Abstract
Type I toxin-antitoxin (TA) systems are widespread in bacteria and consist of a toxin-encoding mRNA and a partially overlapping antisense RNA that blocks expression of the toxin, either at the level of translation or by mRNA degradation. Four type I toxin families have so far been proposed in B. subtilis based on sequence similarity: TxpA/BsrG, BsrH/BsrE, YonT and YhzE and two (TxpA and BsrG) have been studied in some detail. Here we review what is known about these confirmed and putative toxin-antitoxin families in B. subtilis, their regulatory mechanisms, their potential roles and how they may link to the physiology of the cell.
Introduction
Toxin-antitoxin systems are widespread in bacteria and have been classified according to three types I, II and III. Type I toxin loci typically encode a small hydrophobic toxic protein (less than 60 aa) containing a potential transmembrane domain. The antitoxin is an antisense RNA, which alters the stability and/or translation of the toxin mRNA (). In type II systems, the toxin proteins are generally longer (around 100 amino acids) and have endoribonuclease, protein kinase or phosphotransferase activity (for recent reviews see refs. Citation1 and Citation2). They can also generate double-strand breaks poisoning the DNA gyrase. In contrast to type I systems, type II antitoxins are proteins, which bind directly to the toxin and neutralize it. More recently, type III systems have been discoveredCitation3 and are a hybrid between type I and type II systems. Indeed, the only known example codes for a toxic protein having ribonuclease activity, like some type II toxins, but the antitoxin is an RNA, as for type I systems. The RNA binds to the toxin to neutralize it. A recent bioinformatics study allowed detection of more type III systems in Firmicutes, Fusobacteria and Proteobacteria.Citation4 In this review, we will focus on type I toxin-antitoxin systems in Bacillus subtilis.
Figure 1. Type I toxin-antitoxin chromosomal regions in B. subtilis. Toxins are shown as blue, potential toxins as hatched blue and antitoxins as red arrows, while ORFs in the neighbourhood of TA systems are shown as grey arrows. Prophages are indicated to the left.
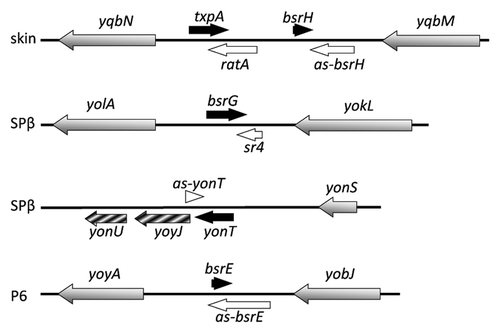
Type I toxin-antitoxin loci were first identified on plasmids and are required for their replication and maintenance. Thereafter, chromosomal toxins were discovered but their role remains unclear. In Gram-negative bacteria, several toxin families have been identified such as Ldr, Hok, TisB, ShoB and EHEC; all have predicted transmembrane domains. In Gram-positive bacteria, the first type I toxin-antitoxin system was identified on plasmid pAD1 of Enterococcus faecalis. This toxin belongs to the par family. The toxin (Fst) is encoded by RNA I and the antitoxin by RNA II. RNA I and RNA II overlap by 35 nucleotides at their 3′ ends and have complementary direct repeats at their 5′ ends.Citation5 Interaction between these direct repeats and their complementary sequences is important for translation repression. Moreover, RNA II is less stable than RNA I, which allows Fst to act as a post-segregational killing system (PSK), ensuring maintenance of pAD1 in cellsCitation6 (for more details, see K. Weaver, this issue). Recently, Fst-like toxin-antitoxin systems were also found on plasmids from Enterococcus faecalis, Lactobacillus curvatus and Staphylococcus aureus, the chromosome of Enterococcus faecalis, Lactobacillus casei and Staphylococcus saprophyticus and a phage from Lactobacillus gasseri.Citation7
The first type I toxin-antitoxin system identified on the chromosome of B. subtilis was txpA-ratA in 2005.Citation8 Five years later, 13 additional potential type I toxin-antitoxin cassettes were identified on the chromosome of B. subtilis by deep sequencingCitation9 and bioinformatics analyses.Citation10 These systems thus appear to be even more highly represented than type II toxin-antitoxin systems in this organism, underlining their importance. Toxins from B. subtilis range from 28 to 60 amino acids and are mostly located in prophage elements, except for those belonging to the YhzE family (see below). Three of the 14 have been shown to be toxic upon overexpression in either B. subtilis (TxpA, BsrG) or E. coli (YonT) and the others are proposed to behave similarly. Based on an alignment of the different toxins of B. subtilis (), four toxin families can be proposed: TxpA/BsrG, BsrH/BsrE, YonT and YheZ. BsrG is about 80% similar to the N-terminal half of TxpA, for example, while BsrE is about 90% similar to BsrH. An alignment of the YheZ family is shown in . In contrast to type II toxins, there is no shared type I toxin-antitoxin family between B. subtilis and Gram-negative bacteria, despite the fact that all of them are predicted to affect membrane integrity. We detail here recent work done to characterize the type I toxin-antitoxin systems of B. subtilis and show how some of them are important for cell physiology.
