Abstract
Insulin is secreted from the pancreatic β-cells in response to elevated glucose. In intact islets the capacity for insulin release is determined by a complex interplay between different cell types. This has made it difficult to specifically assess the role of β-cell defects to the insulin secretory impairment in type 2 diabetes. Here we describe a new approach, based on optogenetics, that enables specific investigation of β-cells in intact islets.
We used transgenic mice expressing the light-sensitive cation channel Channelrhodopsin-2 (ChR2) under control of the insulin promoter. Glucose tolerance in vivo was assessed using intraperitoneal glucose tolerance tests, and glucose-induced insulin release was measured from static batch incubations. ChR2 localization was determined by fluorescence confocal microscopy. The effect of ChR2 stimulation with blue LED light was assessed using Ca2+ imaging and static islet incubations.
Light stimulation of islets from transgenic ChR2 mice triggered prompt increases in intracellular Ca2+. Moreover, light stimulation enhanced insulin secretion in batch-incubated islets at low and intermediate but not at high glucose concentrations. Glucagon release was not affected. Beta-cells from mice rendered diabetic on a high-fat diet exhibited a 3.5-fold increase in light-induced Ca2+ influx compared with mice on a control diet. Furthermore, light enhanced insulin release also at high glucose in these mice, suggesting that high-fat feeding leads to a compensatory potentiation of the Ca2+ response in β-cells.
The results demonstrate the usefulness and versatility of optogenetics for studying mechanisms of perturbed hormone secretion in diabetes with high time-resolution and cell-specificity.
Introduction
Defective insulin secretion is central to the pathogenesis of type 2 diabetes.Citation1,Citation2 Insulin release from the pancreatic β-cells is triggered by glucose metabolism, resulting in membrane depolarization, opening of voltage-dependent Ca2+-channels and in turn Ca2+-dependent exocytosis.Citation3 In addition, insulin release is influenced by other islet cell types, including α- and delta-cells, through a number of paracrine mechanisms.Citation4,Citation5 This complex interplay between the different islet cell types has made it difficult to delineate the role of β-cell defects to the overall insulin secretory impairment in type 2 diabetes. Beta-cell exocytosis can be measured using the patch-clamp technique. This method has been used extensively in single-cell preparations; however, the approach has a number of limitations, including the disrupted islet architecture induced by the cell dispersion.Citation6,Citation7 The patch-clamp technique has also been used to measure exocytosis from single β-cells within intact islets. This preserves the islet architecture, but the experiments are technically demanding and do not assess the overall secretory capacity of the islet.Citation7
New tools to specifically study β-cell function in intact islets are therefore needed. Here we describe a new alternative method based on ‘optogenetics’. The light-sensitive algal protein Channelrhodopsin-2 (ChR2) has been widely used to enable temporally precise stimulation of neurons.Citation8 ChR2 opens in response to blue light, which leads to cell depolarization.Citation9 Since β-cells are electrically excitable, we hypothesized that an experimental model in which ChR2 is expressed under the insulin promoter could be used to study β-cell function in intact islets. We crossed transgenic mice expressing a Cre-dependent ChR2Citation10 with Rip-Cre miceCitation11 and set up a system in which Ca2+-influx and insulin secretion in intact islets could be precisely and robustly controlled by light. This represents a new and versatile tool that is easy to use and may open up exciting possibilities to specifically study the β-cell population of intact islets under normal and diabetic conditions.
Results
We first assessed glucose tolerance and insulin secretion in transgenic (TG) ChR2+/−Rip-Cre+/− mice. Glucose tolerance (analyzed as AUC) was similar in TG and control mice () and there were no differences in insulin secretion from batch-incubated islets (). ChR2 is produced as a YFP-coupled fusion protein. Islets from TG animals exhibited a strong YFP signal, mainly confined to cell membranes (). Islets from control animals had no detectable YFP (, inset). We next dispersed TG islets into single cells and stained for insulin and glucagon. Of the insulin-expressing cells, 91.6 ± 2.7% were YFP-positive (). No glucagon-positive cells produced YFP. Fluorescence-activated cell sorting (FACS) yielded comparable results with 95.6 ± 1.5% YFP-positive β-cells and merely 2.7 ± 0.7% YFP-negative β-cells (; ). The number of YFP-positive non-β-cells was negligible (0.5 ± 0.1%), confirming that ChR2 was restricted to β-cells.
Figure 1. (A) IPGTTs of transgenic (TG, dotted trace; n = 7) and control mice (Ctrl, continuous trace; n = 8) show no significant difference. (B) Insulin secretion from batches of TG or control islets in response to 1 h incubations at 2.8 mmol/L (empty bars; n = 3 experiments) or 16.7 mmol/L glucose (gray bars; n = 3). (C) ChR2-YFP fluorescence in TG islets. Right panel shows an islet at higher magnification and a non-TG (Ctrl) islet stained with DAPI (inset). (D) Representative examples of ChR2-YFP fluorescence in dispersed islets cells stained for insulin (upper panel) or glucagon (lower panel). Merged images are shown to the right. Scale bar is 100 μm. Data are means ± SEM *P < 0.05. (E) Contour plot of fluorescent cell counting using FACS (representative example of β-cell fraction from one animal) with the PE-A signal representing insulin-positive (Ins+) and the FITC-A signal denoting YFP-positive cells (YFP+), all in the β-cell gate.
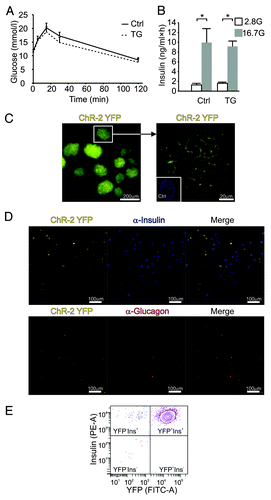
Table 1. FACS results of dispersed islet cells of 4 transgenic animals.
