Abstract
The efficacy of DNA vaccines is highly dependent on the methods used for their delivery and the choice of delivery sites/targets for gene injection, pointing at the necessity of a strict control over the gene delivery process. Here, we have investigated the effect of the injection site on gene expression and immunogenicity in BALB/c mice, using as a model a weak gene immunogen, DNA encoding firefly luciferase (Luc) delivered by superficial or deep injection with subsequent electroporation (EP). Immunization was assessed by monitoring the in vivo expression of luciferase by 2D- and 3D-bioluminescence imaging (BLI) and by the end-point immunoassays. Anti-Luc antibodies were assessed by ELISA, and T-cell response by IFN-γ and IL-2 FluoroSpot in which mouse splenocytes were stimulated with Luc or a peptide representing its immunodominant CD8+ T-cell epitope GFQSMYTFV. Monitoring of immunization by BLI identified EP parameters supporting the highest Luc gene uptake and expression. Superficial injection of Luc DNA followed by optimal EP led to a low level Luc expression in the mouse skin, and triggered a CD8+ T-cell response characterized by the peptide-specific secretion of IFN-γ and IL-2, but no specific antibodies. Intramuscular gene delivery resulted in a several-fold higher Luc expression and anti-Luc antibody, but induced low IL-2 and virtually no specific IFN-γ. Photon flux from the sites of Luc gene injection was inversely proportional to the immune response against GFQSMYTFV (p < 0.05). Thus, BLI permitted to control the accuracy of gene delivery and transfection with respect to the injection site as well as the parameters of electroporation. Further, it confirmed the critical role of the site of DNA administration for the type and magnitude of the vaccine-specific immune response. This argues for the use of luminescent reporters in the preclinical gene vaccine tests to monitor both gene delivery and the immune response development in live animals.
Introduction
The role of the anatomical site of DNA vaccine delivery in the vaccine immunogenicity has been widely disputed. Both skin and muscle are suitable targets for plasmid DNA delivery.Citation1,Citation2 Skin is a very attractive site for delivery as it is an immunological barrier, which contains a high number of immunocompetent antigen-presenting cells (APCs) such as Langerhans cells. These cells constituting 1–4% of the total cells of the epidermis, greatly contribute to the induction of immune response after DNA delivery.Citation3 Muscle tissue on the other hand provides the machinery for a more efficient plasmid DNA expression. It is composed of terminally differentiated myocytesorganized into muscle fibers that persist through most of the person’s life. Degeneration after damage occurs in the limited segments of muscle fibers, the surviving segments remain viable, providing a stable environment for the continuous transgene expression.Citation4 Additionally, the syncytial nature of muscle fibers facilitates transgene dispersal from a single penetration site to a large number of neighboring nuclei within the same fiber. This dispersal mechanism has been proposed to contribute to more efficient transgene expression in muscles compared with other tissues.Citation4
Among the factors determining DNA vaccine immunogenicity are the vaccine (plasmid) design, dose and formulation, use of adjuvants, and importantly, the route of plasmid administration. A wide variety of strategies have been developed to selectively target muscle or skin, such as complementing plasmid DNA with lipids, sugars, salts and various drugs. Targeting could be also achieved by the use of different delivery techniques: with Biojector, “gene gun,” via a dendritic cell-targeting topical-vaccine administration, to mucosal surfaces with drops and suppositories, or classically by needle injections.Citation5-Citation8 Recent studies have shown that gene uptake can be strongly promoted by in vivo electroporation, a transfection method in which the electrical pulses are applied over the inoculation site. This leads to two distinct outcomes: (1) creation of transient pores in the cell membrane of target cells, thus facilitating plasmid permeation; (2) reversible damage of nascent tissue, generating a “danger signal” which produces an adjuvant effect recruiting additional APCs to the site.Citation9 The latter results in an increased uptake and expression of the immunogen: when administered after an intradermal or intramuscular injection, electroporation improves gene immunogen expression by 100–1000-fold regardless of the gene dose.Citation10 Apart from the predominant cell type of the target tissue, APCs may also be transfected upon application of the electric pulses.Citation11 Through this, electroporation may aid to reach the threshold required to induce the innate, and adaptive immune response against the plasmid-encoded antigens.
In small laboratory animals the transfection efficiency can be monitored in vivo by using bioluminescent imaging (BLI). It allows for frequent high throughput non-invasive monitoring of bioluminescent reporter protein expression over long periods of time and, therefore, presents an attractive alternative to the ex vivo methods of expression monitoring which require killing of animals at each time point with no possibility for a longitudinal individual follow-up. One of the most often used reporters for BLI is the firefly luciferase. The luminescent light it emits is detectable even after passing through tissue.Citation12,Citation13 This makes the signal traceable even when its origin is in the tissues beyond the skin, which allows for the use of more sophisticated methods of reporter monitoring such as bioluminescence tomography (BLT). While two-dimensional (2D) imaging allows for a rapid screening of protein expression, BLT provides detailed three-dimensional (3D) information about the anatomical location of the signal. Importantly, the low immunogenicity of luciferase secures a longitudinal expression monitoring not affected by the extermination of expressing cells by the luciferase specific immune response.Citation14
The aim of this study was to target DNA immunogen delivery to skin and to muscles, test the possibility to monitor the delivery by BLI, as well as to use BLI to study the development of the specific immune response. We took the advantage of firefly luciferase visibility to in vivo BLI and use it as a model gene immunogen. Although luciferase is a weak immunogen (which allows for its broad use as the reporterCitation15), multiple reports have shown that if highly expressed, luciferase may reach critical levels which trigger host immune responses.Citation4,Citation13 The use of luciferase gene eliminated the need to deliver two separate plasmids (one as an immunogen and the other as a reporter) and allowed to directly assess the delivery and expression of the gene and the effects on expression of the emerging immune response. The ability to track antigen-expressing cells enabled us to associate parameters of in vivo bioluminescence imaging with the parameters of anti-luciferase immune response acquired by immunoassays, in order to develop a surrogate method to characterize DNA immunogen performance by non-invasive, animal-sparing methods.