Figure 2. Alignment of type I toxins using ClustalW. Conserved amino acids are marked with an asterisk (*), strongly similar amino acids with a colon (:), and weakly similar amino acids with a full stop (.). The transmembrane domain predicted with TMpred (http://www.ch.embnet.org/software/TMPRED_form.html) is shaded; charged amino acids are in red.
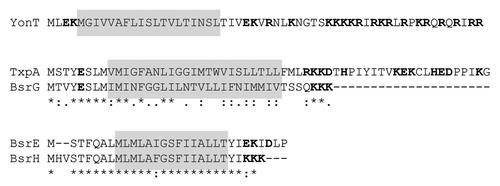
Type I Toxins in B. subtilis: The TxpA/BsrG Family
TxpA / RatA
The toxin-antitoxin module txpA-ratA is located on the skin prophage of B. subtilisCitation8 (). The txpA gene encodes a toxic protein of 59 amino acids.Citation8 This protein has a predicted transmembrane domain at its N-terminus and charged amino acids at the C-terminus ().
The RatA antisense RNA is 220 nucleotides long and overlaps the 3′ end of txpA mRNA by ~120 nucleotides (Durand et al., submitted). The RNAs can potentially interact by forming kissing complexes between two stem loops located near each of their 3′ ends (). After propagation of the base-pairing region to form an extended duplex, RNase III cleaves the duplex, leading to the degradation of both txpA and RatA (Durand et al., submitted). Degradation of txpA mRNA by RNase III is essential for B. subtilis viability. Indeed, strains lacking RNase III are non-viable due to the overexpression of two toxin mRNAs, txpA and yonT (see below). This result shows that the control of txpA mRNA stability plays a major role in the regulation of expression of this toxin. To ensure tight control of toxin expression, the level of RatA is 15-fold greater than that of txpA mRNA in B. subtilis (Durand et al., submitted). This excess of antisense RNA is cleaved by the endoribonuclease RNase Y, followed by degradation of the upstream and downstream fragments by PNPase and RNase J1 respectively (Durand et al., submitted).
Figure 4. Secondary structures of txpA, RatA and the txpA/RatA duplex. (A) Secondary structure of individual txpA and RatA RNAs, showing the potential loop-loop (kissing) interaction between stem-loop (SL) 6 of txpA and the transcription terminator (ter) of RatA, and between the terminator of txpA and SL3 of RatA (Durand et al, submitted). The start and stop codons of txpA are underlined and the Shine-Dalgarno (SD) sequence is indicated. (B) Secondary structure of extended duplex between txpA and RatA. Major sites of RNase III cleavage are indicated.
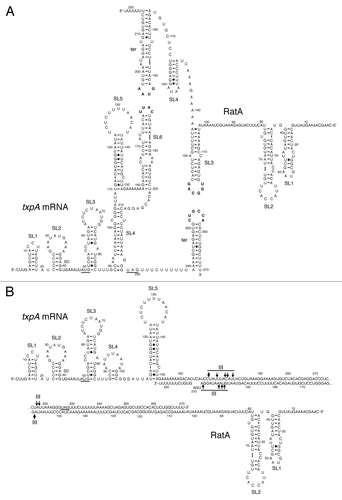
The TxpA toxin family is not only present in B. subtilis but also in other Bacilli and Clostridi.Citation10 A TxpA-related system is also found in a phage infecting Staphylococcus aureus (phage 42E). Although the N- and C-termini are variable in size and sequence, TxpA homologs have a similar hydrophobic domain followed by charged amino acids.
BsrG/SR4
The toxin-antitoxin module bsrG-sr4 is located on the SPβ prophage of the B. subtilis chromosomeCitation11 (). The bsrG RNA is 294 nt long and encodes a toxic peptide of 38 amino acidsCitation11 with a predicted transmembrane domain in the central part and charged amino acids at the C-terminus ().