We next investigated whether activation of ChR2 had any functional effects in β-cells. ChR2 is non-selectively permeable for cations including Ca2+ 9. Since raising the intracellular Ca2+-concentration [Ca2+]i is critical for triggering insulin secretion,Citation15 we examined the effect of light stimulation on [Ca2+]i using Fura-2. Islets were stimulated with blue light corresponding to the maximum activation wavelength of ChR2 (~460 nm),Citation9 applied as a train of ten 100 ms pulses. Each train of light pulses evoked a distinct spike of [Ca2+]i, (). There was no effect of light stimulation on [Ca2+]i in non-ChR2 expressing control islets and no significant difference in basal Ca2+ levels between TG mice and control islets.
Figure 2. (A) Effect of blue LED light illumination on [Ca2+]i in ChR2-expressing transgenic TG islets (n = 11) or ChR-2 negative control islets (control; n = 7) (representative traces shown). Here, each light pulse is 100 ms long (depicted as blue dots, not to scale) and glucose concentration was 2.8 mmol/l. Ten images were acquired in between light pulses. (B) As in (A) but traces show recordings after 15 min pre-incubation with 5 µmol/l isradipine and 100 nmol/l SNX-482 (gray trace; n = 10) compared with untreated TG islets (black trace; n = 11, same trace as in [A]). (C) Changes in [Ca2+]i in response to different light protocols (for details see also Fig. S1). Each blue striped box corresponds to a 1 s stimulation segment during which ten 1 ms (light-colored traces) or ten 10 ms long (dark-colored traces) light pulses were fired at different frequencies: 10 Hz (blue traces; n = 3/8 experiments using 1 ms/10 ms pulses, respectively), 20 Hz (red traces; n = 4/4) or 40 Hz (green traces; n = 4/3). Ten images were acquired in between the 1 s long stimulation segments. (D) As in (c) but using continuous light for 1 s (n = 4) compared with pulsatile stimulation at 40 Hz and 10 ms pulse duration (n = 3). (E) Effect of longer pulsatile illumination on [Ca2+]i. Each thin blue stripe of the stimulation segment corresponds to a 500 ms light pulse with stimulation segment duration as despicted (I: 60, II: 30, and III: 10 s respectively). Images were acquired in between the single light pulses and between the stimulation segments. Representative examples of illumination-evoked currents with 500 ms (F) and 100 ms blue light pulses in single transgenic β-cells. (H) As in (G) but in ChR2-negative control islet cells.
![Figure 2. (A) Effect of blue LED light illumination on [Ca2+]i in ChR2-expressing transgenic TG islets (n = 11) or ChR-2 negative control islets (control; n = 7) (representative traces shown). Here, each light pulse is 100 ms long (depicted as blue dots, not to scale) and glucose concentration was 2.8 mmol/l. Ten images were acquired in between light pulses. (B) As in (A) but traces show recordings after 15 min pre-incubation with 5 µmol/l isradipine and 100 nmol/l SNX-482 (gray trace; n = 10) compared with untreated TG islets (black trace; n = 11, same trace as in [A]). (C) Changes in [Ca2+]i in response to different light protocols (for details see also Fig. S1). Each blue striped box corresponds to a 1 s stimulation segment during which ten 1 ms (light-colored traces) or ten 10 ms long (dark-colored traces) light pulses were fired at different frequencies: 10 Hz (blue traces; n = 3/8 experiments using 1 ms/10 ms pulses, respectively), 20 Hz (red traces; n = 4/4) or 40 Hz (green traces; n = 4/3). Ten images were acquired in between the 1 s long stimulation segments. (D) As in (c) but using continuous light for 1 s (n = 4) compared with pulsatile stimulation at 40 Hz and 10 ms pulse duration (n = 3). (E) Effect of longer pulsatile illumination on [Ca2+]i. Each thin blue stripe of the stimulation segment corresponds to a 500 ms light pulse with stimulation segment duration as despicted (I: 60, II: 30, and III: 10 s respectively). Images were acquired in between the single light pulses and between the stimulation segments. Representative examples of illumination-evoked currents with 500 ms (F) and 100 ms blue light pulses in single transgenic β-cells. (H) As in (G) but in ChR2-negative control islet cells.](/cms/asset/723eeabb-6f01-4681-b5c4-f77546a207c8/kisl_a_10928095_f0002.gif)
It is well established from neuronal studies that opening of ChR2 leads to membrane cation influx, depolarization and increases in [Ca2+]i.Citation9,Citation16 We therefore wanted to examine whether the light-induced increase in [Ca2+]i in β-cells represented a non-selective Ca2+-influx through ChR2 or involved downstream activation of voltage-gated Ca2+-channels. We treated TG islets with isradipine (5 µmol/l) and SNX-482 (100 nmol/l) to block L-type and R-type Ca2+-channels, respectively, which are the functionally most important Ca2+-channels in mouse β-cells.Citation17,Citation18 After 15 min incubation in the presence of the blockers, we observed significantly reduced [Ca2+]i spikes in response to light (, P < 0.001). In non-treated islets, each light pulse increased [Ca2+]i on average by 19.4 ± 0.8 nmol/l compared with 6.7 ± 1 nmol/l in blocker-treated islets (P < 0.001). This suggests that the light-evoked increase in [Ca2+]i is triggered by ChR2, but mainly involves activation of voltage-gated L-type and R-type Ca2+-channels.
