Abstract
Autonomic cardiac neurons have a common origin in the neural crest but undergo distinct developmental differentiation as they mature toward their adult phenotype. Progenitor cells respond to repulsive cues during migration, followed by differentiation cues from paracrine sources that promote neurochemistry and differentiation. When autonomic axons start to innervate cardiac tissue, neurotrophic factors from vascular tissue are essential for maintenance of neurons before they reach their targets, upon which target-derived trophic factors take over final maturation, synaptic strength and postnatal survival. Although target-derived neurotrophins have a central role to play in development, alternative sources of neurotrophins may also modulate innervation. Both developing and adult sympathetic neurons express proNGF, and adult parasympathetic cardiac ganglion neurons also synthesize and release NGF. The physiological function of these “non-classical” cardiac sources of neurotrophins remains to be determined, especially in relation to autocrine/paracrine sustenance during development.
Cardiac autonomic nerves are closely spatially associated in cardiac plexuses, ganglia and pacemaker regions and so are sensitive to release of neurotransmitter, neuropeptides and trophic factors from adjacent nerves. As such, in many cardiac pathologies, it is an imbalance within the two arms of the autonomic system that is critical for disease progression. Although this crosstalk between sympathetic and parasympathetic nerves has been well established for adult nerves, it is unclear whether a degree of paracrine regulation occurs across the autonomic limbs during development. Aberrant nerve remodeling is a common occurrence in many adult cardiovascular pathologies, and the mechanisms regulating outgrowth or denervation are disparate. However, autonomic neurons display considerable plasticity in this regard with neurotrophins and inflammatory cytokines having a central regulatory function, including in possible neurotransmitter changes. Certainly, neurotrophins and cytokines regulate transcriptional factors in adult autonomic neurons that have vital differentiation roles in development. Particularly for parasympathetic cardiac ganglion neurons, additional examinations of developmental regulatory mechanisms will potentially aid in understanding attenuated parasympathetic function in a number of conditions, including heart failure.
Introduction
Sympathetic and parasympathetic branches of the cardiac autonomic nervous system (ANS) work in a reciprocal fashion to modulate heart rate (chronotropy) and conduction velocity (dromotropy) primarily through actions on cardiac pacemaker tissue. In addition, sympathetic nerves innervate atrial and ventricular cardiomyocytes and can thereby influence force of contraction (inotropy) and relaxation (lusitropy).Citation1-Citation8 Postganglionic cardiac sympathetic neurons have their cell bodies primarily in the paravertebral stellate (inferior-middle cervical) ganglion neurons (); 92% of retrogradely-labeled nerves from the heart have their origins in this ganglion.Citation9,Citation10 These sympathetic neurons primarily utilize norepinephrine as their principal neurotransmitter, although other neuropeptides, such as neuropeptide Y (NPY) and galanin, are also co-released from sympathetic terminals.Citation11,Citation12 Among other functions, NPY and galanin decrease acetylcholine release from adjacent parasympathetic terminals.Citation13-Citation16 Parasympathetic neurons receive pre-ganglionic inputs from the vagus and have their cell bodies within the cardiac ganglia (). The mammalian cardiac ganglia are arranged in discrete locations within the atrial epicardium closely associated with epicardial fat, in ganglionated plexi along the walls of the major cardiac vessels, and some are also present within the ventricular wall.Citation17-Citation22 The primary neurotransmitter in cardiac ganglion neurons is acetylcholine; however, like the sympathetic nerves, neuropeptides such as vasoactive intestinal polypeptide (VIP) may also be co-released from parasympathetic terminals, as is nitric oxide.Citation3,Citation23-Citation25 Parasympathetic attenuation of heart rate is effected primarily through hyperpolarization of nodal tissue, both sino-atrial and atrio-ventricular.
Figure 1. Sympathetic stellate ganglion; most cardiac-projecting neurons have their origins in this ganglion. Developmental profile with immunohistochemistry for the noradrenergic marker tyrosine hydroxylase (TH; green) in sheep. Sheep sympathetic neurons express TH by E100 with more prominent expression by E129; staining is localized in neuronal cytoplasm (white arrowheads) and within nerve bundles and fibers (gray arrowheads) at all ages examined (Jonker S., Louey S., Moses M., Macek A., Giraud G., Thornburg K., Hasan W.; unpublished data). Scale bar in C is 200 υm.
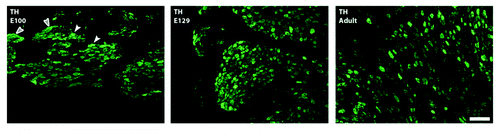
Figure 2. Parasympathetic cardiac ganglion. Immunohistochemical staining in an adult rat cardiac ganglion for cholinergic marker vesicular acetylcholine transporter (VAChT; red) and catecholaminergic marker tyrosine hydroxylase (TH; green). Neurons stain in the soma for VAChT (white arrowhead) and also receive input from cholinergic pre-ganglionic terminals (small varicosities around cell bodies). Small intensely fluorescent (SIF; gray arrows) cells are catecholaminergic and believed to function as inter-neurons; here SIF cells make contact with each other and with a cholinergic neuron (Moses M., Macek A. Hasan W.; unpublished data). Scale bar is 50 υm.
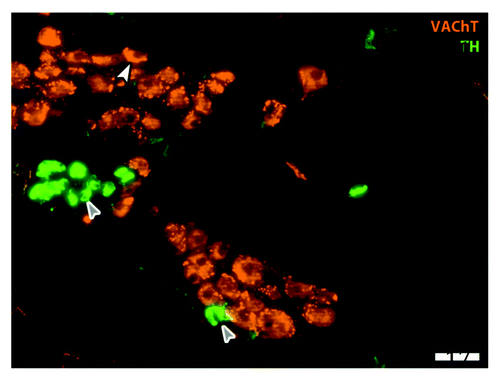
For heart rate control, the close physical proximity of postganglionic cardiac parasympathetic and sympathetic axons in pacemaker regions allows formation of axo-axonal synapses and reciprocal modulation of function, through either acetylcholine inhibition of norepinephrine release or vice versa.Citation3,Citation26-Citation31 Despite mutual modulation, vagal influences on the sinus node predominate over sympathetic effects; however, vagally induced bradycardia is greater in the presence of tonic sympathetic stimulation. Hence, the concept of accentuated antagonism has emerged to define the functional relationship between these systems.Citation3,Citation13,Citation28
An additional layer of complexity within peripheral autonomic limb interactions lies in the intrinsic cardiac nervous system. In addition to a simple relay function for conveying preganglionic vagal impulses, integration of parasympathetic, sensory and sympathetic inputs via local circuit neurons occurs within cardiac ganglia. This level of integration has been studied in detail and is critical for allowing the formation of rapid temporal reflexes that can enable local regulation of heart rate on a beat-to-beat basis.Citation32-Citation35 Key to this integration, aside from intraganglionic crosstalk, are interganglionic connections and descending inputs.Citation36,Citation37 Axo-somatic connections between sympathetic nerves and parasympathetic neurons also mediate prejunctional autonomic interactions within the cardiac gangliaCitation35; for the right atria these interactions involve neurons in the posterior atrial ganglionated plexus. In contrast, ablation of the right atrial ganglionated plexus attenuates vagal bradycardia while retaining vagal inhibition of sympathetic function.Citation35,Citation38 Indeed, Armour and colleagues have demonstrated that local circuit neurons, those that do not project their axons beyond the ganglion, constitute a majority of the neurons in the mammalian cardiac ganglion.Citation35,Citation39 Cardiac ganglia therefore represent an important site for investigations into peripheral autonomic interactions.
The development of cardiac autonomic innervation has four distinct phases; neural crest cell (NCC) migration to the dorsal aorta, differentiation of NCCs into neurons, aggregation/migration of neurons to form either the paravertebral sympathetic chains or the parasympathetic cardiac ganglia, and finally extension of axonal projections into cardiac tissue and terminal differentiation. Throughout this period, extending into early postnatal life, autonomic neurons and their precursors display substantial plasticity. From a developmental perspective, as autonomic control matures, there can be serious consequences for the fetus/infant if blood pressure and heart rate are unduly perturbed. In normal infants, sympathetic cardiac control decreases with postnatal age and parasympathetic control over heart rate increases.Citation40 Conversely, in preterm infants, since maturation is incomplete, blood pressure and heart rate control are impaired and may be causative in sudden infant death syndrome.Citation40-Citation42 Preterm/low birth weight infants also have an increased risk of developing hypertension, coronary heart disease and ischemic heart disease in adulthood.Citation43-Citation46 Although a link with cardiac autonomic control has not been established for this group of at risk children, the ANS may be causative in a number of childhood cardiovascular disease states as it certainly is in adulthood. For example, it has already been demonstrated that increased cardiac sympathetic activation and parasympathetic depression occurs in low-income childrenCitation47 and children with sickle cell anemia.Citation48 Certainly, adult rat offspring of either nutritionally-deprived mothers,Citation49 or obese mothers,Citation50 have elevated sympathetic tone that manifests in increased blood pressure and heart rate. Similarly, in genetically hypertensive rats, significant sympathetic acceleration of the heart is observed in the early postnatal period (P4) suggesting a possible mechanism for subsequent hypertension development.Citation51 Altered autonomic maturity in infancy may therefore have long-lasting consequences, and this is an expanding field of study.
Although deficits in cardiovascular regulation can occur at all levels of neuronal control, there is increasing appreciation that aberrant nerve growth and plasticity in the effector cardiac nerves may have major roles in many cardiac disease processes. Intriguingly, many of the neuroplastic alterations observed in adult cardiac ANS neurons can be traced back to various stages in their development, so the importance of further delineating ontogenetic pathways for these neurons is of substantial importance for future translational studies. This is particularly true for parasympathetic cardiac ganglion neurons for which developmental mechanisms have not been worked out as well as for cardiac sympathetic neurons. The focus of this review is on the postganglionic ANS neurons and not on central and descending ANS pathways, which constitute a separate topic. I hope to demonstrate, however, that plasticity in adult postganglionic cardiac neurons is a robust phenomenon that can be linked to developmental pathways, and that is amenable therefore to physiological manipulations for reversing altered autonomic tone in conditions as varied as the metabolic syndrome, congestive heart failure and arrhythmia generation.
Development of Cardiac Autonomic Innervation
Migration of neural crest cells and ganglia formation
Cardiac sympathetic system
Although the primitive human heart starts to beat at 21 to 22 d, heart development continues to day 50, and it is near the end of this period, during the fifth week, that thoracic neural crest cells migrate from the neural tube through the somites and form aggregations (ganglia) near the dorsal aorta.Citation52 The initial NCC migration, subsequent differentiation, neuronal migration and ganglia formation, and axonal projections to target tissue and terminal differentiation, have all been reviewed in recent yearsCitation53-Citation65 and are only briefly summarized here.
The NCCs are derived from the dorsal part of the closing neural tube under the ectoderm. Migration of trunk NCCs (destined to be sympathetic neurons) along a ventral pathway occurs with migration restricted to the rostral portion of each somite allowing subsequent metameric patterning.Citation66-Citation68 The migration of these NCCs is restricted to the ventral route through expression of repulsive cues such as ephrinB1 on the caudal side of the somites, and its receptor (EphB2) on NCCs.Citation69-Citation71 These progenitor cells become fate-restricted within 30–36 h post-migration.Citation72 Once at the region dorsolateral to the developing dorsal aorta, a final migration of cells occurs either in the rostral or caudal directions in order to make up the paravertebral sympathetic chain followed by a period of rapid mitosis.Citation73-Citation75 Final migration of cells that constitute paravertebral sympathetic ganglia may be directed by a member of the glial cell line-derived neurotrophic factor (GDNF) family, artemin,Citation55,Citation76-Citation78 which is expressed both in the dorsal aorta and along major vessels along which sympathetic axons grow. The patterning of discrete sympathetic ganglia involves Eph-ephrin repulsion as occurs with NCC migration from the neural tube; in addition, adhesive cell-cell contacts at ganglia sites occur through N-cadherin expression by the sympathetic progenitor cells.Citation69
Migration of sympathetic progenitor NCCs to the dorsal aorta involves additional repulsive cues through signaling from the transmembrane protein neuropilin. Neuropilin 1 (NRP1) binds to either semaphorin 3A (SEMA3A) or to an isoform of the vascular endothelial growth factor receptor, termed VEGF164 in mice. Pertinent to sympathetic neuron precursors, Nrp1 and Sema3a-null mice have defective trunk NCC migration resulting in formation of both ectopic sympathetic ganglia and a malformed stellate ganglion.Citation79-Citation81 There are functional consequences for abnormal sympathetic ganglion formation in Nrp1 and Sema3a-null mice, including sinus bradycardia in these mice.Citation82,Citation83 Importantly, the repulsive role of SEMA3A continues throughout developmental axonal patterning in the heart, and is believed to be causal in the epicardial to endocardial gradient of cardiac sympathetic nerves,Citation8,Citation84 probably through semaphorin receptors A3 and A4 expression on sympathetic axons.Citation65,Citation81,Citation85
Cardiac parasympathetic system
NCCs destined for the heart and parasympathetic cardiac ganglia delaminate from the neural folds at the level of the hindbrain. In contrast to the sympathetic ganglia, NCCs destined to form parasympathetic cardiac ganglia do not migrate only in the rostral part of somites, and can run either through the somites or lateral to them; these NCCs do not aggregate at the dorsal aorta but enter the heart along tracts laid down by the vagus nerve.Citation63,Citation86-Citation88 At a temporal level, in both avian and mammalian embryos, parasympathetic NCCs precede the arrival of sympathetic innervation, and parasympathetic innervation is functional before that of the sympathetic system.Citation29,Citation89-Citation92 Although evidence for guidance cues for cardiac ganglion precursors is lacking, NCCs that make up this ganglion also contribute to development of the outflow tracts and aortic arches (the cardiac neural crest).Citation60,Citation93,Citation94 It is highly instructive that the semaphorin receptor, plexinA2, is expressed by this subset of cardiac NCCs, and that in mice lacking semaphorin 3C, congenital heart conditions exist including an interrupted aortic arch and persistent truncus arteriosus.Citation78,Citation95 Although the role of the plexinA2-semaphorin 3C axis has not been delineated for NCCs destined to be cardiac ganglion neurons, the available data suggests that, unlike sympathetic precursors for whom semaphorins serve as repulsive cues, cardiac ganglion-destined NCCs may actually rely on semaphorins for attractive cues during their migration. A role in cardiac ganglion precursor migration has also been suggested, but not confirmed, for the neural adhesion protein HNK1 (human natural killer-1/CD57);Citation96 however, it is evident that there is scope for more research in this regard.
Differentiation of cardiac autonomic neurons
Cardiac sympathetic neurons and progenitors
NCCs aggregate and differentiate in the vicinity of the dorsal aorta before their final migration to paravertebral sympathetic ganglion sites. Bone morphogenetic proteins (BMPs), released from smooth muscle cells of the dorsal aorta, initiate a chain of transcriptional events in NCCs that cause terminal differentiation through expression of neuronal specific genes and those associated with a noradrenergic phenotype. The evidence for BMPs central role in NCC differentiation is comprehensive; in exquisite studies, Rohrer and colleagues showed that implanting noggin (BMP4–7 inhibitor)-releasing beads in avian embryos caused an interruption in NCC differentiation.Citation53,Citation56,Citation97 Similarly, BMP-2 is expressed in the murine dorsal aorta and increases both the rate and extent of NCC differentiation.Citation98,Citation99 Transcriptional factors are activated in a distinct sequence in avian NCCs, with some responsible for neuronal differentiation and others for acquisition of catecholaminergic traits. Of significant importance among these transcriptional factors is the homeodomain protein Phox2b whose elimination interrupts development of most of the peripheral nervous system in mice.Citation100 In addition to Phox2b, Ascl1/Mash1/Cash1, Phox2a, Gata2/3 and Hand2, all have distinct roles in differentiation. Ascl1 has been implicated in development of neuronal markers for both sympathetic and parasympathetic CG neurons,Citation99,Citation101,Citation102 Phox2a, and Phox2b, are positive regulators for expression of the noradrenergic synthesizing enzyme dopamine β-hydroxylase (DBH),Citation103-Citation105 Gata3 is vital for tyrosine hydroxylase (TH; rate-limiting enzyme in NE synthesis) expression,Citation106-Citation108 and Hand2 is essential for maintaining the catecholaminergic phenotype in developmentCitation109-Citation113 and in adults.Citation114 In addition to broad control exerted by dorsal aorta BMPs over these transcription factors, a degree of cross-regulation exists, including repression of Ascl1 by Hes genes, which are effectors of Notch signaling.Citation115 In addition, a role for microRNAs in the differentiation of sympathetic NCCs has recently been proposed; deletion of the microRNA-processing gene Dicer in mouse embryos causes severe hypoplastic sympathetic ganglia in E15 but not E11-E13 embryos.Citation116 These data suggest that microRNAs are not involved in the initial formation of sympathetic ganglia but are required for neuronal survival once NCCs have begun the process of terminal differentiation. A similar role of GDNF has also been recently suggested for murine sympathetic NCC precursors (starting at E13), with GDNF synthesized from NCCs promoting autocrine/paracrine survival of sympathetic progenitors.Citation117 Further, Krieglstein and colleaguesCitation117 also provide evidence for a limited survival-promoting effect of GDNF in cultured neonatal sympathetic neurons.
Cardiac parasympathetic neurons and progenitors
Although parasympathetic cardiac ganglia follow similar developmental mechanisms to sympathetic neurons, such as BMP-induced transcriptional differentiation cascades, there are some fundamental differences. Neither Gata3 nor Hand2 are expressed by developing cholinergic parasympathetic ciliary ganglion neurons.Citation109,Citation118,Citation119 Similarly absent are the transcription factors AP-2β and HoxB8Citation56,Citation120 that are expressed in migrating NCCs and are involved in promotion of DBH and NE.Citation121,Citation122 Extrapolating these findings to the cardiac ganglia may not be accurate, however, as parasympathetic ganglia do have differences in their differentiation mechanisms in comparison to other parasympathetic ganglia. A notable example in this regard is that cardiac ganglia are normal in Phox2a null mice, in stark contrast to the cranial pterygopalatine and otic parasympathetic ganglia that are absent in these mice.Citation123 A rostral-caudal gradient for Phox2a dependency has been suggested by the authors to explain this finding; however, it remains to be determined what other developmental programs additionally regulate cardiac ganglion neuronal survival and differentiation.