The antisense RNA SR4 is 180 nucleotides long and overlaps the 3′ end of bsrG by 123 nucleotidesCitation11 (). The interaction of both RNAs at their 3′ ends promotes degradation of bsrG RNA. RNase III cleaves the bsrG RNA/SR4 duplex at position 185 of bsrG RNA, 8 nt downstream from the stop codon, but is not involved in the degradation of either bsrG RNA or SR4 alone.Citation11 Endonuclease Y and the 3′-5′ exoribonuclease R are responsible for further degradation of both RNAs. PNPase processes SR4 precursors into the mature RNA. The essential function of RNase III in B. subtilis was shown not to be related to the bsrG-sr4 TA system, since a Δrnc suppressor strain neither lysed on agar plates nor had mutations in the bsrG ORF.Citation11 This suppressor strain is now known to have a deletion of the skin prophage and a tendency to lose SPβ, to reduce TxpA and YonT toxicity (Durand et al., submitted). It is not excluded that SR4 not only directs bsrG RNA into the degradation pathway, but additionally inhibits bsrG translation. Preliminary secondary structure analyses of bsrG RNA and the bsrG-RNA /SR4 complex indicate structural alterations around the bsrG-ribosome binding site in the presence of SR4 (Jahn and Brantl, unpublished). These data have to be corroborated by toe-printing studies. Complex formation assays with wild-type bsrG RNA/SR4 yielded an apparent binding constant kapp of 7 x 105 M−1 s−1 (Jahn, unpublished), which is the same order of magnitude as those of other cis-encoded sense/antisense RNA pairs.Citation12 The sr4 promoter is about 6- to 10-fold stronger than the bsrG promoter, which should result in an excess of the antitoxin over the toxin,Citation11 as was seen for txpA-RatA. A potential ResD site is situated upstream of the bsrG promoter. ResD is a transcription factor induced upon oxygen stress in B. subtilis.Citation13
Figure 5. Nucleotide sequences of type I toxin-antitoxins. Only the toxin-encoding strand is shown. Mapped (txpA and bsrG) and predicted (bsrE and bsrH) -10 boxes of the promoters are in bold-type. Transcription start sites are indicated by arrows and transcription termination is indicated by “term”. The complementary region between the toxin and antitoxin RNAs is shaded in yellow. Start and stop codons are in red. Putative ResD binding sites are shaded in light-brown. The predicted SD sequences are boxed.
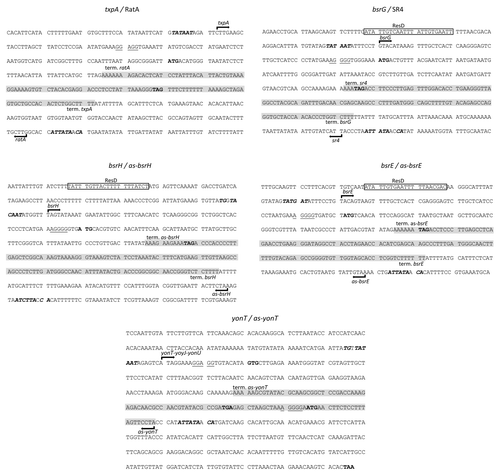
Type I Toxins in B. subtilis: The YonT Family
YonT/as-YonT
The toxin gene yonT and its antitoxin as-yonT were identified by bioinformatic analysisCitation10 and are located on the SPβ prophage (). YonT encodes a protein of 58 amino acids that is toxic when overexpressed in E. coli. This system differs from the other toxins previously described. Indeed, northern blot analysis of yonT shows a band of ~1 kb, suggesting that yonT is transcribed in an operon with the downstream genes yoyJ and yonU (Durand et al., submitted). A shorter band, likely to be a processed species of ~200 nucleotides, codes for just the YonT toxin (Durand et al., submitted).
Northern blots to detect the antisense RNA of the yonT transcript (as-yonT) revealed a band around 100 nucleotides in size,Citation10 consistent with transcription from a putative sigma A promoter that ends at the rho independent terminator found in this region (). This antisense RNA is half as long as those described previously. The as-yonT RNA is complementary to both the 3′ end of yonT and the 5′ end of yoyJ, raising the question of the impact of this antisense RNA on the expression of the downstream yoyJ and yonU genes and whether these might also encode toxins. Like txpA, the yonT mRNA is stabilized upon RNase III depletion. Deletion of these two toxins mRNAs is sufficient to render the RNase III dispensable in B. subtilis (Durand et al., submitted).