We applied a number of stimulation protocols to examine the effect of different durations and frequencies of light pulses (all during 1 s) on Ca2+-influx in TG islets (details in the Methods section and in Fig. S1). When the stimulation frequency was held constant at 10 Hz, extending the pulse duration from 1 to 10 ms caused elevations in [Ca2+]i, (, P < 0.05). No significant effect of a change in pulse duration was detectable at 20 or 40 Hz. However, increasing the frequency from 10 to 20 or 40 Hz, while keeping the duration constant at either 1 or 10 ms, produced stronger increases in [Ca2+]i (P < 0.05 for all). The Ca2+ concentration returned to basal levels within 5 min after the light stimulation (not shown).
We also compared the most effective stimulation protocol (10 ms pulses at 40 Hz) with a more simple light illumination protocol (a single 1 s long pulse during each stimulus) and found no significant difference in light-evoked increases in [Ca2+]i between the 2 conditions (). To assess the effect of longer stimulations, we employed a pulsatile protocol with 500 ms light stimulations interspersed by 500 ms intervals without light. We used 3 different stimulation protocols: (I) 2×1 min pulsatile stimulation separated by 1 min without stimulation, (II) 3×30 s pulsatile stimulation separated by 30 s dark intervals, and (III) 4×10 s pulsatile stimulation separated by 10 s intervals (). The Ca2+ concentration increased rapidly in response to protocols (I) and (II) but declined during the stimulation period, suggestive of channel inactivation. The [Ca2+]i was reduced to near-basal values after the stimulus was removed. For both (I) and (II), the [Ca2+]i increase in response to the first stimulation period was more pronounced. By contrast, this pattern was not seen with protocol (III), having shorter stimulations, for which we rather observed a plateau between the stimulation periods.
Next, we investigated the effect of blue LED light on ion influx by measuring whole-cell Ca2+ currents in dispersed islet cells with the patch-clamp technique. In ChR2-expressing β-cells (YFP-positive) that were voltage-clamped at -70 mV, 500 ms light pulses evoked a rapid current of 19.1 ± 1.2 pA (). A current of 18.6 ± 2.1 pA was elicited in response to 100 ms pulses (). No light-evoked currents were observed in YFP-negative cells from transgenic animals (non-β-cells) and β-cells from control animals ().
We next assessed whether light could trigger insulin release from TG islets. As constant illumination evoked the same increase of [Ca2+]i compared with pulsatile light (), we decided to examine the feasibility of using 1 h constant light illumination to stimulate insulin secretion, as that would enable a considerably simpler and more broadly applicable experimental setup. Thus, a blue LED light was attached to tubes with TG islets (see Methods). Interestingly, light stimulation increased insulin secretion at 2.8 mmol/l glucose 2-fold (from 2.8 ± 0.4 to 5.3 ± 1.1 ng/ml, P < 0.05; ). This effect was not observed in non-ChR2 expressing control islets. In agreement with the Ca2+ measurements, blue light did not stimulate insulin release in the presence of L- and R-type Ca2+-channel blockers (). We found a suppressive effect of the VGCC blockers at 2.8 mmol/l glucose in both TG (3.9 ± 0.4 without blockers vs. 2.5 ± 0.1 ng/ml×h with blockers; P < 0.01, ) and control islets (3.5 ± 0.3 without vs. 2.6 ± 0.2 ng/ml×h with blockers; P < 0.05; Fig. S2). The fold-stimulation of insulin secretion by light was reduced in the presence of blockers (1.3 ± 0.1-fold vs. 1.9 ± 0.2-fold; P < 0.05). We observed no effect of light stimulation on glucagon release ().
Figure 3. (A) Insulin release in response to 1 h constant blue LED light stimulation (LED) in batch-incubated TG islets (n = 4 replicates) or non-ChR2 expressing control islets (n = 4) at 2.8 mmol/l glucose. (B) Insulin secretion from TG islets at 2.8 mmol/l glucose with or without 1 h constant light stimulation and in the presence or absence of 5 µmol/l isradipine and 100 nmol/l SNX-482 (n = 8). (C) Effect of light stimulation on glucagon release in TG (n = 6) and control islets (n = 6) at 2.8 mmol/l glucose. (D) Insulin secretion with or without 1 h light stimulation at 1 mmol/l (empty bars), 5.6 mmol/l (gray bars) and 16.7 mmol/l glucose (black; n = 7 for all). (E) Effect of different glucose concentrations on basal and light-induced [Ca2+]i concentrations subsequently recorded from the same islets with 5 min incubations in the respective concentration (black continuous trace 1 mmol/l; gray trace 2.8 mmol/l; 5.6 mmol/l in green and 16.7 mmol/l in blue; n = 5 experiments). (F) Same as in (E), but data depicted as changes in [Ca2+]i (Δ[Ca2+]i) to facilitate comparison of the peaks. Data in bar charts are means ± SEM *P < 0.05.
![Figure 3. (A) Insulin release in response to 1 h constant blue LED light stimulation (LED) in batch-incubated TG islets (n = 4 replicates) or non-ChR2 expressing control islets (n = 4) at 2.8 mmol/l glucose. (B) Insulin secretion from TG islets at 2.8 mmol/l glucose with or without 1 h constant light stimulation and in the presence or absence of 5 µmol/l isradipine and 100 nmol/l SNX-482 (n = 8). (C) Effect of light stimulation on glucagon release in TG (n = 6) and control islets (n = 6) at 2.8 mmol/l glucose. (D) Insulin secretion with or without 1 h light stimulation at 1 mmol/l (empty bars), 5.6 mmol/l (gray bars) and 16.7 mmol/l glucose (black; n = 7 for all). (E) Effect of different glucose concentrations on basal and light-induced [Ca2+]i concentrations subsequently recorded from the same islets with 5 min incubations in the respective concentration (black continuous trace 1 mmol/l; gray trace 2.8 mmol/l; 5.6 mmol/l in green and 16.7 mmol/l in blue; n = 5 experiments). (F) Same as in (E), but data depicted as changes in [Ca2+]i (Δ[Ca2+]i) to facilitate comparison of the peaks. Data in bar charts are means ± SEM *P < 0.05.](/cms/asset/bff83f8b-d4e0-4137-b371-92dd1d27b54b/kisl_a_10928095_f0003.gif)
We also investigated whether light-stimulated insulin release was dependent on the extracellular glucose concentration. Islets were incubated at 1, 5.6 or 16.7 mmol/l glucose. Light evoked a 2-fold increase of insulin secretion at 1 mmol/l glucose (from 0.9 ± 0.2 to 1.8 ± 0.2 ng/ml, P < 0.01; ) and a 3-fold elevation at 5.6 mmol/l (from 1.0 ± 0.2 to 2.7 ± 0.4 ng/ml; P < 0.01; no significant difference in insulin response between the 2 glucose concentrations). By contrast, at 16.7 mmol/l glucose, the application of light did not further potentiate secretion. Glucose-induced insulin secretion of control islets was unaffected by blue light illumination (not shown).