Cardiac-derived control over neuronal survival and outgrowth
Establishment of functional cardiac autonomic control
Establishment of myocardial innervation occurs at different times depending on the species investigated. In rats and rabbits there are essentially no cardiac sympathetic fibers before birth, however, in guinea pigs and sheep innervation is established in the fetal heart (E85 onwards in sheep).Citation124,Citation125 These structural studies also agree with pharmacological observations in fetal/neonatal sheep and pigs demonstrating progressive increases in parasympathetic regulation of basal heart rate.Citation126-Citation129 In seminal studies, it was demonstrated that functional control of heart rate in the atria by sympathetic nerves is established by P2 in rats; however, sympathetic tone becomes progressively weaker (maximal heart rate at P8) as the parasympathetic system matures close to weaning (P24).Citation130 Similarly, in sheep, the resting heart rate declines postnatally, due to increased parasympathetic activity, reaching the resting adult rate by the eighth week.Citation131,Citation132 Establishment of heart rate control in humans displays a similar developmental profile to that in sheep.Citation40
Sympathetic axonal outgrowth and patterning
A decrease in sensitivity to NE is observed in both atria and ventricles from developing rats after onset of sympathetic innervation, indicating that sympathetic nerves actively regulate agonist sensitivity.Citation133,Citation134 The basis for increased sensitivity to catecholamines prior to innervation is that in the postnatal period, and certainly earlier in embryonic development, heart rate control is effected through additional sources, including catecholamines from the adrenal medulla and possibly extra-adrenal chromaffin tissue.Citation130 Although not a focus of this review, it is important to note that the embryonic heart has an absolute dependence on catecholamines prior to development of functional sympathetic innervation (dependency from E9.5–13.5 in the mouse; equivalent to 4–6 weeks after fertilization in humans).Citation135-Citation139 As an aside, the β-adrenoceptor agonist isoproterenol can cause cardiac hypertrophy in both developing and adult ratsCitation140,Citation141 confirming an intimate two-way trophic relationship between nerves and cardiomyocytes. Intrinsic cardiac adrenergic (ICA) cells may be the source of catecholamines, primarily epinephrine, in the developing rodent and human heart and contribute up to one-third of total cardiac epinephrine content.Citation139,Citation142,Citation143 The neonatal rat heart has functional α1 in addition to β adrenergic receptors, allowing broad responsiveness to epinephrine, particularly for α1-promotion of myocardial hypertrophy, with β1 adrenergic receptor control of heart rate increasing with postnatal age.Citation139,Citation144,Citation145 Although the fate of ICA cells is not certain, some may differentiate into pacemaker cells, however, a resident population is potentially the source of adrenergic support following transplantation.Citation146,Citation147 ICA cells are not believed to be of NCC origin and are distinct from small intensely fluorescent (SIF; ) cells that populate autonomic ganglia and may function as interneurons.Citation148-Citation150
Trophic factor control over outgrowth and survival of cardiac sympathetic neurons
The modulation by neurotrophic proteins, released from targets and exerting control over innervating post-mitotic neurons, occurs late in development, typically in the immediate pre- and post-natal periods. However, it is not only the end targets that can secrete neurotrophins, indeed neurotrophin-3 (NT-3) is secreted from blood vessel smooth muscleCitation151 as sympathetic nerves “piggy-back” on the vasculature to reach diverse targets including the heart. In NT-3 null mice, cardiac projecting axons from the stellate ganglion are shorter, and indeed sympathetic axons are not observed in the heart at E15.5–16.5.Citation152 Once sympathetic axons reach the heart, dependency on NT-3 switches to the prototypical neurotrophin, nerve growth factor (NGF). Of interest in this regard is that both NT-3 and NGF are essential for local axonal growth of post-mitotic sympathetic neurons; however, while both neurotrophins utilize the TrkA receptor for this function, NT-3 is unable to initiate survival-promoting retrograde signaling through TrkA.Citation152,Citation153 Pertinent to the epicardial-to-endocardial gradient of sympathetic ventricular coronary innervation, Mukouyama and colleaguesCitation154 have recently provided some fascinating insight in this regard for the developing mouse heart. A two-stage process is involved; first an initial phase of NGF-directed nerve growth occurs (E13.5–15.5) along large diameter venous subepicardial vessels, nerves grow first along the dorsal aspect of the ventricles followed by innervation of more ventral epicardial areas. NGF expression within venous smooth muscle is then decreased as deeper arteries now increase smooth muscle NGF synthesis (E16.5–17.5), thereby facilitating arterial and myocardial innervation, and establishing the epicardial-to-endocardial gradient.Citation154
In addition to NGF and NT-3, artemin,Citation76,Citation77,Citation155 a member of the GDNF family, and endothelin-3Citation156 are also important in sympathetic axonal guidance to their targets and have been recently commendably reviewed.Citation55 In parallel with neurotrophin-directed nerve ingrowth, the neural chemorepellent semaphorin-3A is expressed strongly in the subendocardium of the mouse heart (E12–15) before being downregulated by P42.Citation81 Semaphorin-3A may therefore work in concert with NT-3/NGF for patterning of the heart including the epicardial-to-endocardial innervation gradient. Functionally, semaphorin-3A−/− mice have sinus bradycardia and premature ventricular contractions (PVCs), whereas mice overexpressing semaphorin-3A in cardiomyocytes display inducible ventricular tachyarrhythmias.Citation81 The aberrant innervation pattern in semaphorin-3A−/− mice is probably causal in the increased incidence of PVCs in these mice. In the semaphorin-3A overexpressing mice, sympathetic ventricular innervation is normal in the subepicardium but substantially reduced in the subendothelium, a pattern inverse to that of semaphorin-3A expression. Intriguingly, in sensory neurons, the pan-neurotrophin receptor p75NTR is able to interfere with semaphorin-3A activity, by acting as a competitive inhibitor to semaphorin-3A receptors, and thereby reducing functional semaphorin-receptor complexes.Citation157 Of interest for cardiac-projecting sympathetic neurons is the demonstration in p75NTR−/− mice, of a ventricular innervation pattern similar to that observed in semaphorin-3A overexpressing mice,Citation158 suggesting that in the absence of p75NTR, sympathetic innervation is more responsive to disruptive cues from semaphorin-3A.Citation65,Citation158
Sympathetic neuronal survival
Once sympathetic nerves reach their targets in the heart, target-derived NGF plays a critical role in neuronal survival, patterning and synaptic strength. The level of NGF in the adult mouse heart corresponds to extent of sympathetic innervation, with atria having higher protein and RNA levels than ventricles.Citation159 In the developing mouse heart, sympathetic nerves reach their ventricular targets at E12 when NGF first becomes detectable; NGF levels then rapidly increase reaching adult levels by E14.Citation160 However, NGF levels are not static after E14, with a decline at birth (E18/P0) followed by a second peak (P8) then decreasing and stabilizing back to adult levels by P21. Sympathetic neurons in the first postnatal week compete for target-derived NGF, as NGF levels in the immediate postnatal period, despite fluctuations, are still subsaturating, with apoptosis occurring for those neurons unable to obtain sufficient levels of NGF.Citation161-Citation166 In addition to neuronal survival, NGF also enhances synaptic strength between sympathetic neurons and neonatal cardiomyocytes by promoting the formation of terminal varicosities.Citation167,Citation168 Intriguingly, NGF may also modulate β3-adrenergic receptor expression by cardiomyocytes, a finding with possible ramifications for arrhythmia generationCitation169 as β3-adenoceptor expression is increased in some cardiac pathological states. NGF is thus essential for many aspects of sympathetic neuronal maturation.
Initiation of NGF expression by cardiomyocytes may occur from factors released by the innervating axons; however, a prominent role for endothelin-1 has been recently identified. Endothelin-1 promotes cardiomyocyte NGF expression and endothelin-1-deficient mice have substantial reductions in stellate ganglion neuron numbers (55% of wildtype in E18.5 embryos), cardiac innervation and cardiac norepinephrine content.Citation170 Cardiac NGF levels in endothelin-1-deficient mice decrease from E15.5–18.5, corresponding with decreased stellate ganglion numbers and restriction of sympathetic nerves to the ventricular epicardium. However, these defects can be reversed with cardiac-specific overexpression of NGF in endothelin-1-deficient mice.Citation170
With regard to cardiac sources of NGF, vascular smooth muscle and cardiomyocytes have been the focus of most investigators; however, there may also be alternative sources. The sympathetic neurite-promoting role of Schwann cells/support cells/glia through NGF synthesis has been examined, particularly after injury.Citation171-Citation174 We have demonstrated that, in addition to synthesis by support cells, NGF is synthesized and secreted from neuronal soma of developing and adult rat sympathetic neurons.Citation175 Levels of NGF transcripts are low in late embryogenesis (E17), peak in postnatal rats (P0–28) before decreasing to adult levels. Although NGF transcripts are demonstrable in sympathetic neurons in this study, both tissue content and neuronal secretion analysis indicate that the predominant form of NGF expressed are high molecular weight pro forms of the protein. Despite abundancy of proNGF in sympathetic neurons, the 13.5 kDa is still present suggesting some intracellular processing occurs; however, most cleavage of NGF occurs extracellularly and our data are consistent with this concept. The physiological significance of neuronally derived NGF remains to be determined for developing sympathetic neurons and will be addressed further in the adult plasticity section Mechanisms for sympathetic nerve growth; NGF from alternate sources.
Cardiac parasympathetic innervation development
Parasympathetic innervation in the avian and rat heart precedes that of the sympathetic system, with nerves arriving shortly before birth (E19 in rats).Citation28,Citation29,Citation176 Neuroeffector transmission for the parasympathetic system is established in the rat heart by E21 with higher transmission in atria than ventricles, and parasympathetic activity increases with postnatal age reaching adult levels by P14,Citation176 similar to what has been mentioned earlier for other species. In the frog heart, cardiac ganglion neurons do not undergo postnatal programmed cell death in order to match neurons to target, as occurs in the sympathetic system.Citation177 Instead, cardiac cells tightly regulate neuronal growth and proliferation, presumably through release of neurotrophic factors. In the frog cardiac ganglion, SIF cells are derived from similar NCC cells and appear at the same time as parasympathetic progenitors, however, the rate of proliferation is faster for neuronal progenitors and early on a 10 to 1 ratio is established that continues into adulthood.Citation178 Similarly in rat sympathetic ganglia, SIF cells are first observed at E13 with numbers declining postnatally before increasing from P14–21 to adult levels.Citation179,Citation180 The parallel development and migration of SIF cells with their neuronal counterparts suggests that the interneuron role of these cells is crucial for optimal autonomic ganglion function.
For cardiac parasympathetic innervation, GDNF and neurturin (a member of the GDNF family) are involved in both trophic support and patterning of innervation. GDNF and neurturin signal by first binding to their cognate receptors, GFRα1 and α2 respectively, and then forming a complex with the c-Ret tyrosine kinase (ret).Citation181-Citation183 Cranial parasympathetic ganglia are dependent on GDNF for survival early in development then switch dependency to neurturin by postnatal day 0.Citation184 Similarly, a central role of neurturin in the development and maintenance of cardiac ganglion neurons has been demonstrated from the use of ret or neurturin knockout mice.Citation185 Neurturin knockout mice have reduced numbers and size of cardiac ganglion neurons, reduced sinoatrial node innervation, attenuated atrial acetylcholine content and attenuated bradycardiac responses to vagal stimulation.Citation186,Citation187 Although neuronal loss in adult neurturin knockout mice is appreciable, about 35% of cardiac ganglion neurons survive and these may have parallel trophic factor dependencies, either to other members of the GDNF family,Citation184,Citation188 or to additional trophic factors.
Aside from members of the GDNF family, what alternative neurotrophic factors may also modulate cardiac parasympathetic neurons? In this regard, a novel finding recently was the demonstration of neurotrophic receptors TrkA and p75NTR on mouse cardiac ganglion neurons.Citation189 Although p75NTR−/− mice have normal atrial innervation and acetylcholine content and release,Citation190,Citation191 there may be a trophic role of NGF or NT-3 on these neurons. Certainly, in non-cardiac parasympathetic neurons, a role for non-GDNF family neurotrophins is well established. For example, NT-3 can promote outgrowth from pelvic ganglion parasympathetic neuronal explantsCitation192 and these neurons upregulate cholinergic markers, including the neuropeptide VIP, when treated with NGF in culture.Citation193 NGF also increases excitability of airway parasympathetic neurons and augments dendritic growth.Citation194 From a developmental perspective, the mouse parasympathetic ciliary ganglion displays neuritogenesis in culture that is NGF-responsive from E6–11.Citation195,Citation196 We postulated that cardiac parasympathetic neurons may utilize NGF as an autocrine/paracrine factorCitation197-Citation199 after demonstrating NGF transcripts and protein within adult rat parasympathetic pterygopalatine and cardiac ganglion neurons. Recently, high frequency electrical stimulation in adult canines has been shown to induce a number of neurotrophins in cardiac ganglion neurons of the interatrial ganglionated plexus, and this neurotrophin upregulation is accompanied by increases in soma size, increased acetylcholine release (NT-3 mediated) and increased outgrowth in culture (NGF-mediated).Citation200 I will address the issue of autocrine/paracrine release of neurotrophins from cardiac autonomic neurons later (Mechanisms for sympathetic nerve growth; NGF from alternate sources); however, it is clear that, aside from GDNF family members, members of the neurotrophin family may also regulate cardiac ganglion neurons in development and into adulthood.
In addition to target-derived factors patterning cardiac autonomic nerves, nerves in turn can also influence development of coronary vessels, including both patterning and differentiation, primarily through release of vascular endothelial growth factor-A (VEGF-A; reviewed by refs. Citation201 and Citation202). In contrast, VEGF can also act as a neurotrophic factor, and NGF/BDNF/NT-3 as trophic factors for vascular smooth muscle, endothelial cells, and cardiomyocytes (reviewed by refs. Citation203–Citation205), suggesting multiple levels of cross-regulation for both angiogenic and nervous patterning. Although this area is not a focus of this review, I would draw particular attention to the findings of Hempstead and colleaguesCitation206 showing that in mice with a knockout of the trkC neurotrophin receptor (specific for NT-3 signaling), heart development is grossly abnormal including presence of valvular and septal defects, pulmonic stenosis and a globular heart.
Adult Plasticity in Cardiac Autonomic Neurons
Sympathetic plasticity in the heart
Sympathetic neuronal remodeling has been implicated in a number of cardiovascular disease states. Aside from structural changes, alterations in neurochemistry and in activity can also occur. The concept of sympathetic overactivity, usually accompanied by reduced parasympathetic activity and heart rate variability, is increasingly recognized as a feature in the pathogenesis of a number of cardiovascular pathologies and has been reviewed recently.Citation207-Citation209 From a clinical perspective, central-acting sympatholytic drugs, such as moxonidine and rilmenidine, have beneficial effects on blood pressure control in hypertensive patients by reducing central sympathetic outflow.Citation210,Citation211 Beta-adrenergic blockade in post-infarct and heart failure patients has also shown clear clinical benefits, partly through targeting of excessive cardiac norepinephrine release. There is increasing appreciation of β-blockers for preventing sudden cardiac death, atrial fibrillation and arrhythmias in patients with underlying cardiovascular disease (clinical benefits reviewed by refs. Citation208, Citation212–Citation216).
Sympathetic nerve growth contribution to arrhythmia generation
Side effects of conventional drugs that target the sympathetic system may involve perturbations in cardiac nerve plasticity. Recently, the β2-adrenoceptor agonist, terbutaline, used illicitly for management of preterm labor, was shown to attenuate cardiac sympathetic neuronal development in neonatal rats after maternal agonist treatment.Citation217 In contrast, β-adrenoceptor antagonists, used routinely in management of various situations of increased sympathetic drive, including hypertension and congestive heart failure, cause sympathetic ventricular hyperinnervation in rats.Citation218 The mechanism for this sympathetic outgrowth appears to be through inhibition of β1-adenoceptor autoreceptors that normally inhibit adult sympathetic axonal growth. Since neuronal remodeling can promote arrhythmia generation, an underappreciated aspect of β-blocker therapy may be in neuroplastic responses of postganglionic sympathetic neurons to these agents.
In addition to pharmacological promotion of sympathetic growth, cardiac neuronal remodeling accompanies hypertension, post-myocardial infarct remodeling, and heart failure. Indeed, Chen and colleaguesCitation219 postulated the nerve-sprouting hypothesis of sudden cardiac death in order to link nerve sprouting and electrical remodeling. The role of nerve sprouting in the generation of arrhythmias has been extensively reviewed;Citation38,Citation220-Citation227 so I will only touch on some points particularly relevant to the topic of this review. A number of studies have demonstrated the presence of aberrant sympathetic, or indeed parasympathetic, outgrowth in human and canine hearts with atrial fibrillation.Citation228-Citation237 Recognition that ectopic or reentrant activity occurs at locations where autonomic fibers aggregate, such as the ligament of Marshall,Citation238-Citation240 has allowed ablation therapy, or localized cardiac denervation or block, to become a common option for reversing atrial or ventricular fibrillation incidence.Citation238,Citation241-Citation244 However, some caution in effectiveness of these therapies comes from the residual presence of scar tissue or fibrosis that will continue to serve as a substrate for arrhythmia generation.Citation245 In addition, a potential role of the parasympathetic neuropeptide vasoactive intestinal polypeptide was demonstrated for promotion of atrial fibrillation in a canine model,Citation246 suggesting that pharmacological block strategies may need to consider neuropeptides as well as classical neurotransmitters.
Foci for ventricular arrhythmia generation are much more likely to develop in areas where electrical signaling is discontinuous, such as an area of fibrosis, or where the myocardium is hypersensitive to catecholamines due to functional or pathological denervation. Consequently, aberrant sympathetic innervation will promote heterogeneity in propagation of action potentials and thereby be causal in ventricular tachyarrythmias and consequent sudden cardiac death. This mechanism has received strong support from studies in canines and humans where correlations between ventricular arrythmias and regional cardiac hyperinnervation have been demonstrated.Citation219,Citation247
Mechanisms for aberrant sympathetic nerve growth; NGF
Given the compelling evidence for a role of sympathetic nerve sprouting in generation of both atrial and ventricular arrhythmias, what underlying mechanism could induce this aberrant growth pattern? Similarly, partial sympathetic re-innervation occurs in the transplanted heart; the mechanisms involved in this nerve re-growth phenomenon also remain to be determined.Citation248 NGF is the obvious candidate for directing nerve growth. After myocardial infarction we, and others, demonstrated a close spatial relationship between hyperinnervating neurons in the ventricular peri-infarct area and NGF-synthesizing inflammatory cells, including macrophages and modified fibroblasts known as myofibroblasts.Citation249-Citation251 In explant culture, neuritogenesis from cardiac sympathetic neurons is markedly increased by peri-infarct myocardium, and this effect is blocked with a function-blocking antibody to NGF. In parallel studies, NGF infusion into the left stellate ganglion of canines causes ventricular sympathetic nerve sproutingCitation252 confirming a role for NGF. NGF upregulation by cardiomyocytes is also implicated in sympathetic hyperinnervation after myocardial infarction or cardiac hypertrophy.Citation253,Citation254 Indeed, the cardiac hypertrophic factor endothelin-1 induces NGF in cardiomyocytes.Citation170 NGF is thus strongly implicated as critical for ventricular sympathetic hyperinnervation; whether it has a role in abnormal atrial innervation patterns linked to atrial fibrillation remains to be determined.
In addition to sympathetic hyperinnervation in the ventricles, shown in a number of models including the human,Citation251,Citation252,Citation255-Citation257 a recent finding was that robust and prolonged sympathetic hyperinnervation also occurs in cardiac-projecting stellate ganglia after acute myocardial infarction. Of particular interest in the latter study is the demonstration that sprouting occurs for both adrenergic and cholinergic axons in the sympathetic ganglion, suggesting that potential NGF-responsiveness is not selective for neuronal phenotype in this model.Citation258 Although sympathetic fibers are the predominant population in the ventricles, at least in the porcine heart significant ventricular cholinergic fibers also exist.Citation259 Whether some of this ventricular cholinergic innervation is actually derived from cholinergic sympathetic neurons remains to be determined, but would certainly add an intriguing dimension to the findings of cholinergic hyperinnervation in the stellate ganglia.
Mechanisms for sympathetic nerve growth; NGF from alternate sources
A possible parallel mechanism for sympathetic sprouting is through localized NGF-release from the axons themselves. As discussed earlier, we showed that NGF is synthesized and released by developing and adult sympathetic neurons primarily as the pro isoform.Citation175 Recently, the expression and secretion by sympathetic neurons of matrix metalloproteinases,Citation220 capable of cleaving proNGF to the mature moiety, as well as neuronal NGF upregulation following electrical stimulation,Citation220 suggests that NGF release by sympathetic neurons is followed rapidly by conversion to the growth-promoting form. Since proNGF promotes sympathetic axonal degeneration in the relative absence of the mature moiety,Citation260-Citation262 the NGF/proNGF ratio will direct physiological effects. These data certainly raise the fascinating possibility that regulation of extracellular cleavage by the neurons themselves may be essential for either the pro-growth (NGF-directed), or potentially pro-apoptotic (proNGF-directed), fate of sympathetic neurons in pathological states.
In addition to autocrine/paracrine release of NGF from sympathetic nerves, a non-intuitive source of NGF in the heart is from parasympathetic cardiac ganglion neurons. Presynaptic release of reciprocal neurotransmitter, acetylcholine inhibition by norepinephrine and vice versa, constitutes a critical regulatory mechanism for heart rate control.Citation3,Citation13,Citation26,Citation263,Citation264 Sympathetic and parasympathetic fibers are closely apposed in effector pacemaker and conduction areas and we showed that maintenance of this spatial association was through NGF synthesis and release by cardiac ganglion neurons.Citation197-Citation199 Not only did cardiac ganglion neurons express NGF, but this expression was dependent on intact sympathetic innervation as cardiac sympathectomy reduced NGF synthesis. In recent studies, we demonstrated that β-adrenoceptor activation on cardiac ganglion neurons promotes NGF synthesis by cardiac ganglion neurons. Further, in congestive heart failure, where cardiac autonomic function is dysregulated, NGF expression by cardiac ganglion neurons is also attenuated suggesting disruption of autonomic crosstalk (Hasan W., Smith P.G.; unpublished data). Is there some relevance here to findings in humans, and in experimental models, that NGF levels in the failing heart are also attenuated as cardiac NE levels decreaseCitation265-Citation267? There certainly seem to be some parallels between these findings and additionally, once disruption to sympathetic-parasympathetic crosstalk occurs, vagal influences that would normally dampen sympathetic outflow may no longer be in a position to effect such a change. Maintenance of atrial sympathetic-parasympathetic connections in heart failure is probably critical therefore toward attenuation of disease progression.
