Abstract
The Rac and Cdc42 GTPases as well as the multiple GTP exchange factors that regulate their activity have been implicated in the pathways that drive actin cytoskeleton reorganization, but the individual contributions of these molecules to cell migration remain unknown. Studies shown here examine the roles of CED-10/Rac, MIG-2/RhoG and CDC-42 in the migration of the QL and QR neuroblasts in C. elegans. CED-10/Rac was found to normally limit protrusion and migration, whereas MIG-2/RhoG was required for protrusion and migration. CED-10/Rac and MIG-2/RhoG also had redundant roles in Q protrusion and migration. Surprisingly, CDC-42 was found to have only weak effects on the protrusion and the migration. We found that a mutation of unc-73/Trio, which encodes a GEF for CED-10/Rac and MIG-2/RhoG, caused protrusions that were thin and filopodia-like, suggesting that UNC-73/Trio is required for robust lamellipodia-like protrusion. A screen of the 19 C. elegans Dbl homology Rho GEF genes revealed that PIX-1 was required for proper Q neuroblast protrusion and migration. Genetic analysis indicated that PIX-1 might act in the CED-10/Rac pathway in parallel to MIG-2/RhoG, and that PIX-1 has redundant function with UNC-73/Trio in Q neuroblast protrusion and migration. These results indicate that Rho GTPases and GEFs have both unique and overlapping roles in neuronal migration.
Introduction
The development of the vertebrate nervous system requires extensive neuronal migration. These neurons must migrate away from their birthplaces to form the various portions of the central nervous system. In order for these migrations to occur, numerous pathways must be activated in order for cells to be able to determine the proper pathway of migration, provide the motile force, and identify the precise stopping point of these migrations. The motile force necessary for these migrations is supplied through the reorganization of the actin cytoskeleton.Citation1 One family of proteins that has been repeatedly implicated in modulation of the cytoskeleton is the Rho subfamily of GTPases, including Rho, Rac and Cdc42.Citation2–Citation4
Small GTPases can act as molecular switches, cycling between active and inactive states. These GTPases are in an active state when bound to GTP and inactive when bound to GDP. Several molecules work with the small GTPases to modulate their activity. Guanine nucleotide exchange factors (GEFs) interact with the small GTPases to facilitate the exchange of GDP for GTP, which in turn activates the GTPases. GTPase-activating proteins (GAPs) act in an opposite manner, inactivating the GTPases by activating the GTPase activity of these small GTPases, leading to the hydrolysis of GTP to GDP. Small GTPases have been found to interact with a wide variety of effector proteins and GEFs and GAPs are able to specifically control the activation of an extensive number of signaling pathways through their interactions with these small GTPases. The C. elegans genome encodes 19 Dbl-homology (DH) GEF molecules and vertebrate genomes encode many more. A central unanswered question in Rho GTPase signaling involves the roles of the multiple GTPases and their many GEF regulators in developmental events.
Many studies have been performed examining the roles of the Rac GTPases and Cdc42 in cell motility.Citation2–Citation4 Rac GTPases and Cdc42 have been found to interact with effector proteins that then modulate the actin cytoskeleton, allowing for directed migration. Rac GTPases and CDC-42 utilize distinct effectors to control cytoskeletal organization. Cdc42 has been shown to directly bind to members of the Wiskcott-Aldrich Syndrome Protein (WASP) family, leading to the activation of the Arp2/3 complex that initiates the addition of branched actin filaments at the leading edge of the cell.Citation5–Citation9 Additionally, Cdc42 has been found to be required for filopodia extension and cell polarity necessary for directed cell movement.Citation10,Citation11 Likewise, numerous reports have found that the Rac GTPases lead to the generation of membrane ruffling necessary for the advancement of migrating cells.Citation10
Most of these studies describing the roles of these small GTPases in cell migration have been performed in cell culture with constitutively active and dominant negative forms of the Rac GTPases and Cdc42.Citation10 The roles of Rho-family GTPases and their GEFs are relatively uncharacterized in neuronal migration in vivo. Furthermore, the distinct roles of the multiple Rho GEF proteins on GTPase function in cell migration remain mysterious.