Putative Type I Toxins in B. subtilis: the BsrH/E Family
BsrH/as-BsrH
The bsrH locus was originally identified by Saito et al.Citation14 as encoding a small regulatory RNA. Following a deep-sequencing analysis, Irnov et al. subsequently proposed that this locus encodes a type I toxin-antitoxin system (bsrH/as-bsrH).Citation9 It is located in the same intergenic region as txpA/ratA in the skin prophage (). The bsrH mRNA encodes a small 29 amino acid peptide. Interestingly, like bsrG, a potential ResD binding site has been found, located around 100 nucleotides upstream of the predicted transcription start site of bsrH ().
The antisense RNA (as-bsrH) is 200 nucleotides long and overlaps bsrH at its 3′ end by approximately 120 nucleotides. In contrast to txpA, bsrH is insensitive to RNase III and Y, but increased levels of bsrH mRNA are detected in RNase J1 and RNase R mutant strains and as-bsrH in an RNase PH mutant strain, suggesting that these RNAs are degraded principally by an exoribonucleolytic mechanism (Durand et al. unpublished result). Although the TxpA and BsrH type I toxins are both encoded in the same intergenic region, the regulation of their turnover is clearly different. This may indicate that the structure of the brsH/as-bsrH duplex is different from that of other TA systems in B. subtilis. It remains to be seen whether as-BsrH affects bsrH stability, or translation, as observed for the Fst toxin of E. faecalis.
BsrE/as-BsrE
The BsrE sRNA was also originally identified by Saito et al.Citation14 Subsequent deep-sequencing analysis confirmed the detection of the BsrE RNA and a partially complementary RNA,Citation9 which encodes a potential toxin, based on sequence homology. To avoid confusion and keep a consistent nomenclature, we propose that the toxin-encoding strand be renamed bsrE and its putative regulatory antisense RNA as-BsrE. The bsrE toxin gene is located in the P6 prophage and encodes a peptide of 30 amino acids (). The amino acid sequence of BsrE is highly similar to BsrH () and, as for the bsrH and bsrG genes, a potential ResD binding site has been detected at position -54 ().Citation9
A northern blot of the regulatory antisense RNA (as-bsrE) reveals two bands at ~190 and 280 nucleotides.Citation9 The band at 190 nucleotides corresponds to the predicted distance between a putative rho independent terminator and a sigma A promoter (). The minor band at 280 nucleotides could be a read-through product from the upstream gene or due to a termination defect.Citation9 The two RNAs overlap by ~100 nucleotides at their 3′ ends.
Putative Type I Toxins in B. subtilis: The YhzE (SscA) Family
Two genes located in the same intergenic region, yhzE (sscA) and yhzE-2, have been proposed to encode toxin proteins by bioinformatic analysis.Citation10 They are under the control of a sporulation specific sigma K promoter and have a putative binding site for the transcription factor GerE, necessary for the expression of late spore coat genes.Citation15
An antisense RNA of ~120 nucleotides to the 3′ end of yhzE-2 gene was detected by northern blotting. YhzE-2 does not seem to be toxic in E. coli or B. subtilis, at least under the conditions of overexpression tested by Fozo et al.Citation10 More recently, a study from Kodoma et al. showed that yhzE (sscA) is involved in spore germination and spore coat assembly.Citation15
Interestingly, B. subtilis encodes 7 other paralogs of the YhzE proteins encoded by the yosA, yjcZ, yoyG, yczM, yczN, yuzJ and ykzV genes. Some of them are duplicated in the same intergenic region, such as yhzE and yhzE-2 and yczN and yczM. These proteins have a conserved hydrophobic region and variable C-terminal and N-terminal domains. The N-terminus is always rich in glycines and aromatic residues (). All these genes have a putative sigma K promoter and a recent tiling array study shows an increase of their expression during sporulation.Citation16 Only yhzE-2 10 and possibly ykzV, yjcZCitation17 and yosA,Citation16 have an antisense RNA detectable by tiling array. Therefore, it remains to be seen whether these are true type I toxin-antitoxin systems or whether they encode simply related but non-toxic peptides with a constructive role in sporulation. Other proteins of the same family have also been detected by BLAST in several bacteria; all of them are spore-forming bacteria belonging to the genus Bacillus or Clostridium.Citation10
Regulation of Toxin Expression by the Antisense RNA
In Gram-negative bacteria, the antisense RNA generally base-pairs with the 5′ end of the toxin mRNA. The only exception described so far are the Sibs RNAs, which overlap the toxin mRNAs (Ibs) completely.Citation18 Interestingly, in B. subtilis and other Gram-positive bacteria, the antisense RNAs of all known type I toxin-antitoxin systems are complementary to the 3′ end of their target RNAs (for review, see refs Citation12, Citation19 and Citation20) (). Why do antitoxins in B. subtilis preferentially target the 3′ ends of toxin mRNAs? One explanation could be the fact that the degradation machinery in Gram-positives is quite different from that of Gram-negative bacteria.Citation21 Indeed, B. subtilis possesses a 5′-3′ exoribonuclease activity, which plays a major role in mRNA degradation.Citation17 Blocking this 5′-3′ mRNA degradation pathway by a pairing with an antisense RNA could be detrimental in some cases.