Results
Efficiency of transfection is dependent on skin resistance
Before starting longitudinal immunogenicity studies we wanted to optimize the electroporation protocol used for the electrotransfer of injected DNA. Our previous data obtained using DermaVax electroporator (Cellectis) implied the existence of a correlation between the parameters of electroporation such as skin resistance, and the efficiency of DNA transfer.Citation16 DermaVax electroporator provides two different skin resistance values: (1) pre-pulse resistance, which shows a real-time estimation of the resistance when electrodes come into contact with the tissues; (2) monitored pulse resistance – the actual resistance value at the time of electric pulse delivery. We have investigated if the electroporation efficiency depends on the skin resistance. For this, using the data from the earlier experiments involving administration of pVax-Luc,Citation14 we analyzed the dependence of luciferase gene expression (the average photon flux from the injection site on day 4 post injection) on the estimated pre-pulse and the monitored pulse resistance values registered by Derma Vax. Lower resistance values correlated with the higher luciferase expression signifying a better DNA transfer (R = - 0.52, monitored pulse resistance, p < 0.5; ). This indicated that for an efficient gene expression, monitored resistance values at the pulse have to be below 1000 Ω, and the pre-pulse resistance values < 3000 Ω.
Figure 1. Dependence of expression of luciferase gene assessed as the total photon flux to the estimated pre-pulse and monitored skin resistance during electroporation (Derma Vax). Analysis of the monitored skin resistance and average photon flux data from pervious Luc gene injection experiments involving 232 injectionsCitation14 (A); Variance of average flux from the injection sites four days after Luc gene injection followed by pre-pulse resistance controlled vs. uncontrolled electroporation (B); Correlation between total photon flux (photons/sec) and electroporation parameters 2 h after injection in mice receiving deep (C) and superficial (D) Luc gene injections. Correlations were done using Spearman rank order test, p < 0.05 were considered significant.
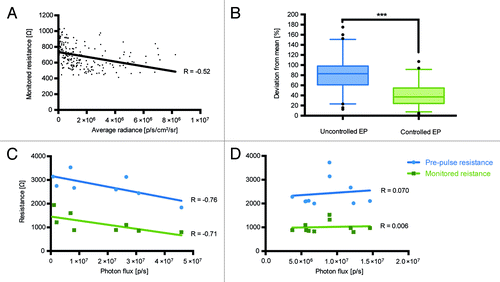
To depict the effect of skin resistance control on gene delivery and expression, we performed a comparative study in which we injected Luc gene into mice and performed electroporation in the pre-pulse resistance controlled (pre-pulse resistance values < 3000 Ω; n = 28) vs. uncontrolled fashion (n = 32). The variances of the photon flux from the individual injection sites from the average in the groups with controlled vs. uncontrolled deliveries were compared. A controlled delivery resulted in a significantly lower variance between the levels of local luciferase expression (p = 0.007 t-test; p = 0.0004 in the nonparametric Kruskal Wallis test), i.e., ensured a more standardized plasmid delivery ().
We further evaluated if the observed correlation between skin resistance and gene expression depended on the localization of expression. For this, we introduced Luc gene into mice by superficial injections (targeting skin tissue; n = 10) or deep (targeting muscle tissue; n = 10) and performed the electroporation at the pre-pulse skin resistance ranging from 1800 to 3700 Ω and the monitored resistance from 700 to 2000 Ω. Pre-pulse and monitored resistance values were then correlated with the luciferase gene expression measured as the total photon flux from the injection/electroporation site 2 h to 22 d post immunization. The analysis revealed that for the deep injections targeting muscle lower resistance values correlated with the higher DNA transfer and luciferase expression (R = -0.76, pre-pulse resistance; R = -0.71, monitored pulse resistance, p < 0.5; ). This dependence was, however, not observed in DNA delivery targeting skin ().
Immunogen expression levels depend on the site of injection
We investigated the kinetics of luciferase expression after transfecting its gene into different anatomical sites, skin and muscles. Luciferase expression was measured by BLI at various time points after transfection. Both mice receiving DNA by superficial injections targeting skin and by deep injections targeting muscles were found to express luciferase at the initial measurement done two hours post immunization (−C). Notably, muscle fibers were found to support somewhat stronger luciferase expression at this time point (9.5 × 106 photons/sec difference; p = 0.085, t-test). Statistically significant difference between the mean luminescence intensity in muscle and in superficial/close to skin tissues was reached 20 h post immunization and continued up to day 3 post immunization (p < 0.05; ). The latter was the time point for the highest level of luciferase expression in mice receiving deep injections. The levels of luciferase expression during the next three measurements at 6, 9, and 15 d post immunization remained unchanged (). Unlike deep-injected mice, superficial injections resulted in a lower peak of expression one day after DNA delivery, which was sustained as a plateau until day 9 when level of luciferase expression started to drop. Mice were followed until day 22, on which somewhat higher luciferase expression was still detected in animals receiving deep as compare with superficial injections (8.98 x 106 photons/sec difference, p = 0.11; ). These data demonstrate that in comparison to the superficial skin-targeting injection, plasmid immunogen delivered via deep injection results in the higher and more prolonged protein expression, and also gives an early peak in the expression of the immunogen.