The synthesis of NGF by cardiac sympathetic and parasympathetic neurons is certainly intriguing but does it have a physiological role as compared with the “classical” cardiac sources of NGF from cardiomyocytes and vascular smooth muscle? In this regard, a recent study by Rana and colleaguesCitation220 has demonstrated that cultured sympathetic neurons have 3-fold higher protein content of NGF than equivalent numbers of atrial cardiomyocytes. The authors also showed that electrical field stimulation not only increased NGF expression within the neurons but also attenuated the same in the cardiomyocytes. Granted that this studyCitation220 was performed with neonatal tissue, however, it certainly provides some food for thought concerning the contribution of neuronally-derived NGF on promotion of sympathetic hyperinnervation.
Sympathetic nerve denervation in heart failure and reduced NGF levels
Although cardiac sympathetic hyperinnervation is a robust phenomenon in several cardiovascular pathologies, it is also established that cardiac autonomic nerves are generally dysfunctional as pathology progresses. In situations of myocardial infarction a patchy sympathetic re-innervation pattern occurs in the newly developed scar;Citation268-Citation270 denervation may occur if the infarct interferes with apical pathways.Citation271 As heart failure develops, sympathetic nerves excessively release NE whereas normal NE re-uptake is disrupted, promoting myocardial fatigue.Citation267,Citation272-Citation276 Examination of the temporal course of cardiac norepinephrine spillover in heart failure patients indicates that sympathetic nerve activity is increased earlier and to a greater extent than sympathetic activity to other organs.Citation277-Citation279 In part, this increased sympathetic activity may be due to a parallel attenuation in parasympathetic tone, as withdrawal of parasympathetic inhibition of norepinephrine release contributes to greater norepinephrine spillover in cardiac tissue as compared with other vascular beds.Citation275,Citation280 Eventually, with heart failure progression, reduced NGF expression promotes widespread myocardial denervation.Citation265,Citation281,Citation282 Intriguingly, adrenergic stimulation, mechanical stretch and the stretch activated serine/threonine phosphatase calcineurin, all reduce NGF release from cultured cardiomyocytes.Citation283 In the progression of heart failure, therefore, chronic elevated adrenergic tone and mechanical stretch may be instrumental in attenuating cardiac NGF expression.
Sympathetic nerve denervation, inflammatory cytokines and parasympathetic function
The role of inflammatory cytokines of the gp130 receptor family, including interleukin-6 (IL-6), cardiotropin-1, leukemia inhibitory factor (LIF) and ciliary neurotrophic factor (CNTF), has been examined in depth primarily by Habecker and colleagues, who have demonstrated cytokine modulation of sympathetic function after acute myocardial infarction. In contrast to chronic coronary artery ligation and rapid atrial pacing models, after acute ventricular ischemia-reperfusion an elevated level of cardiac cytokines promotes sympathetic denervation in peri-infarct areas lateral and apical to the site of ligation.Citation284 Cytokines also cause local suppression of norepinephrine, tyrosine hydroxylase (TH) and norepinephrine transporter (NET) and increase TH degradation.Citation285,Citation286 However, an unexpected augmentation in extracellular and plasma norepinephrine is also seen in ventricles after ischemia-reperfusion, and coupled with increased TH and NET levels in stellate ganglia, suggest that cytokines provoke a generalized sympathetic activation despite local inhibition of noradrenergic components.Citation287-Citation289 Of particular relevance to developmental plasticity is the ability of cytokines to modulate transcription factors, like Hand2, that have a role in sympathetic neuronal differentiation.Citation290 By altering expression of transcription factors involved in neuronal differentiation, inflammatory cytokines may cause re-expression of a developmental phenotype, akin to that which occurs in cholinergic sympathetic neurons that are initially adrenergic.Citation291,Citation292 Although cholinergic sympathetic neurons do not project to the heart, and constitute only a small proportion overall of sympathetic neurons, it is noteworthy that in heart failure, transdifferentiation of adrenergic neurons into cholinergic neurons, probably through LIF and CT-1 activation of gp130 receptors, has been demonstrated in the adult rat.Citation293 Whether similar alterations occur in human heart failure remains to be determined, and the physiological relevance of increased sympathetic cholinergic projections to the heart is also unknown. However, increased cholinergic release in heart failure may be a positive compensatory mechanism given well-known beneficial effects of vagal stimulation on cardiac function (see Parasympathetic plasticity in the adult heart and therapeutic vagal stimulation).
In addition to regulation of sympathetic transmitter synthesis and transport, and differentiation of phenotype, inflammatory cytokines can also regulate neuropeptide expression within sympathetic neurons. Neuropeptides like NPY and galanin, released from sympathetic neurons, can inhibit acetylcholine release from co-projecting cardiac parasympathetic nerves.Citation15,Citation16,Citation294-Citation300 Release of these neuropeptides in situations of increased sympathetic drive, as in heart failure, is likely to additionally contribute to reduced vagal/parasympathetic tone in the failing heart. Of potential interest in this context is that vagus nerve stimulation reduces cytokine release from inflammatory cells.Citation301,Citation302 Although the heart has not been a focus of the latter studies, it is certainly possible that some of the beneficial effects of vagal stimulation on cardiac function (addressed in Parasympathetic plasticity in the adult heart and therapeutic vagal stimulation) are related to inhibition of cytokine effects on sympathetic function, a somewhat contradictory conclusion to that arrived earlier for cholinergic differentiation of adrenergic neurons in heart failure. What is certainly clear is that sympathetic remodeling has many facets and modes of regulation and that interactions with the parasympathetic system are a key component for maintenance of normal sympathetic innervation and function.
Autonomic nerve dysfunction in diabetes and obesity
Cardiac autonomic neuropathy is a frequent complication of diabetic mellitus, and diabetic patients are at high risk for developing arrhythmias, silent myocardial ischemia and sudden cardiac death.Citation303-Citation305 Autonomic tone is disturbed in diabetic hearts and gets progressively worse, with reduced parasympathetic tone preceding sympathetic effects; resting tachycardia is followed by some reduction in heart rate as the sympathetic system gets affected; however, an impairment in stress tests develops and eventually cardiac denervation results in reduced heart rate variability.Citation303-Citation306 Significantly, structural remodeling of autonomic nerves in the diabetic heart occurs for both arms of the autonomic nervous system. The density of sympathetic and parasympathetic fibers is reduced in atria from diabetic mice,Citation307 although in nodal regions cholinergic innervation may be elevated.Citation308 Sympathetic hyperinnervation does occur in ventricles from diabetic rabbits after myocardial infarction; however the extent of hyperinnervation is not as great as in non-diabetic animals.Citation256 Autonomic dysfunction is also a well-known complication of obesity. Overactivity of the sympathetic nervous system, including that to the heart,Citation309-Citation316 and attenuated parasympathetic vagal toneCitation317-Citation320 is present in obese individuals, even when normotensive. Recently, we have shown sympathetic hyperinnervation in ventricles (McCully B.H., Hasan W., Streiff C.T., Houle J., Woodward W.R., Giraud G.D., et al.; unpublished data) and parasympathetic and sympathetic hyperinnervation in atria (Moses M., Macek A., Hasan W.; unpublished data) from obese rats; these rats have an increased risk of inducible tachyarrhythmias suggesting that structural remodeling of autonomic nerves is contributory to increased arrhythmia incidence in obesity. What may be extrapolated from these studies is that heterogeneous autonomic innervation is likely causal in increased incidence of sudden cardiac death brought on by either atrial or ventricular arrhythmias in a number of cardiovascular pathologies.
Parasympathetic plasticity in the adult heart and therapeutic vagal stimulation
The sympathetic dysfunction in heart failure is accompanied by attenuated baroreceptor-mediated bradycardia and hence reduced parasympathetic function.Citation321-Citation323 As mentioned earlier, attenuated parasympathetic function has direct consequences for norepinephrine release; withdrawal of parasympathetic inhibition of NE release contributes to greater NE spillover in cardiac tissue as compared with other vascular beds.Citation275,Citation280 Indeed, reduced vagal activity has a strong association with mortality in humans, including for arrhythmia generation and heart failure.Citation321,Citation323-Citation328 In mice with reduced cardiac acetylcholine release, depressed left ventricle function ultimately progresses to heart failure showing clearly the link between cardiac nerve activity and heart failure development.Citation329 However, in the latter study, it is likely that in addition to the postganglionic cholinergic nerves, both preganglionic sympathetic and parasympathetic terminals were also affected. In contrast, vagal nerve stimulation can be protective against ventricular fibrillation, in canines following myocardial ischemia, or in rats undergoing heart failure.Citation330-Citation334 Indeed, in a recent small clinical trial, chronic vagal nerve stimulation was shown to be safe and efficacious for patients with heart failure, patients showing improved left ventricular function.Citation335,Citation336
Although vagal stimulation has had some success in experimental and clinical models, whether alterations occur in postganglionic parasympathetic nerves in cardiac pathologies has received scant attention. Some limited evidence exists suggesting that neurochemical changes occur in the postganglionic neurons. A decrease in acetylcholinesterase, the enzyme responsible for acetylcholine breakdown, has been reported in the sino-atrial node of canines with heart failure.Citation337 Acetylcholine and its esterase expression are usually regulated in parallel,Citation337 suggesting reduced cholinergic neurotransmission in heart failure. Indeed, cholinergic stimulation with pyridostigmine (cholinesterase inhibitor) reduces ventricular arrhythmia and enhances heart rate variability in patients with heart failure.Citation338 Nitric oxide generated at parasympathetic nerve terminals is believed to facilitate acetylcholine releaseCitation339; in heart failure rats with diminished vagal function, decreased neuronal nitric oxide synthase expression has been reported in the right atriaCitation340 consistent with reduced nitergic, and consequent cholinergic discharge, at parasympathetic terminals. After explant culture, cardiac ganglion neurons increase expression of NPY and pituitary adenylate cyclase-activating polypeptide (PACAP) possibly due to loss of an inhibitory target-derived neurturin effect, demonstrating some neurochemical plasticity in adulthood.Citation341,Citation342 Peptides such as PACAP can also enhance the excitability of cardiac ganglion neurons suggesting a possible preganglionic role for PACAP.Citation343 Some level of plasticity is also suggested by the findings of reduced nicotinic sensitivity of cardiac ganglion interneurons in heart failure.Citation344 Changes in postganglionic cholinergic neuron neurotransmitter synthesis or release, alterations in trophic peptides or proteins, and an alteration in the preganglionic input, may contribute to increased plasticity of parasympathetic neurons in cardiac disease progression.
Parasympathetic plasticity; role of sympathetic neurotransmitters and peptides
Sympathetic nerves can also influence the neurochemical properties of adjacent parasympathetic neurons. In orbital smooth muscle for example, loss of co-projecting sympathetic neurons results in upregulation of catecholaminergic traits in cranial parasympathetic neurons.Citation197,Citation345 Neurotransmission is also altered in these parasympathetic neurons, from pre-junctional inhibition of sympathetic neurotransmission, to forming of stimulatory neuroeffector contacts. Whether similar neuroplasticity occurs in cardiac parasympathetic nerves has not been extensively examined. We have recently identified a reduction in cholinergic traits within adult rat cardiac ganglion neurons following either surgical or chemical sympathectomy,Citation199 or indeed in heart failure (Hasan W., Smith P.G.; unpublished data). Sympathetic neurons therefore intimately regulate the phenotype of cardiac ganglion neurons. Our sympathectomy studies suggest that both intrinsic and extrinsic adrenergic cardiac sources regulate the cholinergic phenotype. In this context, there is some controversy in the literature about the well-documented presence of adrenergic markers within cardiac ganglion neurons. Cardiac ganglion neurons possess a number of catecholaminergic traits including tyrosine hydroxylase, dopamine β-hydroxylase, norepinephrine transporter and vesicular monoamine transporter-2.Citation189,Citation199,Citation346,Citation347 However, there is certainly some species-variability in neurochemistry, and the evidence for a complete adrenergic panel in one species is debatable. Based on these data, however, some investigators have suggested that a pure adrenergic population, or a dual cholinergic/adrenergic population, may reside with cell bodies actually present within the cardiac ganglia.Citation17,Citation348-Citation355 At least in the rat, we were able to definitively demonstrate that glyoxcylic acid histofluorescence for catecholamines was absent from large (20–40 μm diameter) cardiac ganglion neurons, but was present in small (8–10 μm) intensely fluorescent (SIF) cells.Citation199 It would certainly be physiologically significant if, in situations of cardiac pathology, parasympathetic neurons were able to functionally switch to a dual cholinergic/adrenergic or even a pure adrenergic phenotype. Parasympathetic neurons therefore retain plasticity in their neurochemical phenotype into adulthood and peptides, and factors released by sympathetic nerves, are intimately involved in modulating parasympathetic function.
Parasympathetic plasticity; role of neurotrophic factors
The identity of factors, released by sympathetic nerves, that can maintain parasympathetic cholinergic phenotype remain to be determined. As earlier examined for sympathetic neurons, the role of “traditional” target-derived neurotrophic factors is crucial in maintenance of parasympathetic neurochemistry and function. In adult rats, both ret and GFRα2 are expressed by over 90% of cardiac ganglion neurons.Citation187 Since GDNF can signal weakly through GFRα2181, it is possible that both GDNF and neurturin may play a role in maintenance of adult cardiac ganglion neurons. Although evidence for a role of GDNF and neurturin in modulating neurochemistry within parasympathetic neurons is limited, these proteins can promote expression of the cholinergic phenotype in sympathetic neurons. Similarly, various neurotrophins have been implicated as inducers and maintainers of the cholinergic function in a number of neuronal populations including those derived from the neural crest.Citation356-Citation360 In embryonic sympathetic neurons, cholinergic differentiation is triggered by a synexpression group of neurotrophins including CNTF, NT-3 and GDNF, whereas NGF maintains the catecholaminergic phenotype.Citation361,Citation362 Studies on bimodal neonatal sympathetic neurons that maintain dual neurotransmitter status (adrenergic and cholinergic) in co-culture with cardiac myocytes,Citation363 have suggested that BDNF, acting via the pan-neurotrophin p75 receptor, induces a rapid switch in transmission toward acetylcholine release.Citation358,Citation364,Citation365 In contrast, CNTF promotes a more gradual increase in cholinergic markers within these bimodal sympathetic neurons.Citation358 Further, ret signaling is necessary for the maturation of cholinergic traits within sympathetic neurons.Citation366 A number of neurotrophic proteins can therefore promote a cholinergic phenotype; however the relative significance of these trophic proteins for cardiac ganglion neurochemistry and function has not been extensively examined.
Conclusion
There is strong evidence to suggest that developmental programs that govern autonomic nerve differentiation, survival and nerve patterning can be re-activated in mature neurons in situations of cardiac pathology. The crosstalk between both limbs of the autonomic nervous system is critical for maintenance of normal cardiac rhythm and function. Examination of the mechanisms involved in development of these intimate connections will potentially allow therapeutic approaches to be harnessed for reversing breakdown in these communications in diseased states.
Acknowledgments
The author wishes to thank Drs. Alison Ting (Oregon National Primate Center) and Sonnet Jonker (Oregon Health and Science University) for thoughtful insights during the preparation of this manuscript. Funding from NIH (NS077063) and the Knight Cardiovascular Institute supports research in the author’s laboratory.
Disclosure of Potential Conflicts of Interest
No potential conflicts of interest were disclosed.
References
- Westfall TC. Local regulation of adrenergic neurotransmission. Physiol Rev 1977; 57:659 - 728; PMID: 198824
- Smolen AJ. Morphology of synapses in the autonomic nervous system. J Electron Microsc Tech 1988; 10:187 - 204; http://dx.doi.org/10.1002/jemt.1060100205; PMID: 3068334
- Levy MN. Autonomic interactions in cardiac control. Ann N Y Acad Sci 1990; 601:209 - 21; http://dx.doi.org/10.1111/j.1749-6632.1990.tb37302.x; PMID: 2221687
- Ito M, Zipes DP. Efferent sympathetic and vagal innervation of the canine right ventricle. Circulation 1994; 90:1459 - 68; http://dx.doi.org/10.1161/01.CIR.90.3.1459; PMID: 8087953
- Crick SJ, Anderson RH, Ho SY, Sheppard MN. Localisation and quantitation of autonomic innervation in the porcine heart II: endocardium, myocardium and epicardium. J Anat 1999; 195:359 - 73; http://dx.doi.org/10.1046/j.1469-7580.1999.19530359.x; PMID: 10580851
- Crick SJ, Wharton J, Sheppard MN, Royston D, Yacoub MH, Anderson RH, et al. Innervation of the human cardiac conduction system. A quantitative immunohistochemical and histochemical study. Circulation 1994; 89:1697 - 708; http://dx.doi.org/10.1161/01.CIR.89.4.1697; PMID: 7908612
- Crick SJ, Sheppard MN, Ho SY, Anderson RH. Localisation and quantitation of autonomic innervation in the porcine heart I: conduction system. J Anat 1999; 195:341 - 57; http://dx.doi.org/10.1046/j.1469-7580.1999.19530341.x; PMID: 10580850
- Chow LT, Chow SS, Anderson RH, Gosling JA. Innervation of the human cardiac conduction system at birth. Br Heart J 1993; 69:430 - 5; http://dx.doi.org/10.1136/hrt.69.5.430; PMID: 7686024
- Pardini BJ, Lund DD, Schmid PG. Organization of the sympathetic postganglionic innervation of the rat heart. J Auton Nerv Syst 1989; 28:193 - 201; http://dx.doi.org/10.1016/0165-1838(89)90146-X; PMID: 2628461
- Pardini BJ, Lund DD, Schmid PG. Innervation patterns of the middle cervical--stellate ganglion complex in the rat. Neurosci Lett 1990; 117:300 - 6; http://dx.doi.org/10.1016/0304-3940(90)90681-X; PMID: 2128849
- Protas L, Qu J, Robinson RB. Neuropeptide y: neurotransmitter or trophic factor in the heart?. News Physiol Sci 2003; 18:181 - 5; PMID: 14500795
- Ernsberger U. The development of postganglionic sympathetic neurons: coordinating neuronal differentiation and diversification. Auton Neurosci 2001; 94:1 - 13; http://dx.doi.org/10.1016/S1566-0702(01)00336-8; PMID: 11775697
- Yang T, Levy MN. Sequence of excitation as a factor in sympathetic-parasympathetic interactions in the heart. Circ Res 1992; 71:898 - 905; http://dx.doi.org/10.1161/01.RES.71.4.898; PMID: 1516162
- Warner MR, Levy MN. Neuropeptide Y as a putative modulator of the vagal effects on heart rate. Circ Res 1989; 64:882 - 9; http://dx.doi.org/10.1161/01.RES.64.5.882; PMID: 2706760
- Herring N, Cranley J, Lokale MN, Li D, Shanks J, Alston EN, et al. The cardiac sympathetic co-transmitter galanin reduces acetylcholine release and vagal bradycardia: implications for neural control of cardiac excitability. J Mol Cell Cardiol 2012; 52:667 - 76; http://dx.doi.org/10.1016/j.yjmcc.2011.11.016; PMID: 22172449
- Herring N, Lokale MN, Danson EJ, Heaton DA, Paterson DJ. Neuropeptide Y reduces acetylcholine release and vagal bradycardia via a Y2 receptor-mediated, protein kinase C-dependent pathway. J Mol Cell Cardiol 2008; 44:477 - 85; http://dx.doi.org/10.1016/j.yjmcc.2007.10.001; PMID: 17996892
- Singh S, Johnson PI, Javed A, Gray TS, Lonchyna VA, Wurster RD. Monoamine- and histamine-synthesizing enzymes and neurotransmitters within neurons of adult human cardiac ganglia. Circulation 1999; 99:411 - 9; http://dx.doi.org/10.1161/01.CIR.99.3.411; PMID: 9918529
- Ardell JL, Randall WC. Selective vagal innervation of sinoatrial and atrioventricular nodes in canine heart. Am J Physiol 1986; 251:H764 - 73; PMID: 3021001
- Randall WC, Milosavljevic M, Wurster RD, Geis GS, Ardell JL. Selective vagal innervation of the heart. Ann Clin Lab Sci 1986; 16:198 - 208; PMID: 2872849
- Singh S, Johnson PI, Lee RE, Orfei E, Lonchyna VA, Sullivan HJ, et al. Topography of cardiac ganglia in the adult human heart. J Thorac Cardiovasc Surg 1996; 112:943 - 53; http://dx.doi.org/10.1016/S0022-5223(96)70094-6; PMID: 8873720
- Lachman N, Syed FF, Habib A, Kapa S, Bisco SE, Venkatachalam KL, et al. Correlative anatomy for the electrophysiologist, part II: cardiac ganglia, phrenic nerve, coronary venous system. J Cardiovasc Electrophysiol 2011; 22:104 - 10; http://dx.doi.org/10.1111/j.1540-8167.2010.01882.x; PMID: 20807274
- Kapa S, Venkatachalam KL, Asirvatham SJ. The autonomic nervous system in cardiac electrophysiology: an elegant interaction and emerging concepts. Cardiol Rev 2010; 18:275 - 84; http://dx.doi.org/10.1097/CRD.0b013e3181ebb152; PMID: 20926936
- Conlon K, Collins T, Kidd C. Modulation of vagal actions on heart rate produced by inhibition of nitric oxide synthase in the anaesthetized ferret. Exp Physiol 1996; 81:547 - 50; PMID: 8737087
- Herring N, Zaman JA, Paterson DJ. Natriuretic peptides like NO facilitate cardiac vagal neurotransmission and bradycardia via a cGMP pathway. Am J Physiol Heart Circ Physiol 2001; 281:H2318 - 27; PMID: 11709398
- Henning RJ, Sawmiller DR. Vasoactive intestinal peptide: cardiovascular effects. Cardiovasc Res 2001; 49:27 - 37; http://dx.doi.org/10.1016/S0008-6363(00)00229-7; PMID: 11121793
- Vanhoutte PM, Levy MN. Prejunctional cholinergic modulation of adrenergic neurotransmission in the cardiovascular system. Am J Physiol 1980; 238:H275 - 81; PMID: 6245589
- Manabe N, Foldes FF, Töröcsik A, Nagashima H, Goldiner PL, Vizi ES. Presynaptic interaction between vagal and sympathetic innervation in the heart: modulation of acetylcholine and noradrenaline release. J Auton Nerv Syst 1991; 32:233 - 42; http://dx.doi.org/10.1016/0165-1838(91)90117-L; PMID: 1645381
- Löffelholz K, Pappano AJ. The parasympathetic neuroeffector junction of the heart. Pharmacol Rev 1985; 37:1 - 24; PMID: 2408285
- Pappano AJ. Development of cholinergic neuroeffector transmission in the avian heart. Implications for regulatory mechanisms. Ann N Y Acad Sci 1990; 588:131 - 6; http://dx.doi.org/10.1111/j.1749-6632.1990.tb13203.x; PMID: 2162649
- Ehinger B, Falck B, Sporrong B. Possible axo-axonal synapses between peripheral adrenergic and cholinergic nerve terminals. Z Zellforsch Mikrosk Anat 1970; 107:508 - 21; http://dx.doi.org/10.1007/BF00335438; PMID: 5448481
- Choate JK, Klemm M, Hirst GD. Sympathetic and parasympathetic neuromuscular junctions in the guinea-pig sino-atrial node. J Auton Nerv Syst 1993; 44:1 - 15; http://dx.doi.org/10.1016/0165-1838(93)90374-4; PMID: 8409214
- Parsons RL. Mammalian cardiac ganglia as local integration centers: histochemical and electrophysiological evidence. In: Dun NJ, ed. Neural Mechanisms of Cardiovascular Regulation. Boston: Kluwer Academic Publishers; 2004:335-6.