Even among antitoxins that base-pair with the 3′ end of the toxin mRNA, the regulatory outcome is not the same. The primary effect of RNA II on RNA I of pAD1 plasmid of E. faecalis is inhibition of Fst translation.Citation22 Both RNAs possess complementary direct repeats at their 5′ ends, which block ribosome access by pairing. Neither txpA mRNA nor bsrG mRNAs have such repeats and pairing with their cognate antitoxins provokes mRNA degradation through RNase III cleavages (see above; Durand et al., submitted and ref. Citation11). A study on the regulation of bsrH by as-bsrH may reveal yet another mechanism of regulation, since bsrH is insensitive to the endoribonucleases III and Y and both RNAs are degraded by 3′-5′ exoribonucleases (see above). This result raises the question how these RNAs can be attacked by 3′-5′ exoribonucleases when their 3′ ends are base-paired? These observations highlight the diversity of strategies used by antisense RNA to regulate toxin expression and a careful analysis of toxin-antitoxin sequences may allow educated guesses as to the regulatory mechanism of the antitoxin RNA.
B. subtilis TxpA, BsrG, BsrE and BsrH toxins also share a common feature with the Fst toxin family. Indeed, they all have a potential secondary structure sequestering the SD sequence that likely reduces translation initiation. It was shown for the Fst toxin that the structure between the SD and its complementary sequence is necessary to allow efficient regulation by the antisense RNA (RNA II).Citation22 YonT does not have this structure to trap the SD sequence but has a GUG start codon, which could sufficiently reduce translation to permit base-pairing with its antitoxin. Furthermore, the SD sequences for txpA and yonT mRNAs have the two most extensive complementarities to the anti-SD of 16S rRNA among all mRNAs in B. subtilis, with 12 and 11 potential base-pairs, respectively.Citation23 Extensive base-pairing between the 30S ribosomal subunit and internal ribosome binding sites has been shown to lead to ribosome pausing.Citation24 In the same manner, these “perfect” SD sequences, which are predicted to be extremely efficient at recruiting ribosomes are likely to have slow ribosome release rates. Thus poor translation initiation may be an important feature for these toxins to allow pairing of the antitoxin RNA to the 3′ end.
Deletion of the txpA and bsrG asRNAs is toxic for the cell. Indeed, RatA mutants undergo colony lysis after few days and SR4 mutants lyse overnight on agar plates.Citation8,Citation11 In contrast, deletion of most of the antisense RNAs of type I toxins in Gram-negatives shows no obvious phenotype. It is possible that type I toxins in B. subtilis cause more damage to the cell membrane and/or the basal expression level is higher in these organisms, requiring a tight regulation by the antisense RNA to repress them even in non-stressful environments.
Potential Role of Helper Proteins
In Gram-negative bacteria, a number of trans-encoded base-pairing sRNAs require the abundant RNA chaperone Hfq either for their stability or the interaction with their target RNAs.Citation25 No cis-encoded bacterial sRNA has been found that needs Hfq for its function. In Gram-positive bacteria, there is so far only one case where a role of Hfq for the promotion of sense/antisense RNA interaction was found.Citation26 Although neither bsrG-sr4 nor txpA-ratA seem to depend on Hfq for the control mechanism,Citation8,Citation11 the hybridization region between these two RNAs co-immuniprecipitates with Hfq (W. Winkler personal communication). Indeed this obervation can be extended to the bsrE/as-bsrE and BsrH/as-bsrH TA systems. However, the functional consequences of these interactions are not known. It cannot be entirely excluded that Hfq might be needed to open up secondary structures that prevent translation of some of the toxin mRNAs, as has been found for ahrC RNA regulated by SR1 in B. subtilis.Citation27
Functions of Type I Toxin-Antitoxin Systems
Type I toxin-antitoxins are widespread in bacteria but their function is still unclear. Two type I toxins in E. coli, TisB and SymE, have been shown to be induced by the SOS responseCitation28,Citation29 and TisB to play a role in persistence.Citation30
In B. subtilis, the YhzE protein family seems to be important during sporulation but as mentioned above, it remains to be seen whether these are true type I toxins. The other toxins described previously (TxpA, BsrG, BsrE, BsrH and YonT) are all located in prophages. They contain N-terminal transmembrane domains and charged amino acids at their C-termini (). They are probably localized to the membrane and could act similarly to phage holins, which may have been their primary function when the prophage was still functional.