To investigate whether the glucose-dependent effect of light on insulin secretion was paralleled by similar changes in Ca2+ levels, we performed Ca2+ imaging experiments at different glucose concentrations. Blue LED light increased [Ca2+]i more effectively at 1, 2.8 and 5.6 mmol/glucose compared with 16.7 mmol/l (). The peak increase in [Ca2+]i in response to a light pulse was on average 13% at 1, 2.8 and 5.6 mmol/glucose. The responses to the first pulses were more pronounced (17.9% ± 1% at pulse 1 vs. 10.2% ± 0.2% at pulse 10). The peak elevation of [Ca2+]i was only 7.5 ± 0.7% at 16.7 mmol/l glucose, which is significantly smaller compared with the other 3 glucose concentrations (P < 0.01 for all 3 comparisons). It should be noted that Fura-2 is also loaded into non-β-cells, and particularly the basal values at 1 mmol/l glucose may therefore overestimate the [Ca2+]i in the β-cell population.
Stimulation with high KCl (70 mmol/l added to buffer with 1 mmol/l glucose) elicited a stronger secretory response than any of the previous protocols. This is an expected consequence of all islet cells being simultaneously depolarized (Fig. S3). Light had no effect on [Ca2+]i when high KCl was present (Fig. S3).
To investigate the TG islets in a diabetic context, a subset of the mice was given a high fat diet (HFD) for 8 wk. Mice on HFD were glucose-intolerant compared with animals on a standard chow diet (SCD; P < 0.01; ,), and islets from HFD mice had a 2-fold higher insulin content (P < 0.001; ). Interestingly, the light-evoked increase of [Ca2+]i was substantially higher in HFD islets compared with SCD (). During the first light-induced peak, [Ca2+]i increased by 34 ± 4 nmol/l in SCD islets and by 117 ± 3 nmol/l in HFD islets (P < 0.001), which amounts to a 3.5-fold difference. Moreover, the plateau level of [Ca2+]i after 10 trains of stimulation was significantly higher in HFD islets (360.2 ± 1.3 in HFD vs. 329.8 ± 0.8 in SCD; P < 0.001).
Figure 4. (A) Intraperitoneal glucose tolerance tests in TG (dotted lines) and control animals (solid lines) given a high fat diet for 8 wk (HFD, gray traces; n = 6 TG and 8 control mice) or standard chow diet (SCD, black traces; n = 7 TG and 8 control mice). Differences were assessed by analyzing the AUC. (B) Insulin content in islets from TG or control mice on HFD (gray bars) or SCD (white bars; n = 5 in each group). (C) Representative recordings of Fura-2 fluorescence in response to light stimulation in islets from HFD (gray trace) and SCD mice (black line) at 2.8 mmol/L glucose (n = 4). (D) Insulin secretion from HFD islets with or without 1 h constant light stimulation at 1 mmol/l (empty bars), 5.6 mmol/l (gray) and 16.7 mmol/l glucose (black; n = 5). (E) Insulin release in islets from mice on high-fat diet (n = 3) stimulated with blue LED light (gray bars, +LED) and without LED (white bars; -LED) as well as in the presence (+) or absence (-) of SNX482 (100 nmol/l), isradipine (5 µmol/l). (F) YFP fluorescence intensities in islets from SCD and HFD mice (n = 4). Data are means ± SEM *P < 0.05.
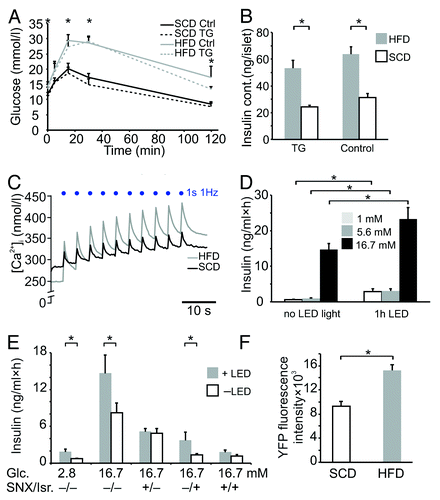
Finally, we measured light-stimulated insulin secretion in islets from the HFD mice. There was a 5-fold light-induced increase in insulin release at 1 mmol/l glucose (0.6 ± 0.1 vs. 2.9 ± 0.7 ng/ml; P < 0.01; ) and a 3-fold elevation at 5.6 mmol/l glucose (1.0 ± 0.1 vs. 3.1 ± 0.6 ng/ml; P < 0.01). Notably, in contrast to islets from SCD mice (), light illumination of HFD islets significantly potentiated insulin release also at high glucose (14.7 ± 1.7 without light and 23.3 ± 3.3 ng/ml with light; , P < 0.05). There was a significant difference between the light-induced fold increases of SCD and HFD at 16.7 mmol/l (1.1 ± 0.1 in SCD vs. 1.7 ± 0.2 in HFD; P < 0.05). To assess which type of voltage-gated Ca2+ channel was responsible for this effect, we also conducted the experiments in the presence of the L-type Ca2+ channel blocker isradipine and the R-type blocker SNX482. Isradipine potently inhibited glucose-induced insulin secretion, but insulin release was still enhanced by light illumination (1.4 ± 0.2 ng/ml without blue LED light and 3.7 ± 1.3 ng/ml with light, P < 0.05, ). By contrast, after incubation with SNX482 the stimulatory effect of light was abolished (4.9 ± 0.8 without light and 5.1 ± 0.6 with light; P > 0.05). This was also observed when both blockers were added (1.2 ± 0.3 without light and 1.8 ± 0.3 with light; P > 0.05). Using confocal fluorescence microscopy, we found that the mean YFP signal in islets from ChR2-positive HFD mice increased by 67 ± 14% compared with islets from SCD mice, suggestive of increased amounts of the ChR2-YFP fusion protein (P < 0.01, ).