- Armour JA. Cardiac neuronal hierarchy in health and disease. Am J Physiol Regul Integr Comp Physiol 2004; 287:R262 - 71; http://dx.doi.org/10.1152/ajpregu.00183.2004; PMID: 15271675
- Ardell JL. Neurohumoral control of cardiac fauntion. In: Sperelakis N, ed. Heart physiology and pathophysiology. San Diego: Academic Press; 2001:45-59.
- Randall DC, Brown DR, McGuirt AS, Thompson GW, Armour JA, Ardell JL. Interactions within the intrinsic cardiac nervous system contribute to chronotropic regulation. Am J Physiol Regul Integr Comp Physiol 2003; 285:R1066 - 75; PMID: 12842863
- Waldmann M, Thompson GW, Kember GC, Ardell JL, Armour JA. Stochastic behavior of atrial and ventricular intrinsic cardiac neurons. J Appl Physiol 2006; 101:413 - 9; http://dx.doi.org/10.1152/japplphysiol.01346.2005; PMID: 16645188
- Thompson GW, Collier K, Ardell JL, Kember G, Armour JA. Functional interdependence of neurons in a single canine intrinsic cardiac ganglionated plexus. J Physiol 2000; 528:561 - 71; http://dx.doi.org/10.1111/j.1469-7793.2000.00561.x; PMID: 11060132
- Olshansky B. Interrelationships between the autonomic nervous system and atrial fibrillation. Prog Cardiovasc Dis 2005; 48:57 - 78; http://dx.doi.org/10.1016/j.pcad.2005.06.004; PMID: 16194692
- Armour JA. Intrinsic cardiac neurons involved in cardiac regulation possess alpha 1-, alpha 2-, beta 1- and beta 2-adrenoceptors. Can J Cardiol 1997; 13:277 - 84; PMID: 9117916
- Yiallourou SR, Sands SA, Walker AM, Horne RS. Maturation of heart rate and blood pressure variability during sleep in term-born infants. Sleep 2012; 35:177 - 86; PMID: 22294807
- Yiallourou SR, Witcombe NB, Sands SA, Walker AM, Horne RS. The development of autonomic cardiovascular control is altered by preterm birth. Early Hum Dev 2013; 89:145 - 52; http://dx.doi.org/10.1016/j.earlhumdev.2012.09.009; PMID: 23058299
- Ledwidge M, Fox G, Matthews T. Neurocardiogenic syncope: a model for SIDS. Arch Dis Child 1998; 78:481 - 3; http://dx.doi.org/10.1136/adc.78.5.481; PMID: 9659101
- Crump C, Winkleby MA, Sundquist K, Sundquist J. Risk of hypertension among young adults who were born preterm: a Swedish national study of 636,000 births. Am J Epidemiol 2011; 173:797 - 803; http://dx.doi.org/10.1093/aje/kwq440; PMID: 21320866
- Barker DJ, Eriksson JG, Forsén T, Osmond C. Fetal origins of adult disease: strength of effects and biological basis. Int J Epidemiol 2002; 31:1235 - 9; http://dx.doi.org/10.1093/ije/31.6.1235; PMID: 12540728
- Barker DJ. Fetal programming of coronary heart disease. Trends Endocrinol Metab 2002; 13:364 - 8; http://dx.doi.org/10.1016/S1043-2760(02)00689-6; PMID: 12367816
- Leon DA, Lithell HO, Vâgerö D, Koupilová I, Mohsen R, Berglund L, et al. Reduced fetal growth rate and increased risk of death from ischaemic heart disease: cohort study of 15 000 Swedish men and women born 1915-29. BMJ 1998; 317:241 - 5; http://dx.doi.org/10.1136/bmj.317.7153.241; PMID: 9677213
- Alkon A, Boyce WT, Davis NV, Eskenazi B. Developmental changes in autonomic nervous system resting and reactivity measures in Latino children from 6 to 60 months of age. J Dev Behav Pediatr 2011; 32:668 - 77; http://dx.doi.org/10.1097/DBP.0b013e3182331fa6; PMID: 22008788
- Treadwell MJ, Alkon A, Styles L, Boyce WT. Autonomic nervous system reactivity: children with and without sickle cell disease. Nurs Res 2011; 60:197 - 207; http://dx.doi.org/10.1097/NNR.0b013e3182186a21; PMID: 21532352
- Mizuno M, Siddique K, Baum M, Smith SA. Prenatal programming of hypertension induces sympathetic overactivity in response to physical stress. Hypertension 2013; 61:180 - 6; http://dx.doi.org/10.1161/HYPERTENSIONAHA.112.199356; PMID: 23150514
- Samuelsson AM, Morris A, Igosheva N, Kirk SL, Pombo JM, Coen CW, et al. Evidence for sympathetic origins of hypertension in juvenile offspring of obese rats. Hypertension 2010; 55:76 - 82; http://dx.doi.org/10.1161/HYPERTENSIONAHA.109.139402; PMID: 19901159
- Tucker DC, Johnson AK. Development of autonomic control of heart rate in genetically hypertensive and normotensive rats. Am J Physiol 1984; 246:R570 - 7; PMID: 6144273
- Moore KL, Persaud TVN. The Developing Human: Clinically Oriented Embryology. 8th ed. Elsevier, 2007.
- Huber K. The sympathoadrenal cell lineage: specification, diversification, and new perspectives. Dev Biol 2006; 298:335 - 43; http://dx.doi.org/10.1016/j.ydbio.2006.07.010; PMID: 16928368
- Luther JA, Birren SJ. Neurotrophins and target interactions in the development and regulation of sympathetic neuron electrical and synaptic properties. Auton Neurosci 2009; 151:46 - 60; http://dx.doi.org/10.1016/j.autneu.2009.08.009; PMID: 19748836
- Davies AM. Extracellular signals regulating sympathetic neuron survival and target innervation during development. Auton Neurosci 2009; 151:39 - 45; http://dx.doi.org/10.1016/j.autneu.2009.07.011; PMID: 19660992
- Rohrer H. Transcriptional control of differentiation and neurogenesis in autonomic ganglia. Eur J Neurosci 2011; 34:1563 - 73; http://dx.doi.org/10.1111/j.1460-9568.2011.07860.x; PMID: 22103414
- Jiang M, Stanke J, Lahti JM. The connections between neural crest development and neuroblastoma. Curr Top Dev Biol 2011; 94:77 - 127; http://dx.doi.org/10.1016/B978-0-12-380916-2.00004-8; PMID: 21295685
- Stewart RA, Lee JS, Lachnit M, Look AT, Kanki JP, Henion PD. Studying peripheral sympathetic nervous system development and neuroblastoma in zebrafish. Methods Cell Biol 2010; 100:127 - 52; http://dx.doi.org/10.1016/B978-0-12-384892-5.00005-0; PMID: 21111216
- Vincentz JW, Rubart M, Firulli AB. Ontogeny of cardiac sympathetic innervation and its implications for cardiac disease. Pediatr Cardiol 2012; 33:923 - 8; http://dx.doi.org/10.1007/s00246-012-0248-1; PMID: 22395650
- Kirby ML, Hutson MR. Factors controlling cardiac neural crest cell migration. Cell Adh Migr 2010; 4:609 - 21; http://dx.doi.org/10.4161/cam.4.4.13489; PMID: 20890117
- Young HM, Cane KN, Anderson CR. Development of the autonomic nervous system: a comparative view. Auton Neurosci 2011; 165:10 - 27; http://dx.doi.org/10.1016/j.autneu.2010.03.002; PMID: 20346736
- Cane KN, Anderson CR. Generating diversity: Mechanisms regulating the differentiation of autonomic neuron phenotypes. Auton Neurosci 2009; 151:17 - 29; http://dx.doi.org/10.1016/j.autneu.2009.08.010; PMID: 19819195
- Hildreth V, Anderson RH, Henderson DJ. Autonomic innervation of the developing heart: origins and function. Clin Anat 2009; 22:36 - 46; http://dx.doi.org/10.1002/ca.20695; PMID: 18846544
- Kawashima T. The autonomic nervous system of the human heart with special reference to its origin, course, and peripheral distribution. Anat Embryol (Berl) 2005; 209:425 - 38; http://dx.doi.org/10.1007/s00429-005-0462-1; PMID: 15887046
- Kimura K, Ieda M, Fukuda K. Development, maturation, and transdifferentiation of cardiac sympathetic nerves. Circ Res 2012; 110:325 - 36; http://dx.doi.org/10.1161/CIRCRESAHA.111.257253; PMID: 22267838
- Kasemeier-Kulesa JC, Kulesa PM, Lefcort F. Imaging neural crest cell dynamics during formation of dorsal root ganglia and sympathetic ganglia. Development 2005; 132:235 - 45; http://dx.doi.org/10.1242/dev.01553; PMID: 15590743
- Lallier TE, Bronner-Fraser M. A spatial and temporal analysis of dorsal root and sympathetic ganglion formation in the avian embryo. Dev Biol 1988; 127:99 - 112; http://dx.doi.org/10.1016/0012-1606(88)90192-3; PMID: 3282939
- Loring JF, Erickson CA. Neural crest cell migratory pathways in the trunk of the chick embryo. Dev Biol 1987; 121:220 - 36; http://dx.doi.org/10.1016/0012-1606(87)90154-0; PMID: 3552788
- Kasemeier-Kulesa JC, Bradley R, Pasquale EB, Lefcort F, Kulesa PM. Eph/ephrins and N-cadherin coordinate to control the pattern of sympathetic ganglia. Development 2006; 133:4839 - 47; http://dx.doi.org/10.1242/dev.02662; PMID: 17108003
- Krull CE, Lansford R, Gale NW, Collazo A, Marcelle C, Yancopoulos GD, et al. Interactions of Eph-related receptors and ligands confer rostrocaudal pattern to trunk neural crest migration. Curr Biol 1997; 7:571 - 80; http://dx.doi.org/10.1016/S0960-9822(06)00256-9; PMID: 9259560
- Wang HU, Anderson DJ. Eph family transmembrane ligands can mediate repulsive guidance of trunk neural crest migration and motor axon outgrowth. Neuron 1997; 18:383 - 96; http://dx.doi.org/10.1016/S0896-6273(00)81240-4; PMID: 9115733
- Henion PD, Weston JA. Timing and pattern of cell fate restrictions in the neural crest lineage. Development 1997; 124:4351 - 9; PMID: 9334283
- Rubin E. Development of the rat superior cervical ganglion: ganglion cell maturation. J Neurosci 1985; 5:673 - 84; PMID: 2983044
- Purves D, Rubin E, Snider WD, Lichtman J. Relation of animal size to convergence, divergence, and neuronal number in peripheral sympathetic pathways. J Neurosci 1986; 6:158 - 63; PMID: 3944617
- Gonsalvez DG, Cane KN, Landman KA, Enomoto H, Young HM, Anderson CR. Proliferation and cell cycle dynamics in the developing stellate ganglion. J Neurosci 2013; 33:5969 - 79; http://dx.doi.org/10.1523/JNEUROSCI.4350-12.2013; PMID: 23554478
- Honma Y, Araki T, Gianino S, Bruce A, Heuckeroth R, Johnson E, et al. Artemin is a vascular-derived neurotropic factor for developing sympathetic neurons. Neuron 2002; 35:267 - 82; http://dx.doi.org/10.1016/S0896-6273(02)00774-2; PMID: 12160745
- Enomoto H, Crawford PA, Gorodinsky A, Heuckeroth RO, Johnson EM Jr., Milbrandt J. RET signaling is essential for migration, axonal growth and axon guidance of developing sympathetic neurons. Development 2001; 128:3963 - 74; PMID: 11641220
- Young HM, Anderson RB, Anderson CR. Guidance cues involved in the development of the peripheral autonomic nervous system. Auton Neurosci 2004; 112:1 - 14; http://dx.doi.org/10.1016/j.autneu.2004.02.008; PMID: 15233925
- Schwarz Q, Maden CH, Vieira JM, Ruhrberg C. Neuropilin 1 signaling guides neural crest cells to coordinate pathway choice with cell specification. Proc Natl Acad Sci U S A 2009; 106:6164 - 9; http://dx.doi.org/10.1073/pnas.0811521106; PMID: 19325129
- Fantin A, Maden CH, Ruhrberg C. Neuropilin ligands in vascular and neuronal patterning. Biochem Soc Trans 2009; 37:1228 - 32; http://dx.doi.org/10.1042/BST0371228; PMID: 19909252
- Ieda M, Kanazawa H, Kimura K, Hattori F, Ieda Y, Taniguchi M, et al. Sema3a maintains normal heart rhythm through sympathetic innervation patterning. Nat Med 2007; 13:604 - 12; http://dx.doi.org/10.1038/nm1570; PMID: 17417650
- Tillo M, Ruhrberg C, Mackenzie F. Emerging roles for semaphorins and VEGFs in synaptogenesis and synaptic plasticity. Cell Adh Migr 2012; 6:541 - 6; http://dx.doi.org/10.4161/cam.22408; PMID: 23076132
- Maden CH, Gomes J, Schwarz Q, Davidson K, Tinker A, Ruhrberg C. NRP1 and NRP2 cooperate to regulate gangliogenesis, axon guidance and target innervation in the sympathetic nervous system. Dev Biol 2012; 369:277 - 85; http://dx.doi.org/10.1016/j.ydbio.2012.06.026; PMID: 22790009
- Kawano H, Okada R, Yano K. Histological study on the distribution of autonomic nerves in the human heart. Heart Vessels 2003; 18:32 - 9; http://dx.doi.org/10.1007/s003800300005; PMID: 12644879
- Waimey KE, Huang PH, Chen M, Cheng HJ. Plexin-A3 and plexin-A4 restrict the migration of sympathetic neurons but not their neural crest precursors. Dev Biol 2008; 315:448 - 58; http://dx.doi.org/10.1016/j.ydbio.2008.01.002; PMID: 18262512
- Chan WY, Cheung CS, Yung KM, Copp AJ. Cardiac neural crest of the mouse embryo: axial level of origin, migratory pathway and cell autonomy of the splotch (Sp2H) mutant effect. Development 2004; 131:3367 - 79; http://dx.doi.org/10.1242/dev.01197; PMID: 15226254
- Verberne ME, Gittenberger-de Groot AC, van Iperen L, Poelmann RE. Distribution of different regions of cardiac neural crest in the extrinsic and the intrinsic cardiac nervous system. Dev Dyn 2000; 217:191 - 204; http://dx.doi.org/10.1002/(SICI)1097-0177(200002)217:2<191::AID-DVDY6>3.0.CO;2-X; PMID: 10706143
- Hildreth V, Webb S, Bradshaw L, Brown NA, Anderson RH, Henderson DJ. Cells migrating from the neural crest contribute to the innervation of the venous pole of the heart. J Anat 2008; 212:1 - 11; PMID: 18031480
- Pappano AJ. Ontogenetic development of autonomic neuroeffector transmission and transmitter reactivity in embryonic and fetal hearts. Pharmacol Rev 1977; 29:3 - 33; PMID: 22089
- Kirby ML, McKenzie JW, Weidman TA. Developing innervation of the chick heart: a histofluorescence and light microscopic study of sympthetic innervation. Anat Rec 1980; 196:333 - 40; http://dx.doi.org/10.1002/ar.1091960309; PMID: 7406224
- Kirby ML, Weidman TA, McKenzie JW. An ultrastructural study of the cardia ganglia in the bulbar plexus of the developing chick heart. Dev Neurosci 1980; 3:174 - 84; http://dx.doi.org/10.1159/000112390; PMID: 7460790
- O’Rahilly R, Müller F. The development of the neural crest in the human. J Anat 2007; 211:335 - 51; http://dx.doi.org/10.1111/j.1469-7580.2007.00773.x; PMID: 17848161
- Kirby ML, Gale TF, Stewart DE. Neural crest cells contribute to normal aorticopulmonary septation. Science 1983; 220:1059 - 61; http://dx.doi.org/10.1126/science.6844926; PMID: 6844926
- Kirby ML, Stewart DE. Neural crest origin of cardiac ganglion cells in the chick embryo: identification and extirpation. Dev Biol 1983; 97:433 - 43; http://dx.doi.org/10.1016/0012-1606(83)90100-8; PMID: 6852374
- Brown CB, Feiner L, Lu MM, Li J, Ma X, Webber AL, et al. PlexinA2 and semaphorin signaling during cardiac neural crest development. Development 2001; 128:3071 - 80; PMID: 11688557
- Verberne ME, Gittenberger-De Groot AC, Poelmann RE. Distribution of antigen epitopes shared by nerves and the myocardium of the embryonic chick heart using different neuronal markers. Anat Rec 2000; 260:335 - 50; http://dx.doi.org/10.1002/1097-0185(200012)260:4<334::AID-AR20>3.0.CO;2-Y; PMID: 11074398
- Schneider C, Wicht H, Enderich J, Wegner M, Rohrer H. Bone morphogenetic proteins are required in vivo for the generation of sympathetic neurons. Neuron 1999; 24:861 - 70; http://dx.doi.org/10.1016/S0896-6273(00)81033-8; PMID: 10624949
- Shah NM, Groves AK, Anderson DJ. Alternative neural crest cell fates are instructively promoted by TGFbeta superfamily members. Cell 1996; 85:331 - 43; http://dx.doi.org/10.1016/S0092-8674(00)81112-5; PMID: 8616889
- Morikawa Y, Zehir A, Maska E, Deng C, Schneider MD, Mishina Y, et al. BMP signaling regulates sympathetic nervous system development through Smad4-dependent and -independent pathways. Development 2009; 136:3575 - 84; http://dx.doi.org/10.1242/dev.038133; PMID: 19793887
- Pattyn A, Morin X, Cremer H, Goridis C, Brunet JF. The homeobox gene Phox2b is essential for the development of autonomic neural crest derivatives. Nature 1999; 399:366 - 70; http://dx.doi.org/10.1038/20700; PMID: 10360575
- Guillemot F, Lo LC, Johnson JE, Auerbach A, Anderson DJ, Joyner AL. Mammalian achaete-scute homolog 1 is required for the early development of olfactory and autonomic neurons. Cell 1993; 75:463 - 76; http://dx.doi.org/10.1016/0092-8674(93)90381-Y; PMID: 8221886
- Pattyn A, Guillemot F, Brunet JF. Delays in neuronal differentiation in Mash1/Ascl1 mutants. Dev Biol 2006; 295:67 - 75; http://dx.doi.org/10.1016/j.ydbio.2006.03.008; PMID: 16677628
- Hirsch MR, Tiveron MC, Guillemot F, Brunet JF, Goridis C. Control of noradrenergic differentiation and Phox2a expression by MASH1 in the central and peripheral nervous system. Development 1998; 125:599 - 608; PMID: 9435281
- Yang C, Kim HS, Seo H, Kim CH, Brunet JF, Kim KS. Paired-like homeodomain proteins, Phox2a and Phox2b, are responsible for noradrenergic cell-specific transcription of the dopamine beta-hydroxylase gene. J Neurochem 1998; 71:1813 - 26; http://dx.doi.org/10.1046/j.1471-4159.1998.71051813.x; PMID: 9798905