Here we examine the endogenous functions of the Rho GTPases CED-10/Rac, MIG-2/RhoG and CDC-42, as well as two Rho GEFs, in neuroblast migration in the nematode Caenorhabditis elegans. Using in vivo loss of function analyses, we found individual, opposing roles for these GTPases in the migrations of the Q neuroblasts. CED-10/Rac appears to be required for limiting the protrusions and migrations of the Q neuroblasts, whereas MIG-2/RhoG and CDC-42 are required for the ability of the Q neuroblasts to migrate. We also found that CED-10/Rac and MIG-2/RhoG have redundant roles in the ability of the Q cells to extend protrusions and to migrate.
We found that the Rac GEF UNC-73/Trio and putative Rac GEF PIX-1 have distinct roles in Q neuroblast polarization and migration. UNC-73/Trio is involved in both migration of neurons and the extension of neurites in C. elegans.Citation12–Citation15 UNC-73/Trio consists of numerous domains, including two GEF domains, with the first of which thought to interact solely with Rac proteins.Citation13 As such, UNC-73/Trio works upstream of the Rac GTPases CED-10/Rac and MIG-2/RhoG in axon pathfinding and neuronal migration.Citation12,Citation13 Additionally, studies examining UNC-73/Trio in the Q neuroblasts have found that UNC-73/Trio is required for Q neuroblast protrusion and migration.Citation16 PIX molecules have Rac GEF activity.Citation17 In C. elegans, PIX-1 acts in parallel to CED-10 and MIG-2 in gonad morphogenesis.Citation18
We found that unc-73 mutant Q cells extended protrusions, but these were thinner and had a more finger-like, filopodial character compared to the lamellipodial character of wild type. Thus, UNC-73/Trio might specifically mediate lamellipodial protrusion. Examination of the other DH containing GEFs revealed that PIX-1 also plays a role in the migration of the Q neuroblasts and descendants. Double mutant analysis of pix-1 with ced-10 and mig-2 found that PIX-1 might be working upstream of CED-10/Rac, but in parallel to MIG-2/RhoG in the protrusion and migration of the Q neuroblasts. pix-1 double mutants often lacked protrusion, similar to ced-10;mig-2 doubles. The filopodial-like extensions seen in unc-73 mutants were not observed in pix-1 mutants.
In sum, our results indicate that Rho GTPases and their GEFs have both distinct and redundant functions in neuroblast migration, indicating that loss of function analysis can reveal the discrete roles of the multiple Rho GTPases and their GEFs in developmental events.
Results
Q neuroblasts extend protrusions and migrate in opposite directions.
The Q neuroblasts make an ideal system in which to study neuroblast polarization, extension of lamellipodial and filopodial protrusions and migration.Citation16,Citation21 During embryogenesis, the bilateral Q neuroblasts are born between the V4 and V5 seam cells and are the sister cells of the V5 seam cellCitation23,Citation24 (). At hatching in L1 larvae, the Q neuroblasts are rounded, unpolarized cells. Between 1–2.5 h after hatching, the Q neuroblasts polarize in opposite directions by extending robust protrusions in opposite directions, with the QR neuroblast sending out protrusions anteriorly over the V4R seam cell and the QL neuroblast sending out protrusions posteriorly over the V5L seam cell ( and B). Between 3–3.5 h after hatching, the Q neuroblast cell bodies then follow these protrusions and migrate over the adjacent seam cells. Between 4–4.5 h after hatching, the Q cells have completed their migrations and undergo their first division, with the QR and QL neuroblasts dividing atop the V4R and V5L seam cells, respectively ( and D). These polarizations, protrusion extensions, and migrations are highly characteristic in wild-type animals, with very little variation from animal to animal ( and F).
After the initial divisions of the Q neuroblasts, the daughter cells continue migrating and undergo additional rounds of division and programmed cell death to ultimately produce three neurons each, with QL producing SDQL, PVM and PQR and QR producing SDQR, AVM and AQR.Citation24,Citation25 Of these neurons, AQR and PQR are the descendants that migrate the farthest, with AQR migrating into the anterior deirid ganglion in the head and PQR migrating into the lumbar ganglion in the tailCitation21,Citation24,Citation25 (). Observations of wild-type AQR and PQR migrations again showed that the migrations of the Q cell lineage display little deviation in the final locations of these neurons after migration. AQR always migrated to the head region of the animal near the second pharyngeal bulb near the anterior deirid ganglion and PQR always migrated to the tail of the animal behind the anus in the lumbar ganglion ().