Why maintain toxin genes on the chromosome? It has been suggested that TxpA helps to maintain the skin element in B. subtilis genome. The skin prophage is excised from the chromosome by a DNA rearrangement during sporulation.Citation31,Citation32 This excision allows reconstitution of a functional sigK gene, a transcription factor controlling mother-cell-specific gene expression at a late stage of sporulation. In this case, TxpA would have a similar function to the post-segregational killing (PSK) system of pAD1 (Fst toxin) of E. faecalis. Curiously, however, the PBSX prophage appears to be maintained on the chromosome of B. subtilis without a type I toxin-antitoxin system. Indeed, the PBSX prophage of B. subtilis is highly similar to the skin element,Citation33 but the intergenic region encoding the TxpA and BsrH toxins in skin is absent in PBSX.
It is also possible that toxins bring advantages to the cell during stress conditions, as observed for some type II toxin-antitoxins or TisB. All toxin-antitoxin modules encoded in B. subtilis prophages are under the control of a sigma A promoter, allowing rapid expression of toxins under defined conditions. One way to express toxins efficiently is to perturb the balance between toxin and antitoxin synthesis through transcriptional activators. Identification of these factors could give some clues about each toxin's function. For example, the bsrG, bsrE and bsrH genes have putative ResD binding sites upstream, suggesting expression of the toxin under conditions of oxygen limitation. In agreement with this hypothesis, a recent tiling array study by Nicolas et al.Citation16 shows increased expression of the three toxin mRNAs in cultures where oxygen is limiting (cultures without shaking) and a reduction of expression upon oxidative stress following paraquat, diamide or H2O2 treatment. One function of these toxins could therefore be to cause bacteriostasis to limit oxygen consumption during oxygen limitation. In the same study, expression of the txpA mRNA seems to be phosphate dependent. Moreover, the mRNA levels of txpA, bsrE, bsrG, bsrH and yonT increase once glucose is exhausted in the medium, linking these toxins to the metabolic state of the cell.Citation11,Citation16 Similarly, a heat shock at 48°C results in 3- to 4-fold faster degradation of bsrG RNA,Citation11 whereas SR4 is not affected. Upon increasing temperature, the toxin RNA might refold into a structure that is more accessible to certain endonucleases. The biological significance of the heat-induced degradation of bsrG RNA is still unclear, but once again there appears to be strong link between toxin expression and cell physiology.
Disclosure of Potential Conflicts of Interest
No potential conflicts of interest were disclosed.
Acknowledgments
This study was supported by the Agence Nationale de la Recherche (subtilRNA2 and asSUPYCO) to C.C. and by grant BR1552/7-2 of the priority program SPP1258 from the Deutsche Forschungsgemeinschaft to S.B.