- Chen S, Ji M, Paris M, Hullinger RL, Andrisani OM. The cAMP pathway regulates both transcription and activity of the paired homeobox transcription factor Phox2a required for development of neural crest-derived and central nervous system-derived catecholaminergic neurons. J Biol Chem 2005; 280:41025 - 36; http://dx.doi.org/10.1074/jbc.M503537200; PMID: 16204240
- Lim KC, Lakshmanan G, Crawford SE, Gu Y, Grosveld F, Engel JD. Gata3 loss leads to embryonic lethality due to noradrenaline deficiency of the sympathetic nervous system. Nat Genet 2000; 25:209 - 12; http://dx.doi.org/10.1038/76080; PMID: 10835639
- Tsarovina K, Pattyn A, Stubbusch J, Müller F, van der Wees J, Schneider C, et al. Essential role of Gata transcription factors in sympathetic neuron development. Development 2004; 131:4775 - 86; http://dx.doi.org/10.1242/dev.01370; PMID: 15329349
- Moriguchi T, Takako N, Hamada M, Maeda A, Fujioka Y, Kuroha T, et al. Gata3 participates in a complex transcriptional feedback network to regulate sympathoadrenal differentiation. Development 2006; 133:3871 - 81; http://dx.doi.org/10.1242/dev.02553; PMID: 16943277
- Müller F, Rohrer H. Molecular control of ciliary neuron development: BMPs and downstream transcriptional control in the parasympathetic lineage. Development 2002; 129:5707 - 17; http://dx.doi.org/10.1242/dev.00165; PMID: 12421710
- Lucas ME, Müller F, Rüdiger R, Henion PD, Rohrer H. The bHLH transcription factor hand2 is essential for noradrenergic differentiation of sympathetic neurons. Development 2006; 133:4015 - 24; http://dx.doi.org/10.1242/dev.02574; PMID: 17008447
- Morikawa Y, D’Autréaux F, Gershon MD, Cserjesi P. Hand2 determines the noradrenergic phenotype in the mouse sympathetic nervous system. Dev Biol 2007; 307:114 - 26; http://dx.doi.org/10.1016/j.ydbio.2007.04.027; PMID: 17531968
- Hendershot TJ, Liu H, Clouthier DE, Shepherd IT, Coppola E, Studer M, et al. Conditional deletion of Hand2 reveals critical functions in neurogenesis and cell type-specific gene expression for development of neural crest-derived noradrenergic sympathetic ganglion neurons. Dev Biol 2008; 319:179 - 91; http://dx.doi.org/10.1016/j.ydbio.2008.03.036; PMID: 18501887
- Vincentz JW, VanDusen NJ, Fleming AB, Rubart M, Firulli BA, Howard MJ, et al. A Phox2- and Hand2-dependent Hand1 cis-regulatory element reveals a unique gene dosage requirement for Hand2 during sympathetic neurogenesis. J Neurosci 2012; 32:2110 - 20; http://dx.doi.org/10.1523/JNEUROSCI.3584-11.2012; PMID: 22323723
- Schmidt M, Lin S, Pape M, Ernsberger U, Stanke M, Kobayashi K, et al. The bHLH transcription factor Hand2 is essential for the maintenance of noradrenergic properties in differentiated sympathetic neurons. Dev Biol 2009; 329:191 - 200; http://dx.doi.org/10.1016/j.ydbio.2009.02.020; PMID: 19254708
- Kameda Y, Saitoh T, Nemoto N, Katoh T, Iseki S. Hes1 is required for the development of the superior cervical ganglion of sympathetic trunk and the carotid body. Dev Dyn 2012; 241:1289 - 300; http://dx.doi.org/10.1002/dvdy.23819; PMID: 22689348
- Zehir A, Hua LL, Maska EL, Morikawa Y, Cserjesi P. Dicer is required for survival of differentiating neural crest cells. Dev Biol 2010; 340:459 - 67; http://dx.doi.org/10.1016/j.ydbio.2010.01.039; PMID: 20144605
- Heermann S, Mätlik K, Hinz U, Fey J, Arumae U, Krieglstein K. Glia cell line-derived neurotrophic factor mediates survival of murine sympathetic precursors. J Neurosci Res 2013; 91:780 - 5; http://dx.doi.org/10.1002/jnr.23188; PMID: 23426908
- Groves AK, George KM, Tissier-Seta JP, Engel JD, Brunet JF, Anderson DJ. Differential regulation of transcription factor gene expression and phenotypic markers in developing sympathetic neurons. Development 1995; 121:887 - 901; PMID: 7720591
- Lee VM, Bronner-Fraser M, Baker CV. Restricted response of mesencephalic neural crest to sympathetic differentiation signals in the trunk. Dev Biol 2005; 278:175 - 92; http://dx.doi.org/10.1016/j.ydbio.2004.10.024; PMID: 15649470
- Schmidt M, Huber L, Majdazari A, Schütz G, Williams T, Rohrer H. The transcription factors AP-2β and AP-2α are required for survival of sympathetic progenitors and differentiated sympathetic neurons. Dev Biol 2011; 355:89 - 100; http://dx.doi.org/10.1016/j.ydbio.2011.04.011; PMID: 21539825
- Hong SJ, Lardaro T, Oh MS, Huh Y, Ding Y, Kang UJ, et al. Regulation of the noradrenaline neurotransmitter phenotype by the transcription factor AP-2beta. J Biol Chem 2008; 283:16860 - 7; http://dx.doi.org/10.1074/jbc.M709106200; PMID: 18424435
- Huber L, Ferdin M, Holzmann J, Stubbusch J, Rohrer H. HoxB8 in noradrenergic specification and differentiation of the autonomic nervous system. Dev Biol 2012; 363:219 - 33; http://dx.doi.org/10.1016/j.ydbio.2011.12.026; PMID: 22236961
- Morin X, Cremer H, Hirsch MR, Kapur RP, Goridis C, Brunet JF. Defects in sensory and autonomic ganglia and absence of locus coeruleus in mice deficient for the homeobox gene Phox2a. Neuron 1997; 18:411 - 23; http://dx.doi.org/10.1016/S0896-6273(00)81242-8; PMID: 9115735
- Lipp JA, Rudolph AM. Sympathetic nerve development in the rat and guinea-pig heart. Biol Neonate 1972; 21:76 - 82; http://dx.doi.org/10.1159/000240497; PMID: 4651153
- Lebowitz EA, Novick JS, Rudolph AM. Development of myocardial sympathetic innervation in the fetal lamb. Pediatr Res 1972; 6:887 - 93; http://dx.doi.org/10.1203/00006450-197212000-00006; PMID: 4643537
- Vapaavouri EK, Shinebourne EA, Williams RL, Heymann MA, Rudolph AM. Development of cardiovascular responses to autonomic blockade in intact fetal and neonatal lambs. Biol Neonate 1973; 22:177 - 88; http://dx.doi.org/10.1159/000240552; PMID: 4147780
- Walker AM, Cannata J, Dowling MH, Ritchie B, Maloney JE. Sympathetic and parasympathetic control of heart rate in unanaesthetized fetal and newborn lambs. Biol Neonate 1978; 33:135 - 43; http://dx.doi.org/10.1159/000241063; PMID: 28158
- Llanos AJ, Green JR, Creasy RK, Rudolph AM. Increased heart rate response to parasympathetic and beta adrenergic blockade in growth-retarded fetal lambs. Am J Obstet Gynecol 1980; 136:808 - 13; PMID: 7355968
- Macdonald AA, Llanos AJ, Heymann MA, Rudolph AM. Cardiovascular responsiveness of the pig fetus to autonomic blockade. Pflugers Arch 1981; 390:262 - 4; http://dx.doi.org/10.1007/BF00658273; PMID: 7196025
- Tucker DC. Components of functional sympathetic control of heart rate in neonatal rats. Am J Physiol 1985; 248:R601 - 10; PMID: 2859811
- Woods JR Jr., Dandavino A, Murayama K, Brinkman CR 3rd, Assali NS. Autonomic control of cardiovascular functions during neonatal development and in adult sheep. Circ Res 1977; 40:401 - 7; http://dx.doi.org/10.1161/01.RES.40.4.401; PMID: 14791
- Nuwayhid B, Brinkman CR 3rd, Su C, Bevan JA, Assali NS. Development of autonomic control of fetal circulation. Am J Physiol 1975; 228:337 - 44; PMID: 235216
- Tanaka H, Kasuya Y, Saito H, Shigenobu K. Organ culture of rat heart: maintained high sensitivity of fetal atria before innervation to norepinephrine. Can J Physiol Pharmacol 1988; 66:901 - 6; http://dx.doi.org/10.1139/y88-147; PMID: 3214802
- Shigenobu K, Tanaka H, Kasuya Y. Changes in sensitivity of rat heart to norepinephrine and isoproterenol during pre- and postnatal development and its relation to sympathetic innervation. Dev Pharmacol Ther 1988; 11:226 - 36; PMID: 3224536
- Thomas SA, Matsumoto AM, Palmiter RD. Noradrenaline is essential for mouse fetal development. Nature 1995; 374:643 - 6; http://dx.doi.org/10.1038/374643a0; PMID: 7715704
- Pfeifer K, Boe SP, Rong Q, Ebert SN. Generating mouse models for studying the function and fate of intrinsic cardiac adrenergic cells. Ann N Y Acad Sci 2004; 1018:418 - 23; http://dx.doi.org/10.1196/annals.1296.051; PMID: 15240397
- Ebert SN, Thompson RP. Embryonic epinephrine synthesis in the rat heart before innervation: association with pacemaking and conduction tissue development. Circ Res 2001; 88:117 - 24; http://dx.doi.org/10.1161/01.RES.88.1.117; PMID: 11139483
- Zhou QY, Quaife CJ, Palmiter RD. Targeted disruption of the tyrosine hydroxylase gene reveals that catecholamines are required for mouse fetal development. Nature 1995; 374:640 - 3; http://dx.doi.org/10.1038/374640a0; PMID: 7715703
- Ebert SN, Taylor DG. Catecholamines and development of cardiac pacemaking: an intrinsically intimate relationship. Cardiovasc Res 2006; 72:364 - 74; http://dx.doi.org/10.1016/j.cardiores.2006.08.013; PMID: 17022958
- Althurwi HN, Tse MM, Abdelhamid G, Zordoky BN, Hammock BD, El-Kadi AO. Soluble epoxide hydrolase inhibitor, TUPS, protects against isoprenaline-induced cardiac hypertrophy. Br J Pharmacol 2013; 168:1794 - 807; http://dx.doi.org/10.1111/bph.12066; PMID: 23176298
- Králová E, Mokrán T, Murín J, Stankovicová T. Electrocardiography in two models of isoproterenol-induced left ventricular remodeling. Physiol Res 2008; 57:Suppl 2 S83 - 9; PMID: 18373388
- Elayan HH, Kennedy BP, Ziegler MG. Cardiac atria and ventricles contain different inducible adrenaline synthesising enzymes. Cardiovasc Res 1990; 24:53 - 6; http://dx.doi.org/10.1093/cvr/24.1.53; PMID: 2328515
- Huang MH, Friend DS, Sunday ME, Singh K, Haley K, Austen KF, et al. An intrinsic adrenergic system in mammalian heart. J Clin Invest 1996; 98:1298 - 303; http://dx.doi.org/10.1172/JCI118916; PMID: 8823294
- Long CS, Kariya K, Karns L, Simpson PC. Sympathetic activity: modulator of myocardial hypertrophy. J Cardiovasc Pharmacol 1991; 17:Suppl 2 S20 - 4; http://dx.doi.org/10.1097/00005344-199117002-00005; PMID: 1715479
- Natarajan AR, Rong Q, Katchman AN, Ebert SN. Intrinsic cardiac catecholamines help maintain beating activity in neonatal rat cardiomyocyte cultures. Pediatr Res 2004; 56:411 - 7; http://dx.doi.org/10.1203/01.PDR.0000136279.80897.4C; PMID: 15333759
- Abboud FM. An intrinsic cardiac adrenergic system can regulate cardiac development and function. J Clin Invest 1996; 98:1275 - 6; http://dx.doi.org/10.1172/JCI118912; PMID: 8823290
- Murphy DA, O’Blenes S, Hanna BD, Armour JA. Capacity of intrinsic cardiac neurons to modify the acutely autotransplanted mammalian heart. J Heart Lung Transplant 1994; 13:847 - 56; PMID: 7803426
- Matthews MR. Small, intensely fluorescent cells and the paraneuron concept. J Electron Microsc Tech 1989; 12:408 - 16; http://dx.doi.org/10.1002/jemt.1060120413; PMID: 2671307
- Tanaka K, Chiba T. Microvascular organization of sympathetic ganglia, with special reference to small intensely-fluorescent cells. Microsc Res Tech 1996; 35:137 - 45; http://dx.doi.org/10.1002/(SICI)1097-0029(19961001)35:2<137::AID-JEMT4>3.0.CO;2-N; PMID: 8923448
- Kriebel RM, Angel A, Parsons RL. Biogenic amine localization in cardiac ganglion intrinsic neurons: electron microscopic histochemistry of SIF cells. Brain Res Bull 1991; 27:175 - 9; http://dx.doi.org/10.1016/0361-9230(91)90064-Q; PMID: 1742604
- Francis N, Farinas I, Brennan C, Rivas-Plata K, Backus C, Reichardt L, et al. NT-3, like NGF, is required for survival of sympathetic neurons, but not their precursors. Dev Biol 1999; 210:411 - 27; http://dx.doi.org/10.1006/dbio.1999.9269; PMID: 10357900
- Kuruvilla R, Zweifel LS, Glebova NO, Lonze BE, Valdez G, Ye H, et al. A neurotrophin signaling cascade coordinates sympathetic neuron development through differential control of TrkA trafficking and retrograde signaling. Cell 2004; 118:243 - 55; http://dx.doi.org/10.1016/j.cell.2004.06.021; PMID: 15260993
- Glebova NO, Ginty DD. Heterogeneous requirement of NGF for sympathetic target innervation in vivo. J Neurosci 2004; 24:743 - 51; http://dx.doi.org/10.1523/JNEUROSCI.4523-03.2004; PMID: 14736860
- Nam J, Onitsuka I, Hatch J, Uchida Y, Ray S, Huang S, et al. Coronary veins determine the pattern of sympathetic innervation in the developing heart. Development 2013; 140:1475 - 85; http://dx.doi.org/10.1242/dev.087601; PMID: 23462468
- Yan H, Newgreen DF, Young HM. Developmental changes in neurite outgrowth responses of dorsal root and sympathetic ganglia to GDNF, neurturin, and artemin. Dev Dyn 2003; 227:395 - 401; http://dx.doi.org/10.1002/dvdy.10294; PMID: 12815625
- Makita T, Sucov HM, Gariepy CE, Yanagisawa M, Ginty DD. Endothelins are vascular-derived axonal guidance cues for developing sympathetic neurons. Nature 2008; 452:759 - 63; http://dx.doi.org/10.1038/nature06859; PMID: 18401410
- Ben-Zvi A, Ben-Gigi L, Klein H, Behar O. Modulation of semaphorin3A activity by p75 neurotrophin receptor influences peripheral axon patterning. J Neurosci 2007; 27:13000 - 11; http://dx.doi.org/10.1523/JNEUROSCI.3373-07.2007; PMID: 18032673
- Lorentz CU, Alston EN, Belcik T, Lindner JR, Giraud GD, Habecker BA. Heterogeneous ventricular sympathetic innervation, altered beta-adrenergic receptor expression, and rhythm instability in mice lacking the p75 neurotrophin receptor. Am J Physiol Heart Circ Physiol 2010; 298:H1652 - 60; http://dx.doi.org/10.1152/ajpheart.01128.2009; PMID: 20190098
- Heumann R, Korsching S, Scott J, Thoenen H. Relationship between levels of nerve growth factor (NGF) and its messenger RNA in sympathetic ganglia and peripheral target tissues. EMBO J 1984; 3:3183 - 9; PMID: 6549295
- Korsching S, Thoenen H. Developmental changes of nerve growth factor levels in sympathetic ganglia and their target organs. Dev Biol 1988; 126:40 - 6; http://dx.doi.org/10.1016/0012-1606(88)90236-9; PMID: 3342935
- Wright LL, Cunningham TJ, Smolen AJ. Developmental neuron death in the rat superior cervical sympathetic ganglion: cell counts and ultrastructure. J Neurocytol 1983; 12:727 - 38; http://dx.doi.org/10.1007/BF01258147; PMID: 6644353
- Ruit KG, Osborne PA, Schmidt RE, Johnson EM Jr., Snider WD. Nerve growth factor regulates sympathetic ganglion cell morphology and survival in the adult mouse. J Neurosci 1990; 10:2412 - 9; PMID: 2376779
- Oppenheim RW. Cell death during development of the nervous system. Annu Rev Neurosci 1991; 14:453 - 501; http://dx.doi.org/10.1146/annurev.ne.14.030191.002321; PMID: 2031577
- Davies AM. Nerve growth factor synthesis and nerve growth factor receptor expression in neural development. Int Rev Cytol 1991; 128:109 - 38; http://dx.doi.org/10.1016/S0074-7696(08)60498-2; PMID: 1655670
- Davies AM. The role of neurotrophins in the developing nervous system. J Neurobiol 1994; 25:1334 - 48; http://dx.doi.org/10.1002/neu.480251103; PMID: 7852989
- Korsching S. The neurotrophic factor concept: a reexamination. J Neurosci 1993; 13:2739 - 48; PMID: 8331370
- Lockhart ST, Mead JN, Pisano JM, Slonimsky JD, Birren SJ. Nerve growth factor collaborates with myocyte-derived factors to promote development of presynaptic sites in cultured sympathetic neurons. J Neurobiol 2000; 42:460 - 76; http://dx.doi.org/10.1002/(SICI)1097-4695(200003)42:4<460::AID-NEU7>3.0.CO;2-#; PMID: 10699983
- Lockhart ST, Turrigiano GG, Birren SJ. Nerve growth factor modulates synaptic transmission between sympathetic neurons and cardiac myocytes. J Neurosci 1997; 17:9573 - 82; PMID: 9391012
- Zhou S, Paz O, Cao JM, Asotra K, Chai NN, Wang C, et al. Differential beta-adrenoceptor expression induced by nerve growth factor infusion into the canine right and left stellate ganglia. Heart Rhythm 2005; 2:1347 - 55; http://dx.doi.org/10.1016/j.hrthm.2005.08.027; PMID: 16360089
- Ieda M, Fukuda K, Hisaka Y, Kimura K, Kawaguchi H, Fujita J, et al. Endothelin-1 regulates cardiac sympathetic innervation in the rodent heart by controlling nerve growth factor expression. J Clin Invest 2004; 113:876 - 84; PMID: 15067320
- Zhou XF, Deng YS, Chie E, Xue Q, Zhong JH, McLachlan EM, et al. Satellite-cell-derived nerve growth factor and neurotrophin-3 are involved in noradrenergic sprouting in the dorsal root ganglia following peripheral nerve injury in the rat. Eur J Neurosci 1999; 11:1711 - 22; http://dx.doi.org/10.1046/j.1460-9568.1999.00589.x; PMID: 10215925
- Ebendal T, Jacobson CO. Tests of possible role of NGF in neurite outgrowth stimulation exerted by glial cells and heart explants in culture. Brain Res 1977; 131:373 - 8; http://dx.doi.org/10.1016/0006-8993(77)90531-5; PMID: 890466