CED-10/Rac limits Q neuroblast protrusion whereas MIG-2/RhoG is required for robust Q neuroblast protrusion.
To determine if the Rac GTPases and CDC-42 are involved in Q neuroblast polarization, protrusion or migration, the protrusions of the Q cells were examined in each of ced-10(n1993), mig-2(mu28) and cdc-42(gk388M+) single mutants. ced-10(n1993) is a hypomorphic loss of function mutation;Citation26 mig-2(mu28) is a null mutation;Citation27 and cdc-42(gk388) is a deletion that removes the predicted translational start site and initiator codon (data not shown and Wormbase) and first exon and is likely a null. The positions and shapes of the V cells were largely normal in ced-10(n1993), mig-2(mu28) and cdc-42(gk388M+). ced-10(n1993) did not strongly affect direction of polarization or protrusion, as all of the observed Q neuroblasts at 1–1.5 h after hatching had extended robust protrusions in their respective directions in the ced-10(n1993) mutants (). However, in 5% of ced-10(n1993) mutants, the QL protrusion displayed over extension; the QL protrusion extended completely over V5 and touched V6, a phenotype never observed in wild type ().
MIG-2/RhoG has previously been shown to affect Q descendant migration.Citation27,Citation28 Furthermore, a mutation in MIG-2 predicted to constitutively activate MIG-2 signaling (rh17;G15R)Citation27 caused a reduction in the speed of migration of the Q cells.Citation29 To determine the consequence of loss of function of mig-2 on Q cell migration, we analyzed the null allele mig-2(mu28). mig-2(mu28) did not affect direction of polarization but did cause weak defects in protrusion, with a small but significant proportion of both QL and QR failing to extend protrusions in any direction at 1–1.5 h after hatching (). For an example of a protrusion defect similar those seen in mig-2(mu28), see the mig-2;ced-10 in . Wild-type Q cells never failed to extend protrusions in this manner.
All of the Q cells in the cdc-42(gk388M+) mutants extended protrusions in the correct direction, though in a small percentage the protrusions were shorter and less robust than those of wild type and did not extend greater than half of the distance over the respective seam cell (weakly polarized versus strongly polarized; and ). These data indicate that CED-10/Rac, MIG-2/RhoG and CDC-42 are not involved in the direction of protrusion of the Q neuroblasts. While the effects were weak, these data also indicate that MIG-2/RhoG and to a lesser extent CDC-42 are required for robust Q neuroblast protrusion. That some ced-10(n1993) QL protrusions extended further than wild type suggests that CED-10/Rac might have a role in limiting extent of protrusion. The ced-10(n1993) allele is an incomplete loss-of-function mutation.Citation26,Citation28 Thus, CED-10/Rac might alone have additional roles in Q protrusion and migration not discovered in this analysis.
CED-10/Rac limits Q neuroblast migration whereas MIG-2/RhoG and CDC-42 are required for migration.
Next, migration of the Q neuroblasts was observed by examining the location of the division of the Q cells at 4–4.5 h after hatching with respect to the surrounding seam cells. In ced-10(n1993), a small but significant percentage of the QR and QL neuroblasts divided at a location farther from their normal division points atop V4 and V5, respectively: 15% of QR cells divided between the V3R and V4R seam cells; and 11% of QL divided between the V5L and V6L seam cells ( and ). This suggests that in ced-10(n1993) mutants, the Q cells migrated too far before division. This is consistent with the observation reported above that some QL protrusions at 1–1.5 h after hatching extended too far in ced-10(n1993) mutants, although in the case of division position, the effect was also observed in QR. These results suggest that CED-10/Rac might have a minor role in limiting Q neuroblast protrusion and migration.