Figure 2. Measurement of luminescence in mice receiving deep and superficial injections. The activity of luciferase was monitored by in vivo bioluminescent imaging of mice receiving Luc gene by deep (A) or superficial (B) injections. The images represent a composite of luminescence data (photons/sec) overlaid with a photograph of the subjects. Deep injections resulted in higher and longer-lasting expression of luciferase (C).
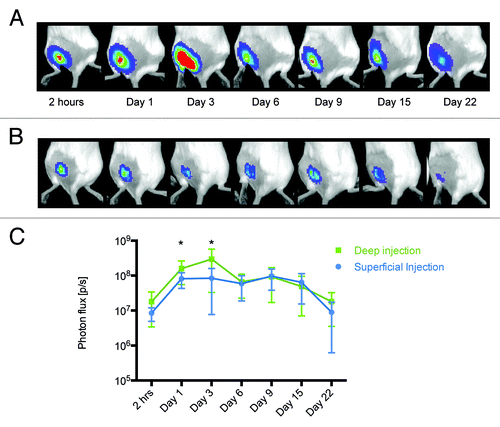
In order to validate the correctness of the injection implementation, we measured the location/depth of the luminescence site by bioluminescent tomography (BLT). In superficial injections, luciferase expression was localized at a depth of 1.0–1.5 mm, whereas deep injections resulted in the protein expression at a depth of 2.0–3.0 mm (). Localization of luciferase expression was confirmed upon surgical excision of the skin and muscle tissue followed by ex vivo BLI monitoring of the specimens ().
Figure 3. Luciferase expression in BALB/c mice receiving deep or superficial injections of Luc gene. The depth of luciferase expression was verified by 3D BLI 2 h after Luc gene administration. The “center of mass” of luciferase expression localized between 2.0–3.0 mm in mice receiving deep (A) and between 1.0–1.5 mm in mice receiving superficial Luc gene injections (D). Skin was excised post mortem and monitoring was repeated directly after. In mice receiving deep injections (A) luciferase activity was localized to the muscle tissues (B) while none was registered in the skin (C). On contrary, in superficially injected animals (D) luciferase activity was recorded in the skin (F) but not in the muscles (E).
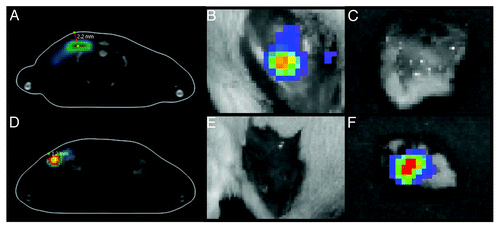
BLT also allowed us to utilize another novel imaging parameter - the transfected volume which measures the space occupied by the transfected/expressing cells and can serve for quantification of their number. After injecting identical volumes of luciferase gene solutions we detected a 2-fold higher transfection volume after the deep as compared with the superficial injections (day 1 after gene administration, p < 0.05; ). In superficial skin-targeting injections, this volume remained relatively unchanged with time, whereas in muscle it gradually decreased, reaching values equal to those in skin by day 9 and then increasing to its initial values by the end of the follow-up period (). It must be noted that the transfection volume included all cells that emitted luminescence and did not discriminate between high and low levels of signal intensity.
Figure 4. Volume of the tissues transfected after superficial and deep Luc gene injections followed by electroporation. Three-dimensional bioluminescence imaging was used to measure the physical volume of cells expressing luciferase. One day after injection of 20 μg DNA (20 μl) a statistically significant difference in volume of transfected cells was observed. This volume measured 17 mm3 in deep injections and 8 mm3 in superficially injected mice.
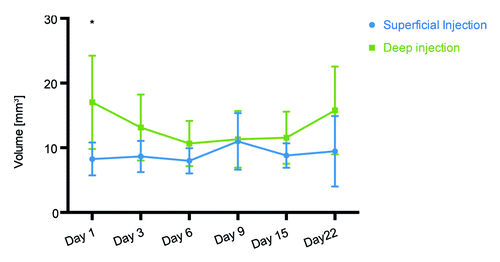
Immune response induction by superficial and deep injection
Next, we compared superficial and deep injections in their ability to induce the luciferase-specific immune response. To assess cellular responses, we measured the ability of splenocytes of immunized mice to secrete type 1 cytokines (IFN-γ or IL-2) when stimulated with luciferase-derived peptide corresponding to its CTL epitope recognized in miceCitation17 or with the recombinant luciferase to monitor CD4+ T-cell response. Superficial immunization induced a 3-fold higher IFN-γ secretion compared with the deep immunization route when splenocytes were stimulated with the GFQSMYTFV peptide (p < 0.05; ). Recombinant protein stimulated low/no IFN-γ response (). Superficial injections were also shown to induce a more potent response against both CD8+ and CD4+ T-cell epitopes in comparison to deep injections in terms of IL-2 secretion ().
Figure 5. Immunogenicity of luciferase DNA in BALB/c mice immunized by superficial or deep injections of luciferase gene followed by electroporation. On day 23 post immunization splenocytes were harvested from mice receiving superficial (n = 9) and deep (n = 7) injections of luciferase-coding pVax-Luc and vector-only controls (pVax1, n = 6). Responses of splenocytes stimulated by a peptide representing CD8+ epitope of Luc (GFQSMYTFV) or recombinant luciferase measured by FluoroSpot: secretion of IFN-γ (A), IL-2 (B); Optical density of luciferase-specific antibodies measured by ELISA (C).