- Varon S, Skaper SD, Manthorpe M. Trophic activities for dorsal root and sympathetic ganglionic neurons in media conditioned by Schwann and other peripheral cells. Brain Res 1981; 227:73 - 87; PMID: 7470935
- Sofroniew MV, Howe CL, Mobley WC. Nerve growth factor signaling, neuroprotection, and neural repair. Annu Rev Neurosci 2001; 24:1217 - 81; http://dx.doi.org/10.1146/annurev.neuro.24.1.1217; PMID: 11520933
- Hasan W, Pedchenko T, Krizsan-Agbas D, Baum L, Smith PG. Sympathetic neurons synthesize and secrete pro-nerve growth factor protein. J Neurobiol 2003; 57:38 - 53; http://dx.doi.org/10.1002/neu.10250; PMID: 12973827
- Marvin WJ Jr., Hermsmeyer K, McDonald RI, Roskoski LM, Roskoski R Jr.. Ontogenesis of cholingergic innervation in the rat heart. Circ Res 1980; 46:690 - 5; http://dx.doi.org/10.1161/01.RES.46.5.690; PMID: 6102497
- Heathcote RD, Sargent PB. Growth and morphogenesis of an autonomic ganglion. I. Matching neurons with target. J Neurosci 1987; 7:2493 - 501; PMID: 3612250
- Heathcote RD, Chen A. Morphogenesis of adrenergic cells in a frog parasympathetic ganglion. J Comp Neurol 1991; 308:139 - 48; http://dx.doi.org/10.1002/cne.903080112; PMID: 1874979
- Soinila S. Clustering of intensely fluorescent sympathetic cells in embryonal and postnatal rats. J Auton Nerv Syst 1984; 11:207 - 22; http://dx.doi.org/10.1016/0165-1838(84)90078-X; PMID: 6491161
- Soinila S, Eränkö O. Intensely fluorescent cells in embryonic and postnatal superior cervical ganglia of the rat cultured with or without hydrocortisone. J Auton Nerv Syst 1984; 11:43 - 57; http://dx.doi.org/10.1016/0165-1838(84)90007-9; PMID: 6470409
- Saarma M. GDNF - a stranger in the TGF-beta superfamily?. Eur J Biochem 2000; 267:6968 - 71; http://dx.doi.org/10.1046/j.1432-1327.2000.01826.x; PMID: 11106404
- Ernsberger U. The role of GDNF family ligand signalling in the differentiation of sympathetic and dorsal root ganglion neurons. Cell Tissue Res 2008; 333:353 - 71; http://dx.doi.org/10.1007/s00441-008-0634-4; PMID: 18629541
- Peterziel H, Paech T, Strelau J, Unsicker K, Krieglstein K. Specificity in the crosstalk of TGFbeta/GDNF family members is determined by distinct GFR alpha receptors. J Neurochem 2007; 103:2491 - 504; http://dx.doi.org/10.1111/j.1471-4159.2007.04962.x; PMID: 17953664
- Enomoto H, Heuckeroth RO, Golden JP, Johnson EM, Milbrandt J. Development of cranial parasympathetic ganglia requires sequential actions of GDNF and neurturin. Development 2000; 127:4877 - 89; PMID: 11044402
- Hiltunen JO, Laurikainen A, Airaksinen MS, Saarma M. GDNF family receptors in the embryonic and postnatal rat heart and reduced cholinergic innervation in mice hearts lacking ret or GFRalpha2. Dev Dyn 2000; 219:28 - 39; http://dx.doi.org/10.1002/1097-0177(2000)9999:9999<::AID-DVDY1031>3.0.CO;2-P; PMID: 10974669
- Mabe AM, Hoover DB. Structural and functional cardiac cholinergic deficits in adult neurturin knockout mice. Cardiovasc Res 2009; 82:93 - 9; http://dx.doi.org/10.1093/cvr/cvp029; PMID: 19176599
- Mabe AM, Hoard JL, Duffourc MM, Hoover DB. Localization of cholinergic innervation and neurturin receptors in adult mouse heart and expression of the neurturin gene. Cell Tissue Res 2006; 326:57 - 67; http://dx.doi.org/10.1007/s00441-006-0215-3; PMID: 16708241
- Hashino E, Shero M, Junghans D, Rohrer H, Milbrandt J, Johnson EM Jr.. GDNF and neurturin are target-derived factors essential for cranial parasympathetic neuron development. Development 2001; 128:3773 - 82; PMID: 11585803
- Hoard JL, Hoover DB, Mabe AM, Blakely RD, Feng N, Paolocci N. Cholinergic neurons of mouse intrinsic cardiac ganglia contain noradrenergic enzymes, norepinephrine transporters, and the neurotrophin receptors tropomyosin-related kinase A and p75. Neuroscience 2008; 156:129 - 42; http://dx.doi.org/10.1016/j.neuroscience.2008.06.063; PMID: 18674600
- Habecker BA, Bilimoria P, Linick C, Gritman K, Lorentz CU, Woodward W, et al. Regulation of cardiac innervation and function via the p75 neurotrophin receptor. Auton Neurosci 2008; 140:40 - 8; http://dx.doi.org/10.1016/j.autneu.2008.03.002; PMID: 18430612
- Hasan W, Woodward WR, Habecker BA. Altered atrial neurotransmitter release in transgenic p75(-/-) and gp130 KO mice. Neurosci Lett 2012; 529:55 - 9; http://dx.doi.org/10.1016/j.neulet.2012.08.089; PMID: 22999927
- Stewart AL, Anderson RB, Kobayashi K, Young HM. Effects of NGF, NT-3 and GDNF family members on neurite outgrowth and migration from pelvic ganglia from embryonic and newborn mice. BMC Dev Biol 2008; 8:73; http://dx.doi.org/10.1186/1471-213X-8-73; PMID: 18657279
- Meusburger SM, Keast JR. Testosterone and nerve growth factor have distinct but interacting effects on structure and neurotransmitter expression of adult pelvic ganglion cells in vitro. Neuroscience 2001; 108:331 - 40; http://dx.doi.org/10.1016/S0306-4522(01)00420-1; PMID: 11734365
- Hazari MS, Pan JH, Myers AC. Nerve growth factor acutely potentiates synaptic transmission in vitro and induces dendritic growth in vivo on adult neurons in airway parasympathetic ganglia. Am J Physiol Lung Cell Mol Physiol 2007; 292:L992 - 1001; http://dx.doi.org/10.1152/ajplung.00216.2006; PMID: 17158596
- Collins F. Developmental time course of the effect of nerve growth factor on the parasympathetic ciliary ganglion. Brain Res 1988; 467:111 - 6; PMID: 3359323
- Collins F, Dawson A. An effect of nerve growth factor on parasympathetic neurite outgrowth. Proc Natl Acad Sci U S A 1983; 80:2091 - 4; http://dx.doi.org/10.1073/pnas.80.7.2091; PMID: 6340114
- Smith PG, Warn JD, Steinle JJ, Krizsan-Agbas D, Hasan W. Modulation of parasympathetic neuron phenotype and function by sympathetic innervation. Auton Neurosci 2002; 96:33 - 42; http://dx.doi.org/10.1016/S1566-0702(01)00371-X; PMID: 11911500
- Hasan W, Smith PG. Nerve growth factor expression in parasympathetic neurons: regulation by sympathetic innervation. Eur J Neurosci 2000; 12:4391 - 7; http://dx.doi.org/10.1046/j.0953-816X.2000.01353.x; PMID: 11122349
- Hasan W, Smith PG. Modulation of rat parasympathetic cardiac ganglion phenotype and NGF synthesis by adrenergic nerves. Auton Neurosci 2009; 145:17 - 26; http://dx.doi.org/10.1016/j.autneu.2008.10.012; PMID: 19019738
- Rana OR, Saygili E, Gemein C, Zink MD, Buhr A, Saygili E, et al. Chronic electrical neuronal stimulation increases cardiac parasympathetic tone by eliciting neurotrophic effects. Circ Res 2011; 108:1209 - 19; http://dx.doi.org/10.1161/CIRCRESAHA.110.234518; PMID: 21441135
- James JM, Mukouyama YS. Neuronal action on the developing blood vessel pattern. Semin Cell Dev Biol 2011; 22:1019 - 27; http://dx.doi.org/10.1016/j.semcdb.2011.09.010; PMID: 21978864
- Storkebaum E, Carmeliet P. Paracrine control of vascular innervation in health and disease. Acta Physiol (Oxf) 2011; 203:61 - 86; http://dx.doi.org/10.1111/j.1748-1716.2011.02333.x; PMID: 21689379
- Lazarovici P, Marcinkiewicz C, Lelkes PI. Cross talk between the cardiovascular and nervous systems: neurotrophic effects of vascular endothelial growth factor (VEGF) and angiogenic effects of nerve growth factor (NGF)-implications in drug development. Curr Pharm Des 2006; 12:2609 - 22; http://dx.doi.org/10.2174/138161206777698738; PMID: 16842161
- Nico B, Mangieri D, Benagiano V, Crivellato E, Ribatti D. Nerve growth factor as an angiogenic factor. Microvasc Res 2008; 75:135 - 41; http://dx.doi.org/10.1016/j.mvr.2007.07.004; PMID: 17764704
- Caporali A, Emanueli C. Cardiovascular actions of neurotrophins. Physiol Rev 2009; 89:279 - 308; http://dx.doi.org/10.1152/physrev.00007.2008; PMID: 19126759
- Donovan MJ, Hahn R, Tessarollo L, Hempstead BL. Identification of an essential nonneuronal function of neurotrophin 3 in mammalian cardiac development. Nat Genet 1996; 14:210 - 3; http://dx.doi.org/10.1038/ng1096-210; PMID: 8841198
- Malpas SC. Sympathetic nervous system overactivity and its role in the development of cardiovascular disease. Physiol Rev 2010; 90:513 - 57; http://dx.doi.org/10.1152/physrev.00007.2009; PMID: 20393193
- Parati G, Esler M. The human sympathetic nervous system: its relevance in hypertension and heart failure. Eur Heart J 2012; 33:1058 - 66; http://dx.doi.org/10.1093/eurheartj/ehs041; PMID: 22507981
- Fisher JP, Paton JF. The sympathetic nervous system and blood pressure in humans: implications for hypertension. J Hum Hypertens 2012; 26:463 - 75; http://dx.doi.org/10.1038/jhh.2011.66; PMID: 21734720
- Wenzel RR, Spieker L, Qui S, Shaw S, Lüscher TF, Noll G. I1-imidazoline agonist moxonidine decreases sympathetic nerve activity and blood pressure in hypertensives. Hypertension 1998; 32:1022 - 7; http://dx.doi.org/10.1161/01.HYP.32.6.1022; PMID: 9856967
- Esler M, Lux A, Jennings G, Hastings J, Socratous F, Lambert G. Rilmenidine sympatholytic activity preserves mental stress, orthostatic sympathetic responses and adrenaline secretion. J Hypertens 2004; 22:1529 - 34; http://dx.doi.org/10.1097/01.hjh.0000125453.28861.b8; PMID: 15257176
- Arsenault KA, Yusuf AM, Crystal E, Healey JS, Morillo CA, Nair GM, et al. Interventions for preventing post-operative atrial fibrillation in patients undergoing heart surgery. Cochrane Database Syst Rev 2013; 1:CD003611; PMID: 23440790
- Feldman D, Elton TS, Menachemi DM, Wexler RK. Heart rate control with adrenergic blockade: clinical outcomes in cardiovascular medicine. Vasc Health Risk Manag 2010; 6:387 - 97; http://dx.doi.org/10.2147/VHRM.S10358; PMID: 20539841
- Triposkiadis F, Karayannis G, Giamouzis G, Skoularigis J, Louridas G, Butler J. The sympathetic nervous system in heart failure physiology, pathophysiology, and clinical implications. J Am Coll Cardiol 2009; 54:1747 - 62; http://dx.doi.org/10.1016/j.jacc.2009.05.015; PMID: 19874988
- Frishman WH, Aronow WS. Pharmacology of antiarrhythmic drugs in elderly patients. Clin Geriatr Med 2012; 28:575 - 615; http://dx.doi.org/10.1016/j.cger.2012.07.001; PMID: 23101572
- Chatterjee S, Biondi-Zoccai G, Abbate A, D’Ascenzo F, Castagno D, Van Tassell B, et al. Benefits of β blockers in patients with heart failure and reduced ejection fraction: network meta-analysis. BMJ 2013; 346:f55; http://dx.doi.org/10.1136/bmj.f55; PMID: 23325883
- Slotkin TA, Seidler FJ. Terbutaline impairs the development of peripheral noradrenergic projections: Potential implications for autism spectrum disorders and pharmacotherapy of preterm labor. Neurotoxicol Teratol 2013; 36:91 - 6; PMID: 22813780
- Clarke GL, Bhattacherjee A, Tague SE, Hasan W, Smith PG. ß-adrenoceptor blockers increase cardiac sympathetic innervation by inhibiting autoreceptor suppression of axon growth. J Neurosci 2010; 30:12446 - 54; http://dx.doi.org/10.1523/JNEUROSCI.1667-10.2010; PMID: 20844139
- Chen PS, Chen LS, Cao JM, Sharifi B, Karagueuzian HS, Fishbein MC. Sympathetic nerve sprouting, electrical remodeling and the mechanisms of sudden cardiac death. Cardiovasc Res 2001; 50:409 - 16; http://dx.doi.org/10.1016/S0008-6363(00)00308-4; PMID: 11334845
- Saygili E, Schauerte P, Küppers F, Heck L, Weis J, Weber C, et al. Electrical stimulation of sympathetic neurons induces autocrine/paracrine effects of NGF mediated by TrkA. J Mol Cell Cardiol 2010; 49:79 - 87; http://dx.doi.org/10.1016/j.yjmcc.2010.01.019; PMID: 20138055
- Zipes DP. Heart-brain interactions in cardiac arrhythmias: role of the autonomic nervous system. Cleve Clin J Med 2008; 75:Suppl 2 S94 - 6; http://dx.doi.org/10.3949/ccjm.75.Suppl_2.S94; PMID: 18540155
- Ieda M, Fukuda K. Cardiac innervation and sudden cardiac death. Curr Cardiol Rev 2009; 5:289 - 95; http://dx.doi.org/10.2174/157340309789317904; PMID: 21037846
- Volders PG. Novel insights into the role of the sympathetic nervous system in cardiac arrhythmogenesis. Heart Rhythm 2010; 7:1900 - 6; http://dx.doi.org/10.1016/j.hrthm.2010.06.003; PMID: 20570754
- Arora R. Recent insights into the role of the autonomic nervous system in the creation of substrate for atrial fibrillation: implications for therapies targeting the atrial autonomic nervous system. Circ Arrhythm Electrophysiol 2012; 5:850 - 9; http://dx.doi.org/10.1161/CIRCEP.112.972273; PMID: 22895601
- Park HW, Shen MJ, Lin SF, Fishbein MC, Chen LS, Chen PS. Neural mechanisms of atrial fibrillation. Curr Opin Cardiol 2012; 27:24 - 8; http://dx.doi.org/10.1097/HCO.0b013e32834dc4e8; PMID: 22139702
- Chen PS, Choi EK, Zhou S, Lin SF, Chen LS. Cardiac neural remodeling and its role in arrhythmogenesis. Heart Rhythm 2010; 7:1512 - 3; http://dx.doi.org/10.1016/j.hrthm.2010.05.020; PMID: 20478404
- Billette J, Tadros R. Concealed autonomic mechanisms underlying atrial fibrillation. J Cardiovasc Electrophysiol 2013; 24:196 - 8; http://dx.doi.org/10.1111/jce.12050; PMID: 23217109
- Olgin JE, Sih HJ, Hanish S, Jayachandran JV, Wu J, Zheng QH, et al. Heterogeneous atrial denervation creates substrate for sustained atrial fibrillation. Circulation 1998; 98:2608 - 14; http://dx.doi.org/10.1161/01.CIR.98.23.2608; PMID: 9843470
- Gould PA, Yii M, McLean C, Finch S, Marshall T, Lambert GW, et al. Evidence for increased atrial sympathetic innervation in persistent human atrial fibrillation. Pacing Clin Electrophysiol 2006; 29:821 - 9; http://dx.doi.org/10.1111/j.1540-8159.2006.00447.x; PMID: 16922997
- Ng J, Villuendas R, Cokic I, Schliamser JE, Gordon D, Koduri H, et al. Autonomic remodeling in the left atrium and pulmonary veins in heart failure: creation of a dynamic substrate for atrial fibrillation. Circ Arrhythm Electrophysiol 2011; 4:388 - 96; http://dx.doi.org/10.1161/CIRCEP.110.959650; PMID: 21421805
- Jayachandran JV, Sih HJ, Winkle W, Zipes DP, Hutchins GD, Olgin JE. Atrial fibrillation produced by prolonged rapid atrial pacing is associated with heterogeneous changes in atrial sympathetic innervation. Circulation 2000; 101:1185 - 91; http://dx.doi.org/10.1161/01.CIR.101.10.1185; PMID: 10715267
- Chang CM, Wu TJ, Zhou S, Doshi RN, Lee MH, Ohara T, et al. Nerve sprouting and sympathetic hyperinnervation in a canine model of atrial fibrillation produced by prolonged right atrial pacing. Circulation 2001; 103:22 - 5; http://dx.doi.org/10.1161/01.CIR.103.1.22; PMID: 11136680
- Lu Z, Scherlag BJ, Lin J, Niu G, Fung KM, Zhao L, et al. Atrial fibrillation begets atrial fibrillation: autonomic mechanism for atrial electrical remodeling induced by short-term rapid atrial pacing. Circ Arrhythm Electrophysiol 2008; 1:184 - 92; http://dx.doi.org/10.1161/CIRCEP.108.784272; PMID: 19808412
- Hamabe A, Chang CM, Zhou S, Chou CC, Yi J, Miyauchi Y, et al. Induction of atrial fibrillation and nerve sprouting by prolonged left atrial pacing in dogs. Pacing Clin Electrophysiol 2003; 26:2247 - 52; http://dx.doi.org/10.1111/j.1540-8159.2003.00355.x; PMID: 14675008
- Miyauchi Y, Zhou S, Okuyama Y, Miyauchi M, Hayashi H, Hamabe A, et al. Altered atrial electrical restitution and heterogeneous sympathetic hyperinnervation in hearts with chronic left ventricular myocardial infarction: implications for atrial fibrillation. Circulation 2003; 108:360 - 6; http://dx.doi.org/10.1161/01.CIR.0000080327.32573.7C; PMID: 12835207
- Tan AY, Zhou S, Ogawa M, Song J, Chu M, Li H, et al. Neural mechanisms of paroxysmal atrial fibrillation and paroxysmal atrial tachycardia in ambulatory canines. Circulation 2008; 118:916 - 25; http://dx.doi.org/10.1161/CIRCULATIONAHA.108.776203; PMID: 18697820
- Swissa M, Zhou S, Tan AY, Fishbein MC, Chen PS, Chen LS. Atrial sympathetic and parasympathetic nerve sprouting and hyperinnervation induced by subthreshold electrical stimulation of the left stellate ganglion in normal dogs. Cardiovasc Pathol 2008; 17:303 - 8; http://dx.doi.org/10.1016/j.carpath.2007.11.003; PMID: 18692409
- Hwang C, Chen PS. Ligament of Marshall: why it is important for atrial fibrillation ablation. Heart Rhythm 2009; 6:Suppl S35 - 40; http://dx.doi.org/10.1016/j.hrthm.2009.08.034; PMID: 19959141
- Wu TJ, Ong JJ, Chang CM, Doshi RN, Yashima M, Huang HL, et al. Pulmonary veins and ligament of Marshall as sources of rapid activations in a canine model of sustained atrial fibrillation. Circulation 2001; 103:1157 - 63; http://dx.doi.org/10.1161/01.CIR.103.8.1157; PMID: 11222481