In contrast to ced-10(n1993), a reduction in the distance of migration prior to division was observed for both the cdc-42(gk388M+) and the mig-2(mu28) single mutants, with the Q neuroblasts dividing between the V4 and V5 seam cells. In most cases, the cells migrated a short distance to either the posterior edge of V4R for QR or the anterior edge of V5L for QL ( and C). is an example of a cell migration defect of this sort in a mig-2;ced-10 double mutant. Rarely, the cells apparently did not migrate at all and divided directly between V4 and V5. is an example of a cell migration defect of this sort in a mig-2;ced-10 double mutant. Only QL was affected in mig-2(mu28), whereas both QL and QR were affected in cdc-42(gk388M+).
Taken together, these results suggest that each of these single mutants have only a small effect on Q cell polarizations or migrations individually. Both MIG-2/RhoG and CDC-42 appear to be required for the protrusion and migration of the Q neuroblasts. Conversely, CED-10/Rac appears to be involved in limiting Q neuroblast protrusion and migration, as the Q cells in the ced-10(n1993) mutant were observed to migrate past their normal stopping points before dividing.
CED-10/Rac, MIG-2/RhoG and CDC-42 have weak effects on Q cell descendant migrations, AQR and PQR.
The neuronal descendants of the Q neuroblasts AQR (from QR) and PQR (from QL) undergo long-range migrations to the anterior and posterior ( and H).Citation23,Citation24 To determine if these GTPases are involved in AQR and PQR long-range migration, the final positions of the AQR and PQR neurons once they had completed their migrations were observed in ced-10(n1993), mig-2(mu28) and cdc-42(gk388M+) single mutants.
Two distinct aspects of AQR and PQR migration were scored: the direction of migration and the extent of migration to their normal destinations. Directional migration defects seen for AQR and PQR have been shown to be dependent on the expression of MAB-5/Hox.Citation23,Citation30,Citation31 MAB-5 is a Hox transcription factor that is induced in QL and QL descendants and directs the further posterior migration of the QL descendants. In the absence of MAB-5/Hox, the QL descendants migrate anteriorly despite an initial posterior QL migration. The expression of MAB-5 is controlled by canonical Wnt signaling, with an EGL-20/Wnt signal being secreted from the posterior of the animal.Citation30–Citation37 Previous results suggest that the initial migrations of the Q neuroblasts affect their responses to the posterior EGL-20/Wnt signal.Citation21 By migrating posteriorly, the QL neuroblast encounters the EGL-20/Wnt signal and turns on the expression of MAB-5. By migrating anteriorly, the QR neuroblast might avoid the posterior EGL-20/Wnt signal. Failure of QL to migrate posteriorly over V5L could result in QL not receiving a robust EGL-20/Wnt signal and not expressing MAB-5/Hox, causing the QL descendants (e.g., PQR) to migrate anteriorly. Additionally, if QR fails to migrate anteriorly, it might be exposed to a stronger EGL-20/Wnt signal that activates MAB-5/Hox in QR and descendants, resulting in posterior migration of the QR descendants (e.g., AQR). QR appears to be inherently less sensitive to the EGL-20/Wnt signal,Citation37 possibly explaining the weaker defects in AQR direction defects compared to PQR (see below).
ced-10(n1993) and cdc-42(gk388M+) had very little effect on AQR and PQR migration ( and C). mig-2(mu28) had the strongest effect on AQR and PQR migration, with 4% of the AQR neurons failing to fully migrate to the wild-type location in the head of the animal and 7% of PQR neurons failing to migrate properly to the wild-type location in the tail (). One point of interest is that 1% of the PQR neurons in mig-2(mu28) had reversed direction of migration and migrated anteriorly to the area surrounding the vulva, a defect never observed in wild type. mig-2(mu28) displayed a low percentage of QL cells that failed to migrate posteriorly (). Possibly, these QL cells failed to receive the EGL-20/Wnt signal and thus PQR migrated anteriorly. No AQR or PQR directional defects were observed in ced-10(n1993) or cdc-42(gk388M+). These mig-2(mu28) defects are consistent with previous reports of Q descendant migration defects in mig-2 mutants.Citation27,Citation28
The Q cell migration defects and AQR/PQR Q descendant migration defects we observed in ced-10(n1993) and mig-2(mu28) alone were rather weak, consistent with the weak effects of mig-2 and ced-10 alone on cell migration described in previous studies. For example, mig-2(mu28) weakly perturbed migrations of the QR descendants AVM and SDQ and the activated mig-2(rh17) mutation more strongly affected Q descendant migration as well as migration of the CAN and HSN neurons.Citation27 mig-2 and ced-10 are required for proper migration of the gonadal distal tip cells in development of the somatic gonad,Citation12 and have roles in the ventral migrations of the P cell nuclei.Citation12,Citation14 mig-2 and ced-10 are also required for short-range migrations of the vulval cells in vulval development.Citation38 Additionally, activated mig-2(rh17) causes a reduction in speed of Q cell migration.Citation29 In these studies, mig-2 and ced-10 generally had weak effects alone but had very strong, synergistic effects in double mutants, prompting us to analyze mig-2(mu28);ced-10(n1993) double mutant Q migration.