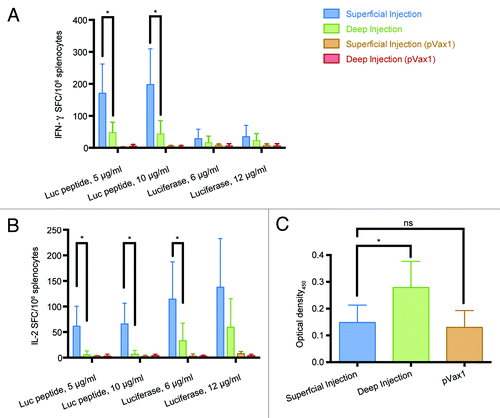
Importantly, there was no difference in the immunogenic performance with respect to production of IFN-γ or IL-2 in mice subjected to BLI only, or to BLT implying exposure to radiation at six occasions during the immunization cycle with the total radiation dose of 0.138 Gy (data not shown).
To investigate humoral immune responses we collected serum samples from both groups and determined the levels of anti-luciferase antibodies. In terms of humoral response, only deep immunization alone was able to raise any luciferase-specific antibodies while sera from superficially injected mice were indistinguishable from those receiving the injections of empty vector ().
These results show that superficial injections of weak gene immunogens targeting skin support weaker and possibly shorter protein expression as compared with muscles, but perform better in inducing cellular IFN-γ and IL-2 responses. However, they fail to induce a humoral response. On the other hand, the delivery of such immunogens into muscle tissue supports higher and more long-lasting protein expression, but low/no cellular immune response, however, it can trigger an antibody response albeit at a low level.
Correlation between BLI parameters and immune response
Subsequent to imaging and assessment of immune responses in splenocytes and sera we analyzed the correlations between in vivo BLI/BLT and the magnitude of immune response measured in the in vitro tests. The BLI parameters utilized included bioluminescence intensity (photons/sec) and volume of the transfected tissue (mm3).
Mice receiving superficial DNA injections demonstrated a strong inverse correlation between the IFN-γ responses and bioluminescence intensity in response to both luciferase-derived peptide and the recombinant protein (R = -0.61; R = -0.71, respectively). This correlation became evident at 3 d post immunization and reappeared 22 d post immunization (), and aided to the establishment of the experimental end-point. The profile of correlations between IFN-γ responses and the luminescence intensity at these two time points displayed a high degree of similarity. This dependency was less pronounced for splenocytes recognition of the recombinant luciferase, where the correlation coefficient between luminescence intensity and anti-luciferase cytokine response satisfied our +/−0.6 threshold (designating p values < 0.05) only at 22 d post immunization.
Figure 6. Heatmap of correlations between immune response as measured by slenocyte IFN-γ and IL-2 production (FluoroSpot) and parameters of BLI such as total photon flux (A) and volume of transfected tissue (B). Green indicates an inverse correlation between IFN-γ/IL-2 and BLI parameters (R < 0), whereas red signifies a direct correlation between IFN-γ/IL-2 and BLI parameters (R > 0). Luminescence intensity and transfection volume were measured by BLI and BLT at 7 different time points between 2 h after immunization and 22 d after immunization. The decrease of luminescence was associated with the development of antigen-specific immune responses resulting in a strong inverse correlation between immune response and total photon flux (A). The relationship became apparent 3 d post immunization and showed some degree of variation until day 22, when correlation coefficients returned to their values from day 3. Transfected tissue volume (B) correlated directly with immune response 9 d post immunization, with the exception of IFN-γ levels in response to stimulation by recombinant luciferase, which correlated directly with the transfected volume 22 d post immunization. Transfection volume was measured only once during the first 24 h post immunization (Day 1) to reduce the initial exposure of mice to radiation.
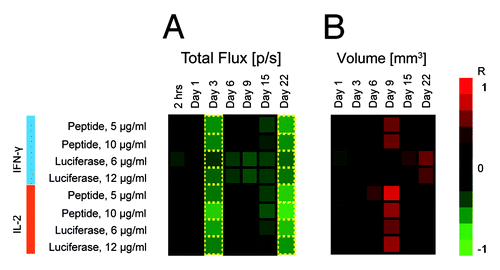
No correlations were found between the immune responses and the volume of transfected tissue during the first week post immunization. However, at day 9 a strong correlation appeared between the transfected volume and the levels of secretion of IL-2 by both peptide and protein-stimulated splenocytes (peptide: R = -0.83/-0.66; protein: R = -0.55/-0.68, p < 0,05; ).
Low level of cellular responses registered in mice receiving deep injections did not allow investigating the correlations between BLI parameters and the immune response for this group. Antibody levels did not show a strong correlation with either bioluminescence intensity or transfected tissue volume in the early and intermediate time points, but demonstrated an inverse correlation to bioluminescence intensity on day 22 post immunization (R = -0.84; data not shown).
Discussion
Increasing knowledge in the field of molecular biology has turned DNA vaccines into a promising approach for treatment and prevention of infectious diseases and cancer.Citation18 However, immunogenicity in large animals and humans is still a concern, hurdling the development of this vaccine modality. DNA delivery technique is one of the parameters strongly modulating gene vaccine immunogenicity.Citation19,Citation20
In the present study we compared the expression and immunogenic performance of a weak immunogen when delivered by injections targeted to skin or to muscle, each followed by electroporation. Electroporation has been shown to dramatically improve DNA uptake into the cells. It also functions as an adjuvant providing a “danger signal” able to recruit macrophages, dendritic cells and lymphocytes to the site of charge deliveryCitation9,Citation21 thus enhancing cellular and humoral immune response. In this study we examined the relationship between the conditions of gene immunization (type of injection, electroporation settings) and the parameters of BLI which assess the uptake and expression of luciferase gene. Monitoring of the effect of skin resistance in electroporation by BLI revealed an inverse correlation between the resistance and luciferase expression in the muscle tissues (R = -0.71, pre-pulse load; R = -0.65, monitored pulse load). This pointed at the necessity to decrease the estimated pre-pulse resistance of the skin, which would increase the electric current during pulse. By making efforts to minimize estimated skin resistance prior to electroporation, a detail usually overlooked in the process, we have managed to significantly increase plasmid DNA uptake by target tissues. Importantly, BLI monitoring of the optimized electroporation procedure demonstrated also a significant decrease in the variance between single plasmid deliveries. BLI monitoring, thus, provided a tool for the standardization of gene delivery.