Discussion
This study describes a new experimental model for studying β-cell function in intact islets using light. We demonstrate high sensitivity and temporal precision of the stimulations (within ms) in a manner that has previously not been feasible in intact islets. The transgenic animals had similar blood glucose levels in vivo and insulin release in vitro compared with non-transgenic littermates (). Although we cannot exclude subtle metabolic differences, the data suggest that the transgenic mice have no major metabolic disturbances.
ChR2 presence is detectable only in β-cells, which demonstrates the high specificity of our approach.
It is well established from studies in neurons that opening of ChR2 results in membrane depolarization and increased [Ca2+]i.Citation9,Citation16 In islets from mice on standard chow diet, the [Ca2+]i response to light was largely abolished when L- and R-type Ca2+-channels were blocked (), suggesting the main light-dependent Ca2+ influx to occur through L- and R-type with only small Ca2+ entry through ChR2 or other Ca2+-channels. Of note, ChR2 conductance and contribution to changes in membrane potential are small.Citation19 Consequently, stimulation of Ca2+ influx after blocking L- and R-type channels had only a small effect on insulin release. This suggests that insulin secretion in the transgenic animals is mainly exerted through activation of voltage-activated L- and R-type Ca2+-channels in support of previous studiesCitation17,Citation18.
The results demonstrate that light stimulation triggers insulin secretion distal to glucose metabolism by increasing Ca2+-influx. Accordingly, the light illumination stimulated insulin release at low glucose but had no effect at high glucose, when the secretory machinery is already highly stimulated. This was paralleled by an attenuated light-induced increase in [Ca2+]i at high glucose compared with low glucose ().
We could not detect any effect on glucagon releaseCitation20,Citation21 by the light stimulations (), which may indicate that the quantity of insulin that is released in response to the light pulses is below the threshold for paracrine suppression of α-cell activity.
Early stages of type 2 diabetes are characterized by hyperinsulinemia,Citation22 which is often followed by a gradual deterioration of secretory capacity. Moreover, mice on HFD exhibit enhanced insulin secretion.Citation23 It has, however, been difficult to specifically investigate the effect of HFD on β-cells in intact islets, since other islet cell types may potentially also be influenced by the diabetic condition and affect the overall insulin release.
By using the optogenetic model we were able to specifically stimulate β-cells while keeping extracellular glucose constant. Interestingly, the light-induced increase in [Ca2+]i was considerably enhanced in HFD islets (). Furthermore, in contrast to SCD islets, insulin release was stimulated by light even at high glucose (). The 67% higher YFP signal, suggestive of higher ChR2 expression in HFD islets, does not fully explain the 3.5-fold increase in light-induced Ca2+ influx. As ChR2 Ca2+ conductance is smallCitation19 (), it rather suggests that there is a compensatory potentiation of the Ca2+-response by voltage-gated Ca2+-channels in β-cells from HFD mice. In fact, previous studies have shown that mice responded to HFD with a sensitization of the Ca2+-response in β-cells.Citation24,Citation25 Furthermore, fatty acids have been shown to enhance [Ca2+]iCitation26 and exocytosis in β-cells by Ca2+-channel activation and expansion of the readily-releasable pool of insulin granules.Citation27 Interestingly, we found the light-induced response observed at high glucose in HFD islets was due to activation of R-type Ca2+ channels (). Although inhibition of L-type channels also strongly reduced insulin release under the same conditions, light could still enhance secretion.
It is tempting to speculate that an exaggerated Ca2+-response mediated by R-type channels could contribute to the initial hyperinsulinemia seen in T2D but may subsequently lead to Ca2+-toxicity and deterioration of the β-cell population.
In summary, we present an experimental system that may be used to address a wide range of pathophysiological questions and to evaluate the effect of pharmacological compounds on β-cell secretory capacity. The method specifically assesses Ca2+-influx and insulin release. These are critical steps in the glucose stimulus-secretion coupling pathway and have been implicated in the pathophysiology of T2D.Citation28 The versatility of the technique opens up new possibilities to measure Ca2+-influx, Ca2+-sensitivity, exocytotic capacity as well as the triggering pathways of insulin secretion using precise stimulation protocols. A major advantage over methods such as the patch-clamp technique is the possibility to stimulate and measure insulin secretion from several β-cells and also multiple islets simultaneously. The experimental setup should also be considerably easier to use and may be applied in medium- or high-throughput settings for testing of drug effects on β-cell secretory capacity. Optogenetics has been instrumental to study neurological disorders, and the optogenetics toolbox is extensive and continuously growing.Citation10,Citation29 In addition to the model described here, transgenic mice expressing ChR2 under the glucagon promoter (in α-cells) or in vitro systems with viral delivery of optogenetic constructs may prove useful to better understand islet function and pathology.