- Okuyama Y, Miyauchi Y, Park AM, Hamabe A, Zhou S, Hayashi H, et al. High resolution mapping of the pulmonary vein and the vein of Marshall during induced atrial fibrillation and atrial tachycardia in a canine model of pacing-induced congestive heart failure. J Am Coll Cardiol 2003; 42:348 - 60; http://dx.doi.org/10.1016/S0735-1097(03)00586-2; PMID: 12875775
- Coleman MA, Bos JM, Johnson JN, Owen HJ, Deschamps C, Moir C, et al. Videoscopic left cardiac sympathetic denervation for patients with recurrent ventricular fibrillation/malignant ventricular arrhythmia syndromes besides congenital long-QT syndrome. Circ Arrhythm Electrophysiol 2012; 5:782 - 8; http://dx.doi.org/10.1161/CIRCEP.112.971754; PMID: 22787014
- Nakagawa H, Scherlag BJ, Patterson E, Ikeda A, Lockwood D, Jackman WM. Pathophysiologic basis of autonomic ganglionated plexus ablation in patients with atrial fibrillation. Heart Rhythm 2009; 6:Suppl S26 - 34; http://dx.doi.org/10.1016/j.hrthm.2009.07.029; PMID: 19959140
- Po SS, Nakagawa H, Jackman WM. Localization of left atrial ganglionated plexi in patients with atrial fibrillation. J Cardiovasc Electrophysiol 2009; 20:1186 - 9; http://dx.doi.org/10.1111/j.1540-8167.2009.01515.x; PMID: 19563367
- Stöllberger C, Schneider B, Winkler-Dworak M, Finsterer J. Prevention of embolic stroke by catheter ablation of atrial fibrillation. Eur J Neurol 2008; 15:1419 - 20; http://dx.doi.org/10.1111/j.1468-1331.2008.02341.x; PMID: 19049566
- Li D, Fareh S, Leung TK, Nattel S. Promotion of atrial fibrillation by heart failure in dogs: atrial remodeling of a different sort. Circulation 1999; 100:87 - 95; http://dx.doi.org/10.1161/01.CIR.100.1.87; PMID: 10393686
- Liu Y, Scherlag BJ, Fan Y, Varma V, Male S, Chaudhry MA, et al. Inducibility of atrial fibrillation after GP ablations and “autonomic blockade”: evidence for the pathophysiological role of the nonadrenergic and noncholinergic neurotransmitters. J Cardiovasc Electrophysiol 2013; 24:188 - 95; http://dx.doi.org/10.1111/j.1540-8167.2012.02449.x; PMID: 23066921
- Fallavollita JA, Canty JM Jr.. Dysinnervated but viable myocardium in ischemic heart disease. J Nucl Cardiol 2010; 17:1107 - 15; http://dx.doi.org/10.1007/s12350-010-9292-5; PMID: 20857351
- Buendia-Fuentes F, Almenar L, Ruiz C, Vercher JL, Sánchez-Lázaro I, Martínez-Dolz L, et al. Sympathetic reinnervation 1 year after heart transplantation, assessed using iodine-123 metaiodobenzylguanidine imaging. Transplant Proc 2011; 43:2247 - 8; http://dx.doi.org/10.1016/j.transproceed.2011.05.020; PMID: 21839246
- Wernli G, Hasan W, Bhattacherjee A, van Rooijen N, Smith PG. Macrophage depletion suppresses sympathetic hyperinnervation following myocardial infarction. Basic Res Cardiol 2009; 104:681 - 93; http://dx.doi.org/10.1007/s00395-009-0033-3; PMID: 19437062
- Hiltunen JO, Laurikainen A, Väkevä A, Meri S, Saarma M. Nerve growth factor and brain-derived neurotrophic factor mRNAs are regulated in distinct cell populations of rat heart after ischaemia and reperfusion. J Pathol 2001; 194:247 - 53; http://dx.doi.org/10.1002/path.878; PMID: 11400155
- Hasan W, Jama A, Donohue T, Wernli G, Onyszchuk G, Al-Hafez B, et al. Sympathetic hyperinnervation and inflammatory cell NGF synthesis following myocardial infarction in rats. Brain Res 2006; 1124:142 - 54; http://dx.doi.org/10.1016/j.brainres.2006.09.054; PMID: 17084822
- Cao JM, Chen LS, KenKnight BH, Ohara T, Lee MH, Tsai J, et al. Nerve sprouting and sudden cardiac death. Circ Res 2000; 86:816 - 21; http://dx.doi.org/10.1161/01.RES.86.7.816; PMID: 10764417
- Kimura K, Ieda M, Kanazawa H, Yagi T, Tsunoda M, Ninomiya S, et al. Cardiac sympathetic rejuvenation: a link between nerve function and cardiac hypertrophy. Circ Res 2007; 100:1755 - 64; http://dx.doi.org/10.1161/01.RES.0000269828.62250.ab; PMID: 17495227
- Zhou S, Chen LS, Miyauchi Y, Miyauchi M, Kar S, Kangavari S, et al. Mechanisms of cardiac nerve sprouting after myocardial infarction in dogs. Circ Res 2004; 95:76 - 83; http://dx.doi.org/10.1161/01.RES.0000133678.22968.e3; PMID: 15166093
- Vracko R, Thorning D, Frederickson RG. Fate of nerve fibers in necrotic, healing, and healed rat myocardium. Lab Invest 1990; 63:490 - 501; PMID: 2232703
- Wang Y, Xuan YL, Hu HS, Li XL, Xue M, Cheng WJ, et al. Risk of ventricular arrhythmias after myocardial infarction with diabetes associated with sympathetic neural remodeling in rabbits. Cardiology 2012; 121:1 - 9; http://dx.doi.org/10.1159/000336148; PMID: 22377967
- Vracko R, Thorning D, Frederickson RG. Nerve fibers in human myocardial scars. Hum Pathol 1991; 22:138 - 46; http://dx.doi.org/10.1016/0046-8177(91)90035-N; PMID: 1705914
- Nguyen BL, Li H, Fishbein MC, Lin SF, Gaudio C, Chen PS, et al. Acute myocardial infarction induces bilateral stellate ganglia neural remodeling in rabbits. Cardiovasc Pathol 2012; 21:143 - 8; http://dx.doi.org/10.1016/j.carpath.2011.08.001; PMID: 22001051
- Ulphani JS, Cain JH, Inderyas F, Gordon D, Gikas PV, Shade G, et al. Quantitative analysis of parasympathetic innervation of the porcine heart. Heart Rhythm 2010; 7:1113 - 9; http://dx.doi.org/10.1016/j.hrthm.2010.03.043; PMID: 20381645
- Lobos E, Gebhardt C, Kluge A, Spanel-Borowski K. Expression of nerve growth factor (NGF) isoforms in the rat uterus during pregnancy: accumulation of precursor proNGF. Endocrinology 2005; 146:1922 - 9; http://dx.doi.org/10.1210/en.2004-0925; PMID: 15637294
- Bierl MA, Isaacson LG. Increased NGF proforms in aged sympathetic neurons and their targets. Neurobiol Aging 2007; 28:122 - 34; http://dx.doi.org/10.1016/j.neurobiolaging.2005.11.008; PMID: 16377033
- Al-Shawi R, Hafner A, Olsen J, Chun S, Raza S, Thrasivoulou C, et al. Neurotoxic and neurotrophic roles of proNGF and the receptor sortilin in the adult and ageing nervous system. Eur J Neurosci 2008; 27:2103 - 14; http://dx.doi.org/10.1111/j.1460-9568.2008.06152.x; PMID: 18412630
- Loiacono RE, Story DF. Effect of alpha-adrenoceptor agonists and antagonists on cholinergic transmission in guinea-pig isolated atria. Naunyn Schmiedebergs Arch Pharmacol 1986; 334:40 - 7; http://dx.doi.org/10.1007/BF00498738; PMID: 2878372
- Wetzel GT, Goldstein D, Brown JH. Acetylcholine release from rat atria can be regulated through an alpha 1-adrenergic receptor. Circ Res 1985; 56:763 - 6; http://dx.doi.org/10.1161/01.RES.56.5.763; PMID: 2986875
- Qin F, Vulapalli RS, Stevens SY, Liang CS. Loss of cardiac sympathetic neurotransmitters in heart failure and NE infusion is associated with reduced NGF. Am J Physiol Heart Circ Physiol 2002; 282:H363 - 71; PMID: 11748083
- Kaye DM, Vaddadi G, Gruskin SL, Du XJ, Esler MD. Reduced myocardial nerve growth factor expression in human and experimental heart failure. Circ Res 2000; 86:E80 - 4; http://dx.doi.org/10.1161/01.RES.86.7.e80; PMID: 10764418
- Kreusser MM, Buss SJ, Krebs J, Kinscherf R, Metz J, Katus HA, et al. Differential expression of cardiac neurotrophic factors and sympathetic nerve ending abnormalities within the failing heart. J Mol Cell Cardiol 2008; 44:380 - 7; http://dx.doi.org/10.1016/j.yjmcc.2007.10.019; PMID: 18037433
- Stanton MS, Tuli MM, Radtke NL, Heger JJ, Miles WM, Mock BH, et al. Regional sympathetic denervation after myocardial infarction in humans detected noninvasively using I-123-metaiodobenzylguanidine. J Am Coll Cardiol 1989; 14:1519 - 26; http://dx.doi.org/10.1016/0735-1097(89)90391-4; PMID: 2809013
- Minardo JD, Tuli MM, Mock BH, Weiner RE, Pride HP, Wellman HN, et al. Scintigraphic and electrophysiological evidence of canine myocardial sympathetic denervation and reinnervation produced by myocardial infarction or phenol application. Circulation 1988; 78:1008 - 19; http://dx.doi.org/10.1161/01.CIR.78.4.1008; PMID: 3168182
- Hartikainen J, Kuikka J, Mäntysaari M, Länsimies E, Pyörälä K. Sympathetic reinnervation after acute myocardial infarction. Am J Cardiol 1996; 77:5 - 9; http://dx.doi.org/10.1016/S0002-9149(97)89125-4; PMID: 8540457
- Barber MJ, Mueller TM, Henry DP, Felten SY, Zipes DP. Transmural myocardial infarction in the dog produces sympathectomy in noninfarcted myocardium. Circulation 1983; 67:787 - 96; http://dx.doi.org/10.1161/01.CIR.67.4.787; PMID: 6825234
- Backs J, Haunstetter A, Gerber SH, Metz J, Borst MM, Strasser RH, et al. The neuronal norepinephrine transporter in experimental heart failure: evidence for a posttranscriptional downregulation. J Mol Cell Cardiol 2001; 33:461 - 72; http://dx.doi.org/10.1006/jmcc.2000.1319; PMID: 11181015
- Böhm M, La Rosée K, Schwinger RH, Erdmann E. Evidence for reduction of norepinephrine uptake sites in the failing human heart. J Am Coll Cardiol 1995; 25:146 - 53; http://dx.doi.org/10.1016/0735-1097(94)00353-R; PMID: 7798493
- Chidsey CA, Braunwald E, Morrow AG. Catecholamine Excretion and Cardiac Stores of Norepinephrine in Congestive Heart Failure. Am J Med 1965; 39:442 - 51; http://dx.doi.org/10.1016/0002-9343(65)90211-1; PMID: 14338295
- Hasking GJ, Esler MD, Jennings GL, Burton D, Johns JA, Korner PI. Norepinephrine spillover to plasma in patients with congestive heart failure: evidence of increased overall and cardiorenal sympathetic nervous activity. Circulation 1986; 73:615 - 21; http://dx.doi.org/10.1161/01.CIR.73.4.615; PMID: 3948363
- Eisenhofer G, Friberg P, Rundqvist B, Quyyumi AA, Lambert G, Kaye DM, et al. Cardiac sympathetic nerve function in congestive heart failure. Circulation 1996; 93:1667 - 76; http://dx.doi.org/10.1161/01.CIR.93.9.1667; PMID: 8653872
- Rundqvist B, Elam M, Bergmann-Sverrisdottir Y, Eisenhofer G, Friberg P. Increased cardiac adrenergic drive precedes generalized sympathetic activation in human heart failure. Circulation 1997; 95:169 - 75; http://dx.doi.org/10.1161/01.CIR.95.1.169; PMID: 8994433
- Meredith IT, Broughton A, Jennings GL, Esler MD. Evidence of a selective increase in cardiac sympathetic activity in patients with sustained ventricular arrhythmias. N Engl J Med 1991; 325:618 - 24; http://dx.doi.org/10.1056/NEJM199108293250905; PMID: 1861695
- Ramchandra R, Hood SG, Denton DA, Woods RL, McKinley MJ, McAllen RM, et al. Basis for the preferential activation of cardiac sympathetic nerve activity in heart failure. Proc Natl Acad Sci U S A 2009; 106:924 - 8; http://dx.doi.org/10.1073/pnas.0811929106; PMID: 19136635
- Floras JS. Clinical aspects of sympathetic activation and parasympathetic withdrawal in heart failure. J Am Coll Cardiol 1993; 22:Suppl A 72A - 84A; http://dx.doi.org/10.1016/0735-1097(93)90466-E; PMID: 8376699
- Himura Y, Felten SY, Kashiki M, Lewandowski TJ, Delehanty JM, Liang CS. Cardiac noradrenergic nerve terminal abnormalities in dogs with experimental congestive heart failure. Circulation 1993; 88:1299 - 309; http://dx.doi.org/10.1161/01.CIR.88.3.1299; PMID: 8102598
- Kimura K, Kanazawa H, Ieda M, Kawaguchi-Manabe H, Miyake Y, Yagi T, et al. Norepinephrine-induced nerve growth factor depletion causes cardiac sympathetic denervation in severe heart failure. Auton Neurosci 2010; 156:27 - 35; http://dx.doi.org/10.1016/j.autneu.2010.02.005; PMID: 20335077
- Rana OR, Saygili E, Meyer C, Gemein C, Krüttgen A, Andrzejewski MG, et al. Regulation of nerve growth factor in the heart: the role of the calcineurin-NFAT pathway. J Mol Cell Cardiol 2009; 46:568 - 78; http://dx.doi.org/10.1016/j.yjmcc.2008.12.006; PMID: 19150448
- Li W, Knowlton D, Van Winkle DM, Habecker BA. Infarction alters both the distribution and noradrenergic properties of cardiac sympathetic neurons. Am J Physiol Heart Circ Physiol 2004; 286:H2229 - 36; http://dx.doi.org/10.1152/ajpheart.00768.2003; PMID: 14726300
- Parrish DC, Alston EN, Rohrer H, Nkadi P, Woodward WR, Schütz G, et al. Infarction-induced cytokines cause local depletion of tyrosine hydroxylase in cardiac sympathetic nerves. Exp Physiol 2010; 95:304 - 14; http://dx.doi.org/10.1113/expphysiol.2009.049965; PMID: 19880537
- Shi X, Habecker BA. gp130 cytokines stimulate proteasomal degradation of tyrosine hydroxylase via extracellular signal regulated kinases 1 and 2. J Neurochem 2012; 120:239 - 47; http://dx.doi.org/10.1111/j.1471-4159.2011.07539.x; PMID: 22007720
- Esler M, Jennings G, Lambert G, Meredith I, Horne M, Eisenhofer G. Overflow of catecholamine neurotransmitters to the circulation: source, fate, and functions. Physiol Rev 1990; 70:963 - 85; PMID: 1977182
- Mathes P, Cowan C, Gudbjarnason S. Storage and metabolism of norepinephrine after experimental myocardial infarction. Am J Physiol 1971; 220:27 - 32; PMID: 5538664
- Parrish DC, Gritman K, Van Winkle DM, Woodward WR, Bader M, Habecker BA. Postinfarct sympathetic hyperactivity differentially stimulates expression of tyrosine hydroxylase and norepinephrine transporter. Am J Physiol Heart Circ Physiol 2008; 294:H99 - 106; http://dx.doi.org/10.1152/ajpheart.00533.2007; PMID: 17951370
- Pellegrino MJ, Parrish DC, Zigmond RE, Habecker BA. Cytokines inhibit norepinephrine transporter expression by decreasing Hand2. Mol Cell Neurosci 2011; 46:671 - 80; http://dx.doi.org/10.1016/j.mcn.2011.01.008; PMID: 21241805
- Guidry GL, Landis SC. Developmental regulation of neurotransmitters in sympathetic neurons. Adv Pharmacol 1998; 42:895 - 8; http://dx.doi.org/10.1016/S1054-3589(08)60891-1; PMID: 9328042
- Habecker BA, Symes AJ, Stahl N, Francis NJ, Economides A, Fink JS, et al. A sweat gland-derived differentiation activity acts through known cytokine signaling pathways. J Biol Chem 1997; 272:30421 - 8; http://dx.doi.org/10.1074/jbc.272.48.30421; PMID: 9374533
- Kanazawa H, Ieda M, Kimura K, Arai T, Kawaguchi-Manabe H, Matsuhashi T, et al. Heart failure causes cholinergic transdifferentiation of cardiac sympathetic nerves via gp130-signaling cytokines in rodents. J Clin Invest 2010; 120:408 - 21; http://dx.doi.org/10.1172/JCI39778; PMID: 20051627
- Smith-White MA, Wallace D, Potter EK. Sympathetic-parasympathetic interactions at the heart in the anaesthetised rat. J Auton Nerv Syst 1999; 75:171 - 5; http://dx.doi.org/10.1016/S0165-1838(98)00169-6; PMID: 10189119
- Lundberg JM, Hua XY, Franco-Cereceda A. Effects of neuropeptide Y (NPY) on mechanical activity and neurotransmission in the heart, vas deferens and urinary bladder of the guinea-pig. Acta Physiol Scand 1984; 121:325 - 32; http://dx.doi.org/10.1111/j.1748-1716.1984.tb07463.x; PMID: 6091417
- Alston EN, Parrish DC, Hasan W, Tharp K, Pahlmeyer L, Habecker BA. Cardiac ischemia-reperfusion regulates sympathetic neuropeptide expression through gp130-dependent and independent mechanisms. Neuropeptides 2011; 45:33 - 42; http://dx.doi.org/10.1016/j.npep.2010.10.002; PMID: 21035185
- Potter EK, Smith-White MA. Galanin modulates cholinergic neurotransmission in the heart. Neuropeptides 2005; 39:345 - 8; http://dx.doi.org/10.1016/j.npep.2004.12.006; PMID: 15944033
- Smith-White MA, Iismaa TP, Potter EK. Galanin and neuropeptide Y reduce cholinergic transmission in the heart of the anaesthetised mouse. Br J Pharmacol 2003; 140:170 - 8; http://dx.doi.org/10.1038/sj.bjp.0705404; PMID: 12967946
- Smith-White MA, Herzog H, Potter EK. Role of neuropeptide Y Y(2) receptors in modulation of cardiac parasympathetic neurotransmission. Regul Pept 2002; 103:105 - 11; http://dx.doi.org/10.1016/S0167-0115(01)00368-8; PMID: 11786149
- Schwertfeger E, Klein T, Vonend O, Oberhauser V, Stegbauer J, Rump LC. Neuropeptide Y inhibits acetylcholine release in human heart atrium by activation of Y2-receptors. Naunyn Schmiedebergs Arch Pharmacol 2004; 369:455 - 61; http://dx.doi.org/10.1007/s00210-004-0930-9; PMID: 15103451
- Shishehbor MH, Alves C, Rajagopal V. Inflammation: implications for understanding the heart-brain connection. Cleve Clin J Med 2007; 74:Suppl 1 S37 - 41; http://dx.doi.org/10.3949/ccjm.74.Suppl_1.S37; PMID: 17455542