CED-10/Rac and MIG-2/RhoG act synergistically to control protrusion and migration of the Q neuroblasts.
Previous studies have shown that CED-10/Rac and MIG-2/RhoG act in parallel pathways to control axon pathfinding,Citation12,Citation14,Citation28 P cell migration,Citation12,Citation14 CAN neuron migration,Citation12 and vulval cell migration.Citation38 Neither single mutation alone had strong axon pathfinding defects. Additionally, shown here and in Shakir et al. 2006,Citation28 CED-10/Rac and MIG-2/RhoG also act in parallel pathways in directing AQR and PQR migration. Since only weak defects in polarization and migration were observed for the Q neuroblasts in single mutants, mig-2(mu28);ced-10(n1993M+) double mutants were analyzed to determine if they act redundantly in Q migration. While the V cells were sometimes misshapen in ced-10;mig-2 mutants, their gross positions and morphologies were similar to wild type.
At 1–1.5 h after hatching when in wild-type animals the Q neuroblasts would be polarized and extending protrusions in opposite directions, most of the Q cells in the mig-2(mu28);ced-10(n1993M+) double mutants did not extend obvious protrusions in either anterior or posterior directions () (60% of QR and 80% of QL). Of the Q cells that did send out protrusions, the QL protrusions were always directed posteriorly and the QR protrusions were always directed anteriorly, as in wild type.
The divisions of the Q cells were observed at 4–4.5 h after hatching, after migration had occurred. A strong failure to migrate atop the neighboring seam cells before division was observed in the mig-2(mu28);ced-10(n1993M+) double mutants (; 56% of QR and 100% ofQL). Most of the mig-2(mu28);ced-10(n1993M+) Q cells did not migrate at all before dividing and divided between V4 and V5. No defects in direction of QL or QR migration were apparent. These results suggest that CED-10/Rac and MIG-2/RhoG are required redundantly in parallel pathways for Q cell protrusion and migration, but are not required for direction of polarization.
cdc-42 mutation weakly enhances mig-2 and ced-10 in Q neuroblast protrusion and migration.
CDC-42 has been shown to be involved in establishing cell polarity and to be involved in directional migration in cell culture.Citation10,Citation39 Since only weak defects in migration were observed for the Q cell descendants for the cdc-42 single mutants, double mutants were constructed of ced-10(n1993) and mig-2(mu28) with cdc-42(gk388M+) to determine if a robust role for CDC-42 could be observed in this sensitized background. As Cdc42 is involved in many aspects of cell polarization, protrusion and migration in other systems,Citation10,Citation39 it was a surprise to find that CDC-42 had only weak effects on Q cell protrusion and migration even in double mutant combinations, as described below. The V cells had the same gross position and morphology as wild type in cdc-42 double mutants.