Methods
Animals
Channelrhodopsin-2(H134R)-YFP mice (Jackson laboratories #012569Citation10) were bred with hemizygous Rip-Cre miceCitation11 to obtain ChR2+/−Rip-Cre+/−, denoted transgenics [TG]. Presence of Cre, restricted to β-cells, excises a STOP cassette upstream of ChR2-YFP, whose expression is then driven by the CAG promoter. ChR2+/+Rip-Cre+/− animals were not used as they had lower birth weight and produced smaller litters. Non-ChR2-expressing littermates were used as controls. Islet preparations and intraperitoneal glucose tolerance tests were performed as in.Citation11 The high fat and standard chow diet contained 6 0kcal% and 10 kcal% fat, respectively (Research Diets). Genotyping for Cre was performed as inCitation11 and for ChR2 using the standard protocol from Jackson laboratories. All animal experiments were approved by the local ethics committee. Intraperitoneal glucose tolerance tests (IPGTT) with blood glucose measured at 0, 5, 15, 30 and 120 min after glucose injection. Area under the curve (AUC) for comparing glucose tolerance was calculated according to.Citation2
Hormone release assays
Islet hormone release was assessed in static batch incubations with 12 islets in tubes containing 500 µl Krebs-Ringer bicarbonate (KRB) buffer. Islets were pre-incubated for 30 min at 2.8 mmol/l glucose, followed by 60 min incubation with glucose as indicated +/− light. Insulin was determined by RIA (Millipore AB) and glucagon by an electrochemiluminescence assay (Mesoscale).
Imaging
Islets were placed on glass-bottom dishes coated with Cell-Tak (BD Biosciences) and incubated in RPMI1640 supplemented with 5 mmol/l glucose and 10% FBS for 24 h. They were then perfused with an imaging buffer as used inCitation1 containing 2.8 mmol/l glucose (1 mmol/l for the imaging experiments in ), and Fura-2AM imaging was performed as previously described.Citation2 Images were acquired between the light pulses at a rate of > 2/s for each 340 nm and 380 nm excitation with emission being captured at 510 nm. Ratios and peaks were analyzed using in-house scripts. Absolute Ca2+ concentrations were calculated after calibration with a Fura-2 Ca2+ imaging calibration kit (Molecular Probes) using the formula in reference Citation12 YFP fluorescence intensity was quantified as described in reference Citation13 after islets being fixed in 4% PFA for 30 min, washed 3 times with PBS and directly mounted. The system was tested using fluorescent beads in different concentrations to ensure we work well below saturation limits and that our YFP signals were within a linear range where differences in fluorescence signal reflect changes in YFP protein.
Light stimulations
To record light-evoked [Ca2+]i changes, we used a fiber-coupled blue LED (LE-1B-C/CE, WT&T) with an attached miniature collimator (011-TU2, WT&T). The LED was triggered by ER-BOB100 and the imaging software iQ2 (Andor Technology). Islets were stimulated at a ~45° angle which at 3.5 cm distance delivered ~0.5 mW/mm2, as measured with a digital power meter (PM100D; Thorlabs Sweden AB). The intensity of laboratory room light was ~100 µW at 460 nm, which is negligible compared with that in the stimulation protocols. Nevertheless, light stimulations were performed in the dark to avoid any possible light contamination. Light stimulation was applied as trains of ten 1 s stimulation segments. Ten images were acquired in the interval between 2 segments. Each 1 s segment consisted of several light pulses, depending on the frequency used (see Fig. S1 for details). For the static batch incubations, 5 mm blue LEDs (Cree LC503FBL1-15P-A3-00001, Premier Farnell) were attached to the inside of the lids of 4 ml polystyrene tubes and connected to a standard power source. Light intensity was ~0.1 mW/mm2. For light stimulation during patch-clamp recordings, we used the same light source as for Ca2+ imaging but with a custom-made collimator and narrowing bandwidth filter (F230FC-custom and FB460-10-12.5-SP, respectively; both from Thorlabs).
Electrophysiology
Measurement of blue LED light-induced currents was performed as in reference Citation14 on a HEKA EPC9 amplifier (HEKA; Lambrecht-Pfalz) with an extracellular solution containing 138 mM NaCl, 5.6 mM KCl, 2.6 mM CaCl2, 1.2 mM MgCl2, 5 mM HEPES, and 5 mM glucose (pH 7.4 with NaOH) and an intracellular solution consisting of 125 mM Cs-glutamate, 10 mM CsCl, 10 mM NaCl, 1 mM MgCl2, 5 mM HEPES, 3 mM Mg-ATP, 0.1 mM cAMP, and 0.05 mM EGTA. Cells were clamped at -70 mV while light pulses of either 500 ms or 5 x 100 ms at 13 µW/mm2 were elicited, triggered by the acquisition software. ChR2-positive cells were identified by the presence of YFP fluorescence.
Fluorescent cell counting
Mouse islets were dispersed in 0.5 ml Stem Pro Accutase (Gibco, Life Technologies Europe) for 5 min at 37 °C, while shaken at 300 rpm, then vortexed for 10 s and rested for 5 min at RT in 5 ml RPMI1640 with 5% FBS. After filtering through a 100 µm cell strainer and centrifugation at 10 °C and 800 g, cells were resuspended in 100 µl RPMI, fixed/permeabilized using a Perfix-nc Kit according to the manufacturers recommendations (Beckman Coulter) and finally stained against insulin with a phycoerythrin (PE) conjugated anti-insulin antibody at 1:100 (Cat.no. 8508, Cell Signaling Technology) for 1 h. Cells were then washed twice and after resuspension in wash buffer counted on a BD FacsAria (BD Biosciences) using the fluorescein (FITC) and PE channels.