We can speculate that lower values of skin resistance not only allowed for a higher electric current (higher charge) and therefore a better gene uptake, but were also more beneficial for the expressing tissues. High skin resistance resulting in Joule heating has been associated with increased thermal damage and cell death after electroporation as opposed to the reversible tissue damage that normally occurs.Citation22 Moreover, excessive heating due to high tissue resistance can potentially damage plasmid DNA.Citation23 Interestingly, keeping to lower resistance values when electroporating skin/superficial tissues did not improve the expression. This could be due to the characteristics of the needle-shaped electrodes used here. Being 2 mm long, they targeted muscle tissue more efficiently than skin which allowed to reveal correlations of skin resistance with the expression in muscles. Further experiments with a different set of electrodes are needed to optimize the electroporation settings required for skin applications.
Transfection of skin by superficial injection targeted to skin resulted in a low-level gene expression with a tendency to subside three weeks after immunization. Being only weakly immunogenic, luciferase was still persisting at the end point of the 22-d follow up, however, its quantities were significantly lower than those detected in the muscle tissues. This can be expected as in comparison with muscle cells, epidermal and dermal cells divide much more frequently and are a subject to constant cell death and desquamation, in the case of keratinocytes.Citation24
Another rationale for higher protein expression in muscle tissue might be an enhanced susceptibility of this anatomical location to the in vivo transfection. Injection of fluid into the muscle and skin results in different outcome in terms of its distribution. Injection of 20 μl of DNA solution clearly exceeds the fluid capacity of both skin and muscle and results in noticeable tissue swelling. It has been shown that this increased pressure in muscle promotes the dispersion of fluid not only through the entire muscle but also into interstitial spaces.Citation25 This enhances the possibility of more cells taking up the DNA and justify a higher volume of the transfected/expressing cells registered after deep injections.
Superficial tissues such as skin have a much lower fluid capacity; injections delivered in such sites are more prone to partial leakage as opposed to the internal dispersal. Furthermore, physical pressure applied by the electrode surface in the process of electroporation further increases the chances of such leakage. Thus, fewer cells come in contact with the injected DNA, which would explain our observations of a low transfected/expressing cell volume.
The anatomical site of gene delivery, the number (volume) of transfected cells and the level of gene expression therein shape the subsequent immune response. Longer duration and higher amounts of antigen expression appear not to be crucial for the T-cell response to a plasmid vaccine. Rather, protein expression needs to occur for a time sufficient for the induction of an adaptive immune response. Instead of the high expression, skin offers a benefit of a high number of residing antigen presenting cells, which support a strong antigen response even for a weak immunogen expressed at a comparatively low level, as was confirmed by the present study.
On the contrary, only mice receiving deep injections targeted to muscle were able to develop a specific although weak antibody response. This suggests that seroconversion requests a higher level of antigen expression than T cell response (i.e., there possibly exists a threshold expression level under which the antigen does not induce the antibody production). Previous reports have also implied that induction of antibodies relies on the longitudinal high-level expression of the antigen.Citation26 Miller et al. have shown that the antigen expression levels were indeed a determining factor for the induction of antibody response. Beta-galactosidase (β-gal) was expressed in muscle from the plasmids in which the gene was under the immediate early promoter of CMV (CMVIE), major late promoter of adenovirus, or early promoter of SV40. Promoters supported different levels of β-gal expression both in vitro and in vivo with CMVIE promoting the highest β-gal expression levels. Mice receiving plasmid DNA were able to raise detectable cellular responses to β-gal irrespective of the origins of the promoter, however, only mice immunized with CMVIE-regulated β-gal gene responded with anti-β-gal antibodies.Citation27 The importance of the levels of antigen expression has recently been emphasized by Hallengärd et al. who showed that an enhanced delivery of plasmid DNA into BALB/c mice by the Biojector with subsequent electroporation could considerably boost the humoral immune response.Citation28 Myocytes are much more efficient in expressing transfected antigens at higher levels than epidermal cells,Citation4 this explains seroconversion in mice immunized by intramuscular plasmid injections. Our results accentuate the importance of antigen expression level for induction of specific antibodies, and point at the necessity to optimize DNA vaccine delivery to achieve an enhanced humoral immune response.
In vivo imaging of luciferase enables the continuous monitoring of antigen turnover without using too many animals in the process. This may provide an instrument for monitoring immune response as the immune-mediated extermination of the expressing cells. To this end, we have investigated the relationship between the parameters of BLI taken in dynamics and the immune data acquired by the end-point immunoassays. In skin targeted immunizations, induction of IFN-γ response was correlated with a low level of protein expression (low luminescence intensity) at days 3 to 22 post immunization. On the contrary, in muscle, IFN-γ responses after muscle immunization required relatively high levels of luciferase expression (high luminescence intensity) first two weeks post immunization. In terms of the volume of transfected/expressing cells, both skin and muscle showed an inverse correlation of immune response with the luminescence intensity, i.e., a small volume of transfected/expressing cells 3–9 d post immunization signified higher levels of cytokine secretion. These results demonstrate a close correlation between the pattern of antigen expression assessed here by the parameters of BLI and BLT, and immune responses recorded by the immunoasays. Importantly, radiation received by mice subjected to computer tomography during BLT did not appear to affect the results of the end-point immune assays. Altogether, this stresses the utility BLI/BLT parameters assessing gene immunogen/reporter expression signatures for prediction of the type and magnitude of immune responses. Besides, building of an extensive base of expression signatures acquired through BLI/BLT can reliably deduce the localization of antigen expression as shown here.