References
- Van Melderen L, Saavedra De Bast M. Bacterial toxin-antitoxin systems: more than selfish entities?. PLoS Genet 2009; 5:e1000437; http://dx.doi.org/10.1371/journal.pgen.1000437; PMID: 19325885
- Van Melderen L. Toxin-antitoxin systems: why so many, what for?. Curr Opin Microbiol 2010; 13:781 - 5; http://dx.doi.org/10.1016/j.mib.2010.10.006; PMID: 21041110
- Fineran PC, Blower TR, Foulds IJ, Humphreys DP, Lilley KS, Salmond GP. The phage abortive infection system, ToxIN, functions as a protein-RNA toxin-antitoxin pair. Proc Natl Acad Sci U S A 2009; 106:894 - 9; http://dx.doi.org/10.1073/pnas.0808832106; PMID: 19124776
- Blower TR, Short FL, Rao F, Mizuguchi K, Pei XY, Fineran PC, et al. Identification and classification of bacterial Type III toxin-antitoxin systems encoded in chromosomal and plasmid genomes. Nucleic Acids Res 2012; 40:6158 - 73; http://dx.doi.org/10.1093/nar/gks231; PMID: 22434880
- Greenfield TJ, Weaver KE. Antisense RNA regulation of the pAD1 par post-segregational killing system requires interaction at the 5′ and 3′ ends of the RNAs. Mol Microbiol 2000; 37:661 - 70; http://dx.doi.org/10.1046/j.1365-2958.2000.02034.x; PMID: 10931359
- Weaver KE, Jensen KD, Colwell A, Sriram SI. Functional analysis of the Enterococcus faecalis plasmid pAD1-encoded stability determinant par. Mol Microbiol 1996; 20:53 - 63; http://dx.doi.org/10.1111/j.1365-2958.1996.tb02488.x; PMID: 8861204
- Weaver KE, Reddy SG, Brinkman CL, Patel S, Bayles KW, Endres JL. Identification and characterization of a family of toxin-antitoxin systems related to the Enterococcus faecalis plasmid pAD1 par addiction module. Microbiology 2009; 155:2930 - 40; http://dx.doi.org/10.1099/mic.0.030932-0; PMID: 19542006
- Silvaggi JM, Perkins JB, Losick R. Small untranslated RNA antitoxin in Bacillus subtilis.. J Bacteriol 2005; 187:6641 - 50; http://dx.doi.org/10.1128/JB.187.19.6641-6650.2005; PMID: 16166525
- Irnov I, Sharma CM, Vogel J, Winkler WC. Identification of regulatory RNAs in Bacillus subtilis.. Nucleic Acids Res 2010; 38:6637 - 51; http://dx.doi.org/10.1093/nar/gkq454; PMID: 20525796
- Fozo EM, Makarova KS, Shabalina SA, Yutin N, Koonin EV, Storz G. Abundance of type I toxin-antitoxin systems in bacteria: searches for new candidates and discovery of novel families. Nucleic Acids Res 2010; 38:3743 - 59; http://dx.doi.org/10.1093/nar/gkq054; PMID: 20156992
- Jahn N, Preis H, Wiedemann C, Brantl S. BsrG/SR4 from Bacillus subtilis--the first temperature-dependent type I toxin-antitoxin system. Mol Microbiol 2012; 83:579 - 98; http://dx.doi.org/10.1111/j.1365-2958.2011.07952.x; PMID: 22229825
- Brantl S. Regulatory mechanisms employed by cis-encoded antisense RNAs. Curr Opin Microbiol 2007; 10:102 - 9; http://dx.doi.org/10.1016/j.mib.2007.03.012; PMID: 17387036
- Nakano MM, Zuber P, Glaser P, Danchin A, Hulett FM. Two-component regulatory proteins ResD-ResE are required for transcriptional activation of fnr upon oxygen limitation in Bacillus subtilis.. J Bacteriol 1996; 178:3796 - 802; PMID: 8682783
- Saito S, Kakeshita H, Nakamura K. Novel small RNA-encoding genes in the intergenic regions of Bacillus subtilis.. Gene 2009; 428:2 - 8; http://dx.doi.org/10.1016/j.gene.2008.09.024; PMID: 18948176
- Kodama T, Matsubayashi T, Yanagihara T, Komoto H, Ara K, Ozaki K, et al. A novel small protein of Bacillus subtilis involved in spore germination and spore coat assembly. Biosci Biotechnol Biochem 2011; 75:1119 - 28; http://dx.doi.org/10.1271/bbb.110029; PMID: 21670523
- Nicolas P, Mäder U, Dervyn E, Rochat T, Leduc A, Pigeonneau N, et al. Condition-dependent transcriptome reveals high-level regulatory architecture in Bacillus subtilis.. Science 2012; 335:1103 - 6; http://dx.doi.org/10.1126/science.1206848; PMID: 22383849