Statistical evaluation
Numbers (n) denote experiments, and 2–3 mice of each genotype were used per experiment unless otherwise indicated. Statistical significance was evaluated using 2-tailed Student t tests.
Abbreviations: | ||
AUC | = | area under the curve |
CAG | = | cytomegalovirus enhancer/chicken beta-actin/rabbit beta-globin hybrid promoter |
ChR2 | = | channelrhodopsin-2(H134R) |
FACS | = | fluorescence-activated cell sorting |
FITC | = | fluorescein isothiocyanate |
HFD | = | high-fat diet |
PE | = | phycoerythrin |
IPGTT | = | intraperitoneal glucose tolerance test |
SCD | = | standard chow diet |
TG | = | transgenic (ChR2-YFP-positive) |
VGCC | = | voltage-gated calcium channel |
Additional material
Download Zip (314.8 KB)Disclosure of Potential Conflicts of Interest
No potential conflicts of interest were disclosed.
Acknowledgments
Supported by the NovoNordisk Foundation, the Magnus Bergvall Foundation, the Royal Physiographic Society, the Swedish Diabetes Association and the Swedish Research Council. We thank B.M. Nilsson, A.M. Ramsay and P.A. Bertilsson for technical assistance.
Author contributions
T.M.R. designed the study, performed the majority of the experiments and wrote the manuscript. F.S. and A.S.A. researched data and contributed to discussion. I.G.M. developed analysis software, analyzed data and discussed the manuscript. A.H.R. designed the study and wrote the paper.
References
- Rosengren AH, Jokubka R, Tojjar D, Granhall C, Hansson O, Li DQ, Nagaraj V, Reinbothe TM, Tuncel J, Eliasson L, et al. Overexpression of alpha2A-adrenergic receptors contributes to type 2 diabetes. Science 2010; 327:217 - 20
- Reinbothe TM, Alkayyali S, Ahlqvist E, Tuomi T, Isomaa B, Lyssenko V, Renström E. The human L-type calcium channel Cav1.3 regulates insulin release and polymorphisms in CACNA1D associate with type 2 diabetes. Diabetologia 2013; 56:340 - 9; http://dx.doi.org/10.1007/s00125-012-2758-z; PMID: 23229155
- Rorsman P, Braun M, Zhang Q. Regulation of calcium in pancreatic α- and β-cells in health and disease. Cell Calcium 2012; 51:300 - 8; http://dx.doi.org/10.1016/j.ceca.2011.11.006; PMID: 22177710
- Hauge-Evans AC, King AJ, Carmignac D, Richardson CC, Robinson IC, Low MJ, Christie MR, Persaud SJ, Jones PM. Somatostatin secreted by islet delta-cells fulfills multiple roles as a paracrine regulator of islet function. Diabetes 2009; 58:403 - 11; http://dx.doi.org/10.2337/db08-0792; PMID: 18984743
- Kieffer TJ, Heller RS, Unson CG, Weir GC, Habener JF. Distribution of glucagon receptors on hormone-specific endocrine cells of rat pancreatic islets. Endocrinology 1996; 137:5119 - 25; PMID: 8895386
- Jaques F, Jousset H, Tomas A, Prost AL, Wollheim CB, Irminger JC, Demaurex N, Halban PA. Dual effect of cell-cell contact disruption on cytosolic calcium and insulin secretion. Endocrinology 2008; 149:2494 - 505; http://dx.doi.org/10.1210/en.2007-0974; PMID: 18218692
- Göpel S, Zhang Q, Eliasson L, Ma XS, Galvanovskis J, Kanno T, Salehi A, Rorsman P. Capacitance measurements of exocytosis in mouse pancreatic alpha-, beta- and delta-cells within intact islets of Langerhans. J Physiol 2004; 556:711 - 26; http://dx.doi.org/10.1113/jphysiol.2003.059675; PMID: 14966302
- Deisseroth K. Optogenetics. Nat Methods 2011; 8:26 - 9; PMID: 21191368
- Nagel G, Szellas T, Huhn W, Kateriya S, Adeishvili N, Berthold P, Ollig D, Hegemann P, Bamberg E. Channelrhodopsin-2, a directly light-gated cation-selective membrane channel. Proc Natl Acad Sci U S A 2003; 100:13940 - 5; http://dx.doi.org/10.1073/pnas.1936192100; PMID: 14615590
- Madisen L, Mao T, Koch H, Zhuo JM, Berenyi A, Fujisawa S, Hsu YW, Garcia AJ 3rd, Gu X, Zanella S, et al. A toolbox of Cre-dependent optogenetic transgenic mice for light-induced activation and silencing. Nat Neurosci 2012; 15:793 - 802; http://dx.doi.org/10.1038/nn.3078; PMID: 22446880
- Kalis M, Bolmeson C, Esguerra JL, Gupta S, Edlund A, Tormo-Badia N, Speidel D, Holmberg D, Mayans S, Khoo NK, et al. Beta-cell specific deletion of Dicer1 leads to defective insulin secretion and diabetes mellitus. PLoS One 2011; 6:e29166; http://dx.doi.org/10.1371/journal.pone.0029166; PMID: 22216196
- Grynkiewicz G, Poenie M, Tsien RY. A new generation of Ca2+ indicators with greatly improved fluorescence properties. J Biol Chem 1985; 260:3440 - 50; PMID: 3838314
- Buda P, Reinbothe T, Nagaraj V, Mahdi T, Luan C, Tang Y, Axelsson AS, Li D, Rosengren AH, Renström E, et al. Eukaryotic translation initiation factor 3 subunit e controls intracellular calcium homeostasis by regulation of cav1.2 surface expression. PLoS One 2013; 8:e64462; http://dx.doi.org/10.1371/journal.pone.0064462; PMID: 23737983
- Reinbothe TM, Ivarsson R, Li DQ, Niazi O, Jing X, Zhang E, Stenson L, Bryborn U, Renstrom E. Glutaredoxin-1 mediates NADPH-dependent stimulation of calcium-dependent insulin secretion. Molecular endocrinology (Baltimore, Md 2009; 23:893-900.