We foresee the use of BLI/BLT parameters as the alternative to the current histological assays aimed to localize protein expression and as a surrogate marker of the outcome of DNA immunization. Application of the method would help to omit the immune assays for poorly performing immunogens, setting new end-points for the gene immunogen trials in small laboratory animals. Altogether, this would significantly reduce and refine animal experimentation, as well as time, labor and reagent costs spent in the preclinical DNA vaccine trials.
Methods
Animals
Eight-week-old BALB/c mice were purchased from the breeding facility of the Department of Microbiology, Tumor and Cell biology (Karolinska Institute) and housed under a light-dark (12h/12h) cycle with ad libitum access to water and food. Animals were anesthetized by a mixture of 4% isofluorane with oxygen and maintained in 2.3% isofluorane flow administered through facial masks during all intradermal/intramuscular injections and electroporations. All experimental procedures at the Karolinska Institute were approved by the Ethical Committee for the Animal Research of the Northern Stockholm (Stockholms Norra Djurförsöksetiska Nämnd).
Plasmid DNA
The luciferase-coding plasmid, pVax-luc 4,663 bp (pVaxLuc) constructed by inserting the cDNA of firefly luciferase from pGL2-basic vector (Promega, #E1641) into vector pVAX1 (Invitrogen, #V260–20) was kindly provided by Roos AK (Karolinska Institutet). The pVAX1 vector contains a human cytomegalovirus immediate/early promoter and a polyadenylation signal from the bovine growth hormone gene.Citation29
Gene immunization and in vivo electroporation
In experiment 1, groups of 8-week old female BALB/c mice were injected in the skin with pVax-Luc (10 μg in 20 μl PBS) on two sites to the left and to the right of the base of the tail. DNA injections were performed as was described previously.Citation29 Immediately after injection delivery, the immunization sites were electroporated by placing a needle array electrode over the skin and applying 2 pulses of 1125 V/cm (50μs interval) and 8 pulses of 275 V/cm (10μs interval). The needle arrays consisted of eight 2-mm pins arranged in 2 rows (1.5 × 4mm gaps) (BTX, #47-0040). Electrical pulses were generated by the DERMA VAX Clinical DNA vaccine delivery system (Cellectis Glen Burnie). Electroporation was performed in a controlled (keeping pre-pulse skin resistance < 3000 Ω; n = 14, 28 sites) vs. uncontrolled (n = 16, 32 sites) fashion.
In experiment 2, groups of 8-week old female BALB/c mice were immunized by single intradermal (n = 10) and intramuscular (n = 10) injections with 20μg pVax-Luc dissolved in 20μl PBS made on the back, near the base of the tail, using a 29G insulin syringe (Micro-Fine, BD, #037-7614). A matching group of the control mice received superficial or deep injections of the empty vector pVax1. Electroporation was performed at the pre-pulse skin resistance ranging from 1800 to 3700 Ω and the actual resistance from 700 to 2000 Ω.
Real-time in vivo imaging
In vivo imaging of bioluminescence was performed with a highly sensitive CCD camera, mounted in a light-tight chamber (IVIS Spectrum CT, Caliper LifeSciences). Anesthesia was induced by 4% isofluorane and maintained by 2.3% isofluorane throughout the imaging procedure. Ten minutes prior to the capturing the luminescent signal mice were injected intraperitoneally with the solution of D-luciferin (PerkinElmer, #122796) in PBS at a dose of 150 mg/kg mouse weight. In experiment 1, animals were monitored first time on day 4, and in experiment 2, two hours after immunization and then on days 1, 3, 6; and in both experiments, on days 9, 15, and 22. Before the acquisition of bioluminescence, selected mice (n = 3–4 per group per time point) were subjected to a microCT scan, delivering 23 mGy of radiation. Camera exposure time was automatically determined by the system and varied between 1–60 sec depending on the intensity of the bioluminescent signal. Four mice at a time were scanned in 2D-, and two, in the 3D-imaging. Regions of interest were localized around the injections sites and were quantified as luminescence flux in photons/s. CT/BLI data were processed using the Living Image® software version 4.1 (Caliper Life Sciences) to generate values of signal intensity, depth, and volume of the transfected/expressing area.
IFN-γ and IL-2 FluoroSpot assay
Mice were sacrificed 23 d post immunization, spleens were collected and cellular immune responses were measured by interferon gamma (IFN-γ) and interleukin 2 (IL-2) FluoroSpot assay according to the manufacturer’s directions (MabTech AB, # FS-4142-10) as previously described.Citation30 Briefly, polyvinylidene difluoride plates were treated with ethanol and coated with monoclonal antibodies for IFN-γ (AN18) and IL-2 (1A12) detection. A total of 1 × 105 splenocytes per well were plated and stimulated for 20 h with antigens: recombinant luciferase (Promega, #E1701) at 6 μg/ml and 12 μg/ml and luciferase-derived peptide GFQSMYTFV (GL Biochemical) at 5 μg/ml and 10 μg/ml of RPMI.Citation17 The costimulatory anti-CD28 antibody was added to the cells during the incubation. Bound cytokines were detected with fluorescein isothiocyanate (FITC)-labeled antibody (R4-6A2) and biotynylated antibody (5H4) followed by anti-FITC antibody conjugated to a green fluorochrome and streptavidin conjugated to a red fluorochrome. Finally, the number of spot-forming cells was detected using an iSpot reader (AID GmbH) allowing for the simultaneous analysis of cells secreting both cytokines.