In cdc-42(gk388M+);ced-10(n1993) animals, 2% of both QL and QR failed to extend obvious protrusions at 1–1.5 h after hatching (), a defect not observed in either single mutant alone (compare to and C). The proportion of all defects was not significantly increased, however. Protrusions were always in the correct direction. The Q cells were observed to divide much later than in wild type in the cdc-42(gk388M+);ced-10(n1993) animals, at 5.5–6 h after hatching. At the 5.5–6 h time point, cdc-42(gk388M+);ced-10(n1993) did not have significantly different defects in migration and division position compared to either single alone (). No defects in direction of migration were observed. As was observed with the ced-10(n1993) single mutant, a small percentage of the Q cells had migrated past the wild-type positions before dividing in the cdc-42(gk388M+);ced-10(n1993) double mutant, again suggesting that CED-10/Rac also inhibits over-migration of the Q neuroblasts and that CDC-42 does not interact with CED-10/Rac in this process.
In mig-2(mu28);cdc-42(gk388M+) at 1–1.5 h after hatching, Q neuroblast protrusion defects were not significantly different than the additive effects of either mutant alone (). At 4–4.5 h after hatching, examination of the divisions of the Q cells showed a slight increase in migration defects in mig-2(mu28);cdc-42(gk388M+) double mutants compared to mig-2(mu28) and cdc-42(gk388M+) alone, but these differences were not statistically significant (). No defects in direction of protrusion or migration were observed.
In sum, none of the effects of CDC-42 on Q protrusion and migration were as strong as those observed in the mig-2(mu28);ced-10(n1993M+) double mutant. While the effects were weak, these data indicate that CDC-42 might act in parallel to CED-10/Rac in Q cell protrusion and MIG-2/RhoG in Q neuroblast migration. However, a linear relationship between CDC-42 and CED-10 and between CDC-42 and MIG-2 could not be ruled out by the results presented here. Interestingly, no defects in direction of Q polarization were observed, which might have been expected based on the previous studies suggesting that CDC-42 is involved in polarization and directional migration.Citation10,Citation39
CED-10/Rac and MIG-2/RhoG act synergistically in AQR and PQR neuronal migration.
Previous studies indicated that CED-10/Rac and MIG-2/RhoG act redundantly in the migration of the Q descendants AQR and PQR.Citation28 No defects in AQR or PQR direction of migration were observed in these studies, which relied on an osm-6::gfp transgene that was expressed in AQR and PQR but also in other neurons in the head and tail, making it difficult to unambiguously determine the positions of AQR and PQR. To confirm these results and to refine the defects in AQR and PQR migration in mig-2(mu28);ced-10(n1993M+) double mutants, we used the gcy-32::gfp transgene. gcy-32::gfp is expressed in AQR, PQR and the two URX neurons and allows unambiguous identification of AQR and PQR.Citation21 Indeed, we found that mig-2;ced-10 double mutants did have AQR and PQR directional defects.
As shown above, mig-2(mu28);ced-10(n1993M+) double mutants exhibited a large number of Q neuroblasts that had failed to migrate before dividing (). Indeed, many (37%) of the PQR neurons migrated anteriorly rather than posteriorly in mig-2(mu28);ced-10(n1993M+), consistent with the initial failure of QL migration (). No AQR directional defects were observed, consistent with the idea that QR is inherently less sensitive to the EGL-20/Wnt signal than is QL.Citation37
Additionally, defects in the extent of AQR and PQR migration were observed in mig-2(mu28);ced-10(n1993M+) (). For example, the majority of PQR neurons remained in the posterior of the animal near their birthplace (). Many AQR migrations also stopped short of their wild-type position (). Together, these data indicate that CED-10/Rac and MIG-2/RhoG redundantly affect Q descendant neuronal migrations. The directional defects might be due to initial defects in Q cell migration and the defects in extent of migration might indicate a requirement of these CED-10/Rac and MIG-2/RhoG in neuronal migration.
CDC-42 acts with CED-10/Rac and MIG-2/RhoG in Q descendant migration.
cdc-42(gk388M+) strongly and significantly enhanced the AQR and PQR extent of migration defects of mig-2(mu28) (). For the cdc-42(gk388M+);ced-10(n1993) double mutants, a significant synergistic increase in defects was also observed, though not to the same extent as in the mig-2(mu28);cdc-42(gk388M+) double mutants (). This effect was most pronounced in PQR. The mig-2(mu28);cdc-42(gk388M+) and cdc-42(gk388M+);ced-10(n1993) double mutants did not display AQR/PQR directional migration defects, possibly reflecting the weak Q protrusion and migration defects in the double mutants.