- Durand S, Gilet L, Bessières P, Nicolas P, Condon C. Three essential ribonucleases-RNase Y, J1 and III-control the abundance of a majority of Bacillus subtilis mRNAs. PLoS Genet 2012; 8:e1002520; http://dx.doi.org/10.1371/journal.pgen.1002520; PMID: 22412379
- Fozo EM, Kawano M, Fontaine F, Kaya Y, Mendieta KS, Jones KL, et al. Repression of small toxic protein synthesis by the Sib and OhsC small RNAs. Mol Microbiol 2008; 70:1076 - 93; http://dx.doi.org/10.1111/j.1365-2958.2008.06394.x; PMID: 18710431
- Gerdes K, Wagner EG. RNA antitoxins. Curr Opin Microbiol 2007; 10:117 - 24; http://dx.doi.org/10.1016/j.mib.2007.03.003; PMID: 17376733
- Fozo EM, Hemm MR, Storz G. Small toxic proteins and the antisense RNAs that repress them. Microbiol Mol Biol Rev 2008; 72:579 - 89; http://dx.doi.org/10.1128/MMBR.00025-08; PMID: 19052321
- Condon C, Bechhofer DH. Regulated RNA stability in the Gram positives. Curr Opin Microbiol 2011; 14:148 - 54; http://dx.doi.org/10.1016/j.mib.2011.01.010; PMID: 21334965
- Shokeen S, Patel S, Greenfield TJ, Brinkman C, Weaver KE. Translational regulation by an intramolecular stem-loop is required for intermolecular RNA regulation of the par addiction module. J Bacteriol 2008; 190:6076 - 83; http://dx.doi.org/10.1128/JB.00660-08; PMID: 18641135
- Daou-Chabo R, Mathy N, Bénard L, Condon C. Ribosomes initiating translation of the hbs mRNA protect it from 5′-to-3′ exoribonucleolytic degradation by RNase J1. Mol Microbiol 2009; 71:1538 - 50; http://dx.doi.org/10.1111/j.1365-2958.2009.06620.x; PMID: 19210617
- Li GW, Oh E, Weissman JS. The anti-Shine-Dalgarno sequence drives translational pausing and codon choice in bacteria. Nature 2012; 484:538 - 41; http://dx.doi.org/10.1038/nature10965; PMID: 22456704
- Hajnsdorf E, Boni IV. Multiple activities of RNA-binding proteins S1 and Hfq. Biochimie 2012; 94:1544 - 53; http://dx.doi.org/10.1016/j.biochi.2012.02.010; PMID: 22370051
- Nielsen JS, Lei LK, Ebersbach T, Olsen AS, Klitgaard JK, Valentin-Hansen P, et al. Defining a role for Hfq in Gram-positive bacteria: evidence for Hfq-dependent antisense regulation in Listeria monocytogenes.. Nucleic Acids Res 2010; 38:907 - 19; http://dx.doi.org/10.1093/nar/gkp1081; PMID: 19942685
- Heidrich N, Moll I, Brantl S. In vitro analysis of the interaction between the small RNA SR1 and its primary target ahrC mRNA. Nucleic Acids Res 2007; 35:4331 - 46; http://dx.doi.org/10.1093/nar/gkm439; PMID: 17576690
- Vogel J, Argaman L, Wagner EG, Altuvia S. The small RNA IstR inhibits synthesis of an SOS-induced toxic peptide. Curr Biol 2004; 14:2271 - 6; http://dx.doi.org/10.1016/j.cub.2004.12.003; PMID: 15620655
- Kawano M, Aravind L, Storz G. An antisense RNA controls synthesis of an SOS-induced toxin evolved from an antitoxin. Mol Microbiol 2007; 64:738 - 54; http://dx.doi.org/10.1111/j.1365-2958.2007.05688.x; PMID: 17462020
- Dörr T, Vulić M, Lewis K. Ciprofloxacin causes persister formation by inducing the TisB toxin in Escherichia coli.. PLoS Biol 2010; 8:e1000317; http://dx.doi.org/10.1371/journal.pbio.1000317; PMID: 20186264
- Kunkel B, Losick R, Stragier P. The Bacillus subtilis gene for the development transcription factor sigma K is generated by excision of a dispensable DNA element containing a sporulation recombinase gene. Genes Dev 1990; 4:525 - 35; http://dx.doi.org/10.1101/gad.4.4.525; PMID: 2163341
- Popham DL, Stragier P. Binding of the Bacillus subtilis spoIVCA product to the recombination sites of the element interrupting the sigma K-encoding gene. Proc Natl Acad Sci U S A 1992; 89:5991 - 5; http://dx.doi.org/10.1073/pnas.89.13.5991; PMID: 1631085
- Krogh S, O’Reilly M, Nolan N, Devine KM. The phage-like element PBSX and part of the skin element, which are resident at different locations on the Bacillus subtilis chromosome, are highly homologous. Microbiology 1996; 142:2031 - 40; http://dx.doi.org/10.1099/13500872-142-8-2031; PMID: 8760915