Humoral immune responses
Blood samples were drawn by cardiac puncture at the end-point of the experiment on day 22 post immunization. Serum samples were obtained and frozen at -20 °C until thawed for ELISA. Plates were coated with 0.4 μg/ml recombinant luciferase (Promega, #E1701) at 4 °C overnight. Plates were washed and blocked with PBS containing 0.05% Tween 20, 0.5% of BSA and 1% goat serum for 1h at 37 °C. Diluted serum samples were then added and incubated at 4 °C overnight. After washing, goat anti-mouse immunoglobulins conjugated to HRP (1:3000; Dako, #P0447) was added, and plates were incubated at 37 °C for 1 h. Finally, plates were washed and developed with 3,3′,5,5′-tetramethylbenzidine (Medico-Diagnostic Laboratory). After 15 min, the reaction was stopped by adding H2SO4 and the OD450 nm was determined.Citation27
Statistical analysis
Two-tailed Student’s t-tests were employed to investigate statistical differences. Microsoft Excel 12 (Microsoft) and Prism 6 (GraphPad) were used to manage and analyze data.
To compare single injection/electroporation sites in mice receiving pre-pulse resistance controlled vs. uncontrolled electroporations, average photon flux from the injection sites per group and a variance of each individual site from the average were calculated in radians/sec/cm2, and in % to the average flux value (taken for 100%). Absolute variance values between the groups were compared using a parametric t-test and the nonparametric Kruskal Wallis test.
The nonparametric Spearman’s rank order test was used to determine correlation coefficients between parameters including: photon flux, transfection volume, IFN-γ spot forming cells (SFCs), and IL-2 SFCs. Statistical significance was defined at the standard 5% level.
Conclusions
Here, we have demonstrated that in vivo bioluminescence imaging permits to control the accuracy of gene delivery and transfection and thus to standardize the immunization process. This results in a significantly lower variance between the animals within one group in the gene expression aiding for a more consistent immune response. Further, this study demonstrates the critical role of the accuracy of DNA vaccine delivery for the type and magnitude of the vaccine-specific immune response. Superficial gene injection targeted to skin led to a potent T cell response even in response to weak DNA immunogens expressed at a low to moderate level whereas a deeper injection targeting muscle resulted in a poor immunogenic performance. We found cellular immune response to correlate with the loss of the bioluminescent signals from the immunizations sites. This argues for the use of luminescent reporters in preclinical gene vaccine tests to monitor not only gene delivery, but also the magnitude of the cellular immune response and its development over time in live animals. This can significantly diminish the animal use and the number of immune tests to perform in preclinical testing of genetic vaccines.
Acknowledgments
The authors gratefully acknowledge the financial support from the Swedish Research Council (grant K2011-79X-21744-01-6); Russian Foundation for Basic Research (grant 13-04-01523); and the New Visby program of the Swedish Institute.
Disclosure of Potential Conflicts of Interest
No potential conflicts of interest were disclosed.
References
- Lambert PH, Laurent PE. Intradermal vaccine delivery: will new delivery systems transform vaccine administration?. Vaccine 2008; 26:3197 - 208; http://dx.doi.org/10.1016/j.vaccine.2008.03.095; PMID: 18486285
- McCluskie MJ, Brazolot Millan CL, Gramzinski RA, Robinson HL, Santoro JC, Fuller JT, et al. Route and method of delivery of DNA vaccine influence immune responses in mice and non-human primates. Mol Med 1999; 5:287 - 300; PMID: 10390545
- Vandermeulen G, Staes E, Vanderhaeghen ML, Bureau MF, Scherman D, Préat V. Optimisation of intradermal DNA electrotransfer for immunisation. J Control Release 2007; 124:81 - 7; http://dx.doi.org/10.1016/j.jconrel.2007.08.010; PMID: 17854939
- Lu QL, Bou-Gharios G, Partridge TA. Non-viral gene delivery in skeletal muscle: a protein factory. Gene Ther 2003; 10:131 - 42; http://dx.doi.org/10.1038/sj.gt.3301874; PMID: 12571642
- Abdulhaqq SA, Weiner DB. DNA vaccines: developing new strategies to enhance immune responses. Immunol Res 2008; 42:219 - 32; http://dx.doi.org/10.1007/s12026-008-8076-3; PMID: 19066740
- Lori F. DermaVir: a plasmid DNA-based nanomedicine therapeutic vaccine for the treatment of HIV/AIDS. Expert Rev Vaccines 2011; 10:1371 - 84; http://dx.doi.org/10.1586/erv.11.118; PMID: 21988301
- Hinkula J. Clarification of how HIV-1 DNA and protein immunizations may be better used to obtain HIV-1-specific mucosal and systemic immunity. Expert Rev Vaccines 2007; 6:203 - 12; http://dx.doi.org/10.1586/14760584.6.2.203; PMID: 17408370
- Babiuk LA, Pontarollo R, Babiuk S, Loehr B, van Drunen Littel-van den Hurk S. Induction of immune responses by DNA vaccines in large animals. Vaccine 2003; 21:649 - 58; http://dx.doi.org/10.1016/S0264-410X(02)00574-1; PMID: 12531334
- Liu J, Kjeken R, Mathiesen I, Barouch DH. Recruitment of antigen-presenting cells to the site of inoculation and augmentation of human immunodeficiency virus type 1 DNA vaccine immunogenicity by in vivo electroporation. J Virol 2008; 82:5643 - 9; http://dx.doi.org/10.1128/JVI.02564-07; PMID: 18353952
- Mathiesen I. Electropermeabilization of skeletal muscle enhances gene transfer in vivo. Gene Ther 1999; 6:508 - 14; http://dx.doi.org/10.1038/sj.gt.3300847; PMID: 10476210
- Grønevik E, Tollefsen S, Sikkeland LIB, Haug T, Tjelle TE, Mathiesen I. DNA transfection of mononuclear cells in muscle tissue. J Gene Med 2003; 5:909 - 17; http://dx.doi.org/10.1002/jgm.416; PMID: 14533200
- Mezzanotte L, Que I, Kaijzel E, Branchini B, Roda A, Löwik C. Sensitive dual color in vivo bioluminescence imaging using a new red codon optimized firefly luciferase and a green click beetle luciferase. PLoS One 2011; 6:e19277; http://dx.doi.org/10.1371/journal.pone.0019277; PMID: 21544210
- Bloquel C, Trollet C, Pradines E, Seguin J, Scherman D, Bureau MF. Optical imaging of luminescence for in vivo quantification of gene electrotransfer in mouse muscle and knee. BMC Biotechnol 2006; 6:16; http://dx.doi.org/10.1186/1472-6750-6-16; PMID: 16524461
- Starodubova E, Krotova O, Hallengärd D, Kuzmenko Y, Engström G, Legzdina D, et al. Cellular immunogenicity of novel gene immunogens in mice monitored by in vivo imaging. Mol Imaging 2012; 11:471 - 86; PMID: 23084248
- Roos AK, Eriksson F, Timmons JA, Gerhardt J, Nyman U, Gudmundsdotter L, et al. Skin electroporation: effects on transgene expression, DNA persistence and local tissue environment. PLoS One 2009; 4:e7226; http://dx.doi.org/10.1371/journal.pone.0007226; PMID: 19789652
- Isaguliants M. In vivo monitoring of immune response in preclinical trials of genetic vaccines. In: DNA Vaccines 2012: The 21st Century Vaccine Technology: Expanding Research Horizons and Pathways to Product Licensure. San Diego, 2012.