- Borovikova LV, Ivanova S, Zhang M, Yang H, Botchkina GI, Watkins LR, et al. Vagus nerve stimulation attenuates the systemic inflammatory response to endotoxin. Nature 2000; 405:458 - 62; http://dx.doi.org/10.1038/35013070; PMID: 10839541
- Karayannis G, Giamouzis G, Cokkinos DV, Skoularigis J, Triposkiadis F. Diabetic cardiovascular autonomic neuropathy: clinical implications. Expert Rev Cardiovasc Ther 2012; 10:747 - 65; http://dx.doi.org/10.1586/erc.12.53; PMID: 22894631
- Kuehl M, Stevens MJ. Cardiovascular autonomic neuropathies as complications of diabetes mellitus. Nat Rev Endocrinol 2012; 8:405 - 16; http://dx.doi.org/10.1038/nrendo.2012.21; PMID: 22371159
- Pop-Busui R. What do we know and we do not know about cardiovascular autonomic neuropathy in diabetes. J Cardiovasc Transl Res 2012; 5:463 - 78; http://dx.doi.org/10.1007/s12265-012-9367-6; PMID: 22644723
- Pop-Busui R, Cleary PA, Braffett BH, Martin CL, Herman WH, Low PA, et al, DCCT/EDIC Research Group. Association between cardiovascular autonomic neuropathy and left ventricular dysfunction: DCCT/EDIC study (Diabetes Control and Complications Trial/Epidemiology of Diabetes Interventions and Complications). J Am Coll Cardiol 2013; 61:447 - 54; http://dx.doi.org/10.1016/j.jacc.2012.10.028; PMID: 23265339
- Yang B, Chon KH. Assessment of diabetic cardiac autonomic neuropathy in type I diabetic mice. Conf Proc IEEE Eng Med Biol Soc 2011; 2011:6560 - 3; PMID: 22255842
- Mabe AM, Hoover DB. Remodeling of cardiac cholinergic innervation and control of heart rate in mice with streptozotocin-induced diabetes. Auton Neurosci 2011; 162:24 - 31; http://dx.doi.org/10.1016/j.autneu.2011.01.008; PMID: 21334985
- Lambert GW, Straznicky NE, Lambert EA, Dixon JB, Schlaich MP. Sympathetic nervous activation in obesity and the metabolic syndrome--causes, consequences and therapeutic implications. Pharmacol Ther 2010; 126:159 - 72; http://dx.doi.org/10.1016/j.pharmthera.2010.02.002; PMID: 20171982
- Skrapari I, Tentolouris N, Perrea D, Bakoyiannis C, Papazafiropoulou A, Katsilambros N. Baroreflex sensitivity in obesity: relationship with cardiac autonomic nervous system activity. Obesity (Silver Spring) 2007; 15:1685 - 93; http://dx.doi.org/10.1038/oby.2007.201; PMID: 17636086
- Lambert E, Sari CI, Dawood T, Nguyen J, McGrane M, Eikelis N, et al. Sympathetic nervous system activity is associated with obesity-induced subclinical organ damage in young adults. Hypertension 2010; 56:351 - 8; http://dx.doi.org/10.1161/HYPERTENSIONAHA.110.155663; PMID: 20625075
- Straznicky NE, Lambert GW, Lambert EA. Neuroadrenergic dysfunction in obesity: an overview of the effects of weight loss. Curr Opin Lipidol 2010; 21:21 - 30; http://dx.doi.org/10.1097/MOL.0b013e3283329c62; PMID: 19809312
- Rahmouni K, Correia ML, Haynes WG, Mark AL. Obesity-associated hypertension: new insights into mechanisms. Hypertension 2005; 45:9 - 14; PMID: 15583075
- Grassi G, Arenare F, Quarti-Trevano F, Seravalle G, Mancia G. Heart rate, sympathetic cardiovascular influences, and the metabolic syndrome. Prog Cardiovasc Dis 2009; 52:31 - 7; http://dx.doi.org/10.1016/j.pcad.2009.05.007; PMID: 19615491
- Grassi G, Seravalle G, Colombo M, Bolla G, Cattaneo BM, Cavagnini F, et al. Body weight reduction, sympathetic nerve traffic, and arterial baroreflex in obese normotensive humans. Circulation 1998; 97:2037 - 42; http://dx.doi.org/10.1161/01.CIR.97.20.2037; PMID: 9610534
- Tentolouris N, Argyrakopoulou G, Katsilambros N. Perturbed autonomic nervous system function in metabolic syndrome. Neuromolecular Med 2008; 10:169 - 78; http://dx.doi.org/10.1007/s12017-008-8022-5; PMID: 18224460
- Beske SD, Taylor JA. Obesity and autonomic function. Clin Auton Res 2001; 11:61 - 2; http://dx.doi.org/10.1007/BF02322046; PMID: 11570603
- Rossi M, Marti G, Ricordi L, Fornasari G, Finardi G, Fratino P, et al. Cardiac autonomic dysfunction in obese subjects. Clin Sci (Lond) 1989; 76:567 - 72; PMID: 2736876
- Piccirillo G, Vetta F, Viola E, Santagada E, Ronzoni S, Cacciafesta M, et al. Heart rate and blood pressure variability in obese normotensive subjects. Int J Obes Relat Metab Disord 1998; 22:741 - 50; http://dx.doi.org/10.1038/sj.ijo.0800650; PMID: 9725633
- Piccirillo G, Vetta F, Fimognari FL, Ronzoni S, Lama J, Cacciafesta M, et al. Power spectral analysis of heart rate variability in obese subjects: evidence of decreased cardiac sympathetic responsiveness. Int J Obes Relat Metab Disord 1996; 20:825 - 9; PMID: 8880349
- Porter TR, Eckberg DL, Fritsch JM, Rea RF, Beightol LA, Schmedtje JF Jr., et al. Autonomic pathophysiology in heart failure patients. Sympathetic-cholinergic interrelations. J Clin Invest 1990; 85:1362 - 71; http://dx.doi.org/10.1172/JCI114580; PMID: 2332495
- Nolan J, Flapan AD, Capewell S, MacDonald TM, Neilson JM, Ewing DJ. Decreased cardiac parasympathetic activity in chronic heart failure and its relation to left ventricular function. Br Heart J 1992; 67:482 - 5; http://dx.doi.org/10.1136/hrt.67.6.482; PMID: 1622699
- Eckberg DL, Drabinsky M, Braunwald E. Defective cardiac parasympathetic control in patients with heart disease. N Engl J Med 1971; 285:877 - 83; http://dx.doi.org/10.1056/NEJM197110142851602; PMID: 4398792
- Sroka K. On the genesis of myocardial ischemia. Z Kardiol 2004; 93:768 - 83; http://dx.doi.org/10.1007/s00392-004-0137-6; PMID: 15492892
- Watson AM, Hood SG, May CN. Mechanisms of sympathetic activation in heart failure. Clin Exp Pharmacol Physiol 2006; 33:1269 - 74; http://dx.doi.org/10.1111/j.1440-1681.2006.04523.x; PMID: 17184514
- La Rovere MT, Bigger JT Jr., Marcus FI, Mortara A, Schwartz PJ, ATRAMI (Autonomic Tone and Reflexes After Myocardial Infarction) Investigators. Baroreflex sensitivity and heart-rate variability in prediction of total cardiac mortality after myocardial infarction. Lancet 1998; 351:478 - 84; http://dx.doi.org/10.1016/S0140-6736(97)11144-8; PMID: 9482439
- Lechat P, Hulot JS, Escolano S, Mallet A, Leizorovicz A, Werhlen-Grandjean M, et al. Heart rate and cardiac rhythm relationships with bisoprolol benefit in chronic heart failure in CIBIS II Trial. Circulation 2001; 103:1428 - 33; http://dx.doi.org/10.1161/01.CIR.103.10.1428; PMID: 11245648
- Billman GE. A comprehensive review and analysis of 25 years of data from an in vivo canine model of sudden cardiac death: implications for future anti-arrhythmic drug development. Pharmacol Ther 2006; 111:808 - 35; http://dx.doi.org/10.1016/j.pharmthera.2006.01.002; PMID: 16483666
- Lara A, Damasceno DD, Pires R, Gros R, Gomes ER, Gavioli M, et al. Dysautonomia due to reduced cholinergic neurotransmission causes cardiac remodeling and heart failure. Mol Cell Biol 2010; 30:1746 - 56; http://dx.doi.org/10.1128/MCB.00996-09; PMID: 20123977
- De Ferrari GM, Vanoli E, Stramba-Badiale M, Hull SS Jr., Foreman RD, Schwartz PJ. Vagal reflexes and survival during acute myocardial ischemia in conscious dogs with healed myocardial infarction. Am J Physiol 1991; 261:H63 - 9; PMID: 1858931
- Vanoli E, De Ferrari GM, Stramba-Badiale M, Hull SS Jr., Foreman RD, Schwartz PJ. Vagal stimulation and prevention of sudden death in conscious dogs with a healed myocardial infarction. Circ Res 1991; 68:1471 - 81; http://dx.doi.org/10.1161/01.RES.68.5.1471; PMID: 2019002
- Zheng C, Li M, Inagaki M, Kawada T, Sunagawa K, Sugimachi M. Vagal stimulation markedly suppresses arrhythmias in conscious rats with chronic heart failure after myocardial infarction. Conf Proc IEEE Eng Med Biol Soc 2005; 7:7072 - 5; PMID: 17281904
- Li M, Zheng C, Sato T, Kawada T, Sugimachi M, Sunagawa K. Vagal nerve stimulation markedly improves long-term survival after chronic heart failure in rats. Circulation 2004; 109:120 - 4; http://dx.doi.org/10.1161/01.CIR.0000105721.71640.DA; PMID: 14662714
- Bibevski S, Dunlap ME. Prevention of diminished parasympathetic control of the heart in experimental heart failure. Am J Physiol Heart Circ Physiol 2004; 287:H1780 - 5; http://dx.doi.org/10.1152/ajpheart.00430.2004; PMID: 15191889
- De Ferrari GM, Crijns HJ, Borggrefe M, Milasinovic G, Smid J, Zabel M, et al, CardioFit Multicenter Trial Investigators. Chronic vagus nerve stimulation: a new and promising therapeutic approach for chronic heart failure. Eur Heart J 2011; 32:847 - 55; http://dx.doi.org/10.1093/eurheartj/ehq391; PMID: 21030409
- Sabbah HN. Electrical vagus nerve stimulation for the treatment of chronic heart failure. Cleve Clin J Med 2011; 78:Suppl 1 S24 - 9; http://dx.doi.org/10.3949/ccjm.78.s1.04; PMID: 21972326
- Dunlap ME, Bibevski S, Rosenberry TL, Ernsberger P. Mechanisms of altered vagal control in heart failure: influence of muscarinic receptors and acetylcholinesterase activity. Am J Physiol Heart Circ Physiol 2003; 285:H1632 - 40; PMID: 12829433
- Behling A, Moraes RS, Rohde LE, Ferlin EL, Nóbrega AC, Ribeiro JP. Cholinergic stimulation with pyridostigmine reduces ventricular arrhythmia and enhances heart rate variability in heart failure. Am Heart J 2003; 146:494 - 500; http://dx.doi.org/10.1016/S0002-8703(03)00319-3; PMID: 12947369
- Paton JF, Kasparov S, Paterson DJ. Nitric oxide and autonomic control of heart rate: a question of specificity. Trends Neurosci 2002; 25:626 - 31; http://dx.doi.org/10.1016/S0166-2236(02)02261-0; PMID: 12446130
- Nihei M, Lee JK, Honjo H, Yasui K, Uzzaman M, Kamiya K, et al. Decreased vagal control over heart rate in rats with right-sided congestive heart failure: downregulation of neuronal nitric oxide synthase. Circ J 2005; 69:493 - 9; http://dx.doi.org/10.1253/circj.69.493; PMID: 15791049
- Lynch SW, Braas KM, Harakall SA, Kennedy AL, Mawe GM, Parsons RL. Neuropeptide Y (NPY) expression is increased in explanted guinea pig parasympathetic cardiac ganglia neurons. Brain Res 1999; 827:70 - 8; http://dx.doi.org/10.1016/S0006-8993(99)01308-6; PMID: 10320695
- Girard BM, Young BA, Buttolph TR, White SL, Parsons RL. Regulation of neuronal pituitary adenylate cyclase-activating polypeptide expression during culture of guinea-pig cardiac ganglia. Neuroscience 2007; 146:584 - 93; http://dx.doi.org/10.1016/j.neuroscience.2007.02.001; PMID: 17367946
- Tompkins JD, Ardell JL, Hoover DB, Parsons RL. Neurally released pituitary adenylate cyclase-activating polypeptide enhances guinea pig intrinsic cardiac neurone excitability. J Physiol 2007; 582:87 - 93; http://dx.doi.org/10.1113/jphysiol.2007.134965; PMID: 17495034
- Arora RC, Cardinal R, Smith FM, Ardell JL, Dell’Italia LJ, Armour JA. Intrinsic cardiac nervous system in tachycardia induced heart failure. Am J Physiol Regul Integr Comp Physiol 2003; 285:R1212 - 23; PMID: 12893651
- Smith PG, Beauregard CL. Conversion of parasympathetic nerve function from prejunctional inhibition to postjunctional excitation following sympathectomy of rat periorbital smooth muscle. Brain Res 1993; 629:319 - 22; http://dx.doi.org/10.1016/0006-8993(93)91338-S; PMID: 7906603
- Moravec M, Moravec J, Forsgren S. Catecholaminergic and peptidergic nerve components of intramural ganglia in the rat heart. An immunohistochemical study. Cell Tissue Res 1990; 262:315 - 27; http://dx.doi.org/10.1007/BF00309887; PMID: 1706221
- Forsgren S, Moravec M, Moravec J. Catecholamine-synthesizing enzymes and neuropeptides in rat heart epicardial ganglia; an immunohistochemical study. Histochem J 1990; 22:667 - 76; http://dx.doi.org/10.1007/BF01047451; PMID: 1706694
- Slavíková J, Kuncová J, Reischig J, Dvoráková M. Catecholaminergic neurons in the rat intrinsic cardiac nervous system. Neurochem Res 2003; 28:593 - 8; http://dx.doi.org/10.1023/A:1022837810357; PMID: 12675149
- Bałuk P, Gabella G. Some parasympathetic neurons in the guinea-pig heart express aspects of the catecholaminergic phenotype in vivo. Cell Tissue Res 1990; 261:275 - 85; http://dx.doi.org/10.1007/BF00318669; PMID: 1976043
- Forsgren S, Moravec M, Moravec J. Catecholamine-synthesizing enzymes and neuropeptides in rat heart epicardial ganglia; an immunohistochemical study. Histochem J 1990; 22:667 - 76; http://dx.doi.org/10.1007/BF01047451; PMID: 1706694
- Horackova M, Armour JA, Byczko Z. Distribution of intrinsic cardiac neurons in whole-mount guinea pig atria identified by multiple neurochemical coding. A confocal microscope study. Cell Tissue Res 1999; 297:409 - 21; http://dx.doi.org/10.1007/s004410051368; PMID: 10460488
- Weihe E, Schütz B, Hartschuh W, Anlauf M, Schäfer MK, Eiden LE. Coexpression of cholinergic and noradrenergic phenotypes in human and nonhuman autonomic nervous system. J Comp Neurol 2005; 492:370 - 9; http://dx.doi.org/10.1002/cne.20745; PMID: 16217790
- Moravec J, Moravec M. Intrinsic nerve plexus of mammalian heart: morphological basis of cardiac rhythmical activity?. Int Rev Cytol 1987; 106:89 - 148; http://dx.doi.org/10.1016/S0074-7696(08)61711-8; PMID: 3294720
- Hoover DB, Isaacs ER, Jacques F, Hoard JL, Pagé P, Armour JA. Localization of multiple neurotransmitters in surgically derived specimens of human atrial ganglia. Neuroscience 2009; 164:1170 - 9; http://dx.doi.org/10.1016/j.neuroscience.2009.09.001; PMID: 19747529
- Armour JA. Functional anatomy of intrathoracic neurons innervating the atria and ventricles. Heart Rhythm 2010; 7:994 - 6; http://dx.doi.org/10.1016/j.hrthm.2010.02.014; PMID: 20156593
- Brodski C, Schnürch H, Dechant G. Neurotrophin-3 promotes the cholinergic differentiation of sympathetic neurons. Proc Natl Acad Sci U S A 2000; 97:9683 - 8; http://dx.doi.org/10.1073/pnas.160080697; PMID: 10931939
- Slonimsky JD, Mattaliano MD, Moon JI, Griffith LC, Birren SJ. Role for calcium/calmodulin-dependent protein kinase II in the p75-mediated regulation of sympathetic cholinergic transmission. Proc Natl Acad Sci U S A 2006; 103:2915 - 9; http://dx.doi.org/10.1073/pnas.0511276103; PMID: 16476997
- Slonimsky JD, Yang B, Hinterneder JM, Nokes EB, Birren SJ. BDNF and CNTF regulate cholinergic properties of sympathetic neurons through independent mechanisms. Mol Cell Neurosci 2003; 23:648 - 60; http://dx.doi.org/10.1016/S1044-7431(03)00102-7; PMID: 12932444
- Pongrac JL, Rylett RJ. Optimization of serum-free culture conditions for growth of embryonic rat cholinergic basal forebrain neurons. J Neurosci Methods 1998; 84:69 - 76; http://dx.doi.org/10.1016/S0165-0270(98)00099-5; PMID: 9821636
- Tuszynski MH, Mafong E, Meyer S. Central infusions of brain-derived neurotrophic factor and neurotrophin-4/5, but not nerve growth factor and neurotrophin-3, prevent loss of the cholinergic phenotype in injured adult motor neurons. Neuroscience 1996; 71:761 - 71; http://dx.doi.org/10.1016/0306-4522(95)00440-8; PMID: 8867048
- Apostolova G, Dorn R, Ka S, Hallböök F, Lundeberg J, Liser K, et al. Neurotransmitter phenotype-specific expression changes in developing sympathetic neurons. Mol Cell Neurosci 2007; 35:397 - 408; http://dx.doi.org/10.1016/j.mcn.2007.03.014; PMID: 17513123
- Brodski C, Schaubmar A, Dechant G. Opposing functions of GDNF and NGF in the development of cholinergic and noradrenergic sympathetic neurons. Mol Cell Neurosci 2002; 19:528 - 38; http://dx.doi.org/10.1006/mcne.2001.1093; PMID: 11988020
- Potter DD, Landis SC, Matsumoto SG, Furshpan EJ. Synaptic functions in rat sympathetic neurons in microcultures. II. Adrenergic/cholinergic dual status and plasticity. J Neurosci 1986; 6:1080 - 98; PMID: 3009730
- Felder E, Dechant G. Neurotrophic factors acutely alter the sorting of the vesicular acetyl choline transporter and the vesicular monoamine transporter 2 in bimodal sympathetic neurons. Mol Cell Neurosci 2007; 34:1 - 9; http://dx.doi.org/10.1016/j.mcn.2006.09.005; PMID: 17059887
- Yang B, Slonimsky JD, Birren SJ. A rapid switch in sympathetic neurotransmitter release properties mediated by the p75 receptor. Nat Neurosci 2002; 5:539 - 45; http://dx.doi.org/10.1038/nn0602-853; PMID: 11992117
- Burau K, Stenull I, Huber K, Misawa H, Berse B, Unsicker K, et al. c-ret regulates cholinergic properties in mouse sympathetic neurons: evidence from mutant mice. Eur J Neurosci 2004; 20:353 - 62; http://dx.doi.org/10.1111/j.1460-9568.2004.03500.x; PMID: 15233745