone deacetylase 6 (HDAC6)-mediated tubulin deacetylation. 2003; 100: 4389-94.
- Namdar M, Perez G, Ngo L, Marks PA. Selective inhibition of histone deacetylase 6 (HDAC6) induces DNA damage and sensitizes transformed cells to anticancer agents. 2010; 107: 20003-8.
- Parmigiani RB, Xu WS, Venta-Perez G, Erdjument-Bromage H, Yaneva M, Tempst P et al. HDAC6 is a specific deacetylase of peroxiredoxins and is involved in redox regulation. 2008; 105: 9633-8.
- Tang W, Luo T, Greenberg EF, Bradner JE, Schreiber SL. Discovery of histone deacetylase 8 selective inhibitors. 2011; 21: 2601-5.
- Francis YI, Fa M, Ashraf H, Zhang H, Staniszewski A, Latchman DS et al. Dysregulation of histone acetylation in the APP/PS1 mouse model of Alzheimer's disease. 2009; 18: 131-9.
- Kilgore M, Miller CA, Fass DM, Hennig KM, Haggarty SJ, Sweatt JD et al. Inhibitors of class 1 histone deacetylases reverse contextual memory deficits in a mouse model of Alzheimer's disease. 2010; 35: 870-80.
- Su Y, Ryder J, Li B, Wu X, Fox N, Solenberg P et al. Lithium, a common drug for bipolar disorder treatment, regulates amyloid-beta precursor protein processing. 2004; 43: 6899-908.
- Qing H, He G, Ly PT, Fox CJ, Staufenbiel M, Cai F et al. Valproic acid inhibits Abeta production, neuritic plaque formation, and behavioral deficits in Alzheimer's disease mouse models. 2008; 205: 2781-9.
- Blaheta RA, Cinatl JJr Anti-tumor mechanisms of valproate: a novel role for an old drug. 2002; 22: 492-511.
- Johannessen CU. Mechanisms of action of valproate: a commentatory. 2000; 37: 103-10.
- Rosenberg G. The mechanisms of action of valproate in neuropsychiatric disorders: can we see the forest for the trees?. 2007; 64: 2090-103.
- Fleisher AS, Truran D, Mai JT, Langbaum JB, Aisen PS, Cummings JL et al. Chronic divalproex sodium use and brain atrophy in Alzheimer disease. 2011; 77: 1263-71.
- Ricobaraza A, Cuadrado-Tejedor M, Marco S, Perez-Otano I, Garcia-Osta A. Phenylbutyrate rescues dendritic spine loss associated with memory deficits in a mouse model of Alzheimer disease. . 2010Nov 10. [Epub ahead of print]..
- Ricobaraza A, Cuadrado-Tejedor M, Perez-Mediavilla A, Frechilla D, Del Rio J, Garcia-Osta A. Phenylbutyrate ameliorates cognitive deficit and reduces tau pathology in an Alzheimer's disease mouse model. 2009; 34: 1721-32.
- Wiley JC, Pettan-Brewer C, Ladiges WC. Phenylbutyric acid reduces amyloid plaques and rescues cognitive behavior in AD transgenic mice. 2011; 10: 418-28.
- Yao Z, Guo Z, Yang C, Tian Q, Gong CX, Liu G et al. Phenylbutyric acid prevents rats from electroconvulsion-induced memory deficit with alterations of memory-related proteins and tau hyperphosphorylation. 2010; 168: 405-15.
- Mizukami T, Orihashi K, Herlambang B, Takahashi S, Hamaishi M, Okada K et al. Sodium 4-phenylbutyrate protects against spinal cord ischemia by inhibition of endoplasmic reticulum stress. 2010; 52: 1580-6.
- Ryu H, Smith K, Camelo SI, Carreras I, Lee J, Iglesias AH et al. Sodium phenylbutyrate prolongs survival and regulates expression of anti-apoptotic genes in transgenic amyotrophic lateral sclerosis mice. 2005; 93: 1087-98.
- Wiley JC, Meabon JS, Frankowski H, Smith EA, Schecterson LC, Bothwell M et al. Phenylbutyric acid rescues endoplasmic reticulum stress-induced suppression of APP proteolysis and prevents apoptosis in neuronal cells. 2010; 5: e9135.
- Brahe C, Vitali T, Tiziano FD, Angelozzi C, Pinto AM, Borgo F et al. Phenylbutyrate increases SMN gene expression in spinal muscular atrophy patients. 2005; 13: 256-9.
- Marks PA. The clinical development of histone deacetylase inhibitors as targeted anticancer drugs. 2010; 19: 1049-66.
- Salminen A, Tapiola T, Korhonen P, Suuronen T. Neuronal apoptosis induced by histone deacetylase inhibitors. 1998; 61: 203-6.
- Broide RS, Redwine JM, Aftahi N, Young W, Bloom FE, Winrow CJ. Distribution of histone deacetylases 1–11 in the rat brain. 2007; 31: 47-58.