- Limberis MP, Bell CL, Wilson JM. Identification of the murine firefly luciferase-specific CD8 T-cell epitopes. Gene Ther 2009; 16:441 - 7; http://dx.doi.org/10.1038/gt.2008.177; PMID: 19129859
- Stan R, Wolchok JD, Cohen AD. DNA vaccines against cancer. Hematol Oncol Clin North Am 2006; 20:613 - 36; http://dx.doi.org/10.1016/j.hoc.2006.02.004; PMID: 16762727
- Buchan S, Grønevik E, Mathiesen I, King CA, Stevenson FK, Rice J. Electroporation as a “prime/boost” strategy for naked DNA vaccination against a tumor antigen. J Immunol 2005; 174:6292 - 8; PMID: 15879128
- Bråve A, Hallengärd D, Gudmundsdotter L, Stout R, Walters R, Wahren B, et al. Late administration of plasmid DNA by intradermal electroporation efficiently boosts DNA-primed T and B cell responses to carcinoembryonic antigen. Vaccine 2009; 27:3692 - 6; http://dx.doi.org/10.1016/j.vaccine.2009.04.013; PMID: 19428161
- Murtaugh MP, Foss DL. Inflammatory cytokines and antigen presenting cell activation. Vet Immunol Immunopathol 2002; 87:109 - 21; http://dx.doi.org/10.1016/S0165-2427(02)00042-9; PMID: 12072225
- Becker SM, Kuznetsov A. Thermal damage reduction associated with in vivo skin electroporation: A numerical investigation justifying aggressive pre-cooling. Int J Heat Mass Transfer 2007; 50:105 - 16; http://dx.doi.org/10.1016/j.ijheatmasstransfer.2006.06.030
- Goldberg A, Rubinsky B. The effect of electroporation type pulsed electric fields on DNA in aqueous solution. Technol Cancer Res Treat 2010; 9:423 - 30; PMID: 20626208
- Gothelf A, Eriksen J, Hojman P, Gehl J. Duration and level of transgene expression after gene electrotransfer to skin in mice. Gene Ther 2010; 17:839 - 45; http://dx.doi.org/10.1038/gt.2010.35; PMID: 20376097
- Dupuis M, Denis-Mize K, Woo C, Goldbeck C, Selby MJ, Chen M, et al. Distribution of DNA vaccines determines their immunogenicity after intramuscular injection in mice. J Immunol 2000; 165:2850 - 8; PMID: 10946318
- Johnson PA, Conway MA, Daly J, Nicolson C, Robertson J, Mills KH. Plasmid DNA encoding influenza virus haemagglutinin induces Th1 cells and protection against respiratory infection despite its limited ability to generate antibody responses. J Gen Virol 2000; 81:1737 - 45; PMID: 10859379
- Miller M, Rekas G, Dayball K, Wan YH, Bramson J. The efficacy of electroporated plasmid vaccines correlates with long-term antigen production in vivo. Vaccine 2004; 22:2517 - 23; http://dx.doi.org/10.1016/j.vaccine.2004.01.019; PMID: 15193376
- Hallengärd D, Bråve A, Isaguliants M, Blomberg P, Enger J, Stout R, et al. A combination of intradermal jet-injection and electroporation overcomes in vivo dose restriction of DNA vaccines. Genet Vaccines Ther 2012; 10:5; http://dx.doi.org/10.1186/1479-0556-10-5; PMID: 22873174
- Roos AK, Eriksson F, Walters DC, Pisa P, King AD. Optimization of skin electroporation in mice to increase tolerability of DNA vaccine delivery to patients. Mol Ther 2009; 17:1637 - 42; http://dx.doi.org/10.1038/mt.2009.120; PMID: 19532140
- Hallengärd D, Haller BK, Maltais A-K, Gelius E, Nihlmark K, Wahren B, et al. Comparison of plasmid vaccine immunization schedules using intradermal in vivo electroporation. Clin Vaccine Immunol 2011; 18:1577 - 81; http://dx.doi.org/10.1128/CVI.05045-11; PMID: 21752954