- Wollheim CB, Sharp GW. Regulation of insulin release by calcium. Physiol Rev 1981; 61:914 - 73; PMID: 6117094
- Zhang F, Wang LP, Brauner M, Liewald JF, Kay K, Watzke N, Wood PG, Bamberg E, Nagel G, Gottschalk A, et al. Multimodal fast optical interrogation of neural circuitry. Nature 2007; 446:633 - 9; http://dx.doi.org/10.1038/nature05744; PMID: 17410168
- Jing X, Li DQ, Olofsson CS, Salehi A, Surve VV, Caballero J, Ivarsson R, Lundquist I, Pereverzev A, Schneider T, et al. CaV2.3 calcium channels control second-phase insulin release. J Clin Invest 2005; 115:146 - 54; http://dx.doi.org/10.1172/JCI200522518; PMID: 15630454
- Schulla V, Renström E, Feil R, Feil S, Franklin I, Gjinovci A, Jing XJ, Laux D, Lundquist I, Magnuson MA, et al. Impaired insulin secretion and glucose tolerance in beta cell-selective Ca(v)1.2 Ca2+ channel null mice. EMBO J 2003; 22:3844 - 54; http://dx.doi.org/10.1093/emboj/cdg389; PMID: 12881419
- Lin JY. A user’s guide to channelrhodopsin variants: features, limitations and future developments. Exp Physiol 2011; 96:19 - 25; http://dx.doi.org/10.1113/expphysiol.2009.051961; PMID: 20621963
- Franklin I, Gromada J, Gjinovci A, Theander S, Wollheim CB. Beta-cell secretory products activate alpha-cell ATP-dependent potassium channels to inhibit glucagon release. Diabetes 2005; 54:1808 - 15; http://dx.doi.org/10.2337/diabetes.54.6.1808; PMID: 15919803
- Olsen HL, Theander S, Bokvist K, Buschard K, Wollheim CB, Gromada J. Glucose stimulates glucagon release in single rat alpha-cells by mechanisms that mirror the stimulus-secretion coupling in beta-cells. Endocrinology 2005; 146:4861 - 70; http://dx.doi.org/10.1210/en.2005-0800; PMID: 16081632
- Jones CN, Pei D, Staris P, Polonsky KS, Chen YD, Reaven GM. Alterations in the glucose-stimulated insulin secretory dose-response curve and in insulin clearance in nondiabetic insulin-resistant individuals. J Clin Endocrinol Metab 1997; 82:1834 - 8; http://dx.doi.org/10.1210/jcem.82.6.3979; PMID: 9177392
- Reimer MK, Ahrén B. Altered beta-cell distribution of pdx-1 and GLUT-2 after a short-term challenge with a high-fat diet in C57BL/6J mice. Diabetes 2002; 51:Suppl 1 S138 - 43; http://dx.doi.org/10.2337/diabetes.51.2007.S138; PMID: 11815473
- Peyot ML, Pepin E, Lamontagne J, Latour MG, Zarrouki B, Lussier R, Pineda M, Jetton TL, Madiraju SR, Joly E, et al. Beta-cell failure in diet-induced obese mice stratified according to body weight gain: secretory dysfunction and altered islet lipid metabolism without steatosis or reduced beta-cell mass. Diabetes 2010; 59:2178 - 87; http://dx.doi.org/10.2337/db09-1452; PMID: 20547980
- Gonzalez A, Merino B, Marroquí L, Ñeco P, Alonso-Magdalena P, Caballero-Garrido E, Vieira E, Soriano S, Gomis R, Nadal A, et al. Insulin hypersecretion in islets from diet-induced hyperinsulinemic obese female mice is associated with several functional adaptations in individual β-cells. Endocrinology 2013; 154:3515 - 24; http://dx.doi.org/10.1210/en.2013-1424; PMID: 23867214
- Remizov O, Jakubov R, Düfer M, Krippeit Drews P, Drews G, Waring M, Brabant G, Wienbergen A, Rustenbeck I, Schöfl C. Palmitate-induced Ca2+-signaling in pancreatic beta-cells. Mol Cell Endocrinol 2003; 212:1 - 9; http://dx.doi.org/10.1016/j.mce.2003.09.026; PMID: 14654245
- Olofsson CS, Salehi A, Holm C, Rorsman P. Palmitate increases L-type Ca2+ currents and the size of the readily releasable granule pool in mouse pancreatic beta-cells. J Physiol 2004; 557:935 - 48; http://dx.doi.org/10.1113/jphysiol.2004.066258; PMID: 15090611
- Rosengren AH, Braun M, Mahdi T, Andersson SA, Travers ME, Shigeto M, Zhang E, Almgren P, Ladenvall C, Axelsson AS, et al. Reduced insulin exocytosis in human pancreatic β-cells with gene variants linked to type 2 diabetes. Diabetes 2012; 61:1726 - 33; http://dx.doi.org/10.2337/db11-1516; PMID: 22492527
- Zhang F, Gradinaru V, Adamantidis AR, Durand R, Airan RD, de Lecea L, Deisseroth K. Optogenetic interrogation of neural circuits: technology for probing mammalian brain structures. Nat Protoc 2010; 5:439 - 56; http://dx.doi.org/10.1038/nprot.2009.226; PMID: 20203662