When summarizing all of these data with CED-10/Rac, MIG-2/RhoG and CDC-42, it is clear that CED-10/Rac and MIG-2/RhoG are central players in Q protrusion and migration, whereas CDC-42 has only weak effects. All three of these GTPases also act redundantly to control migration of the neuronal descendants of the Q neuroblasts, with CED-10/Rac and MIG-2/RhoG again having the greatest effect and CDC-42 having a lesser effect. The weak effects of CDC-42 are surprising. A caveat with all experiments with CDC-42 is that the cdc-42(gk388) allele is lethal and must be balanced over a wild-type copy. Therefore, activity from the wild-type maternal copy of the gene might be sufficient to mediate Q cell protrusion and migration. We find this unlikely, as maternal cdc-42 activity would be required to perdure through embryogenesis and into larval development. RNAi of cdc-42 results in embryonic lethality,Citation40 proscribing the scoring of Q cell protrusion and migration in cdc-42(RNAi) animals.
The UNC-73/Trio GEF is required for robust Q cell protrusion.
UNC-73/Trio has two Dbl-homology guanine nucleotide exchange factor domains (DH-GEF domains).Citation13 DH1 acts as a GEF for both CED-10/Rac and MIG-2/RhoG, but not CDC-42.Citation13,Citation14,Citation41 The DH2 GEF domain of UNC-73 is specific for Rho. The Rac GEF activity of UNC-73 is required for axon pathfinding and interacts with CED-10/Rac and MIG-2/RhoG.Citation12,Citation28 An allele of unc-73 that is thought to perturb both Rac and Rho GEF activities of the protein has been shown to have defects in Q neuroblast protrusion and migration.Citation16 In contrast, the unc-73(rh40) allele used here is a missense mutation in the DH1 Rac GEF domain that biochemically attenuates the Rac GEF activity of the protein specifically.Citation13 Given that ced-10(n1993) and mig-2(mu28) affect Q protrusion and migration, we tested the effect of the unc-73(rh40) mutation on Q cell protrusion and migration. While the V cells were sometimes misshapen in unc-73(rh40), they had the same gross position and morphology compared to wild type.
At 1–1.5 h after hatching, unc-73(rh40) mutants displayed protrusion defects for both QL and QR, with a small percentage of both QL and QR neuroblasts failing to extend any protrusions (). No defects in direction of protrusion were observed in unc-73(rh40). Comparing these data to that of the mig-2(mu28);ced-10(n1993M+) double mutants (), the unc-73(rh40) mutants were weaker. Intriguingly, the protrusions observed for unc-73(rh40) mutants look very thin compared to wild type, resembling finger-like filopodial protrusions rather than the robust lamellipodia-like protrusions in wild type ( and B). These thin, finger-like protrusions were rarely if ever observed in mig-2, ced-10, cdc-42 or any double mutant combination of the three. These results suggest that Rac GEF function of UNC-73 is required to extend protrusions and is required for robust lamellipodial protrusion when they do form in unc-73(rh40) mutants.
Though the protrusions that the Q neuroblasts normally follow during migration were much less robust in the unc-73(rh40) mutants, the Q cells were still able to migrate correctly in most of the unc-73(rh40) animals observed. Examining the location of division at 4–4.5 h after hatching, most of the QL and QR neuroblasts had fully migrated before dividing over V5L and V4R, respectively, with the remaining neuroblasts migrating to the edges of the seam cells adjacent to their birthplaces before dividing ().
These results suggest that the UNC-73/Trio Rac GEF is playing a role in robust Q cell protrusion. Some Q cells failed to extend any protrusion in unc-73(rh40), similar to but weaker than mig-2;ced-10 double mutants. The thin, finger-like protrusions in unc-73(rh40) were not observed in mig-2;ced-10 doubles. This might represent a distinct, non-Rac-mediated role of UNC-73 in protrusion. More likely, given that the Rac GEF activity is specifically affected in unc-73(rh40), is that the thin protrusions are due to reduced but not eliminated CED-10/Rac and MIG-2/RhoG activity in unc-73(rh40) mutants. Possibly, UNC-73/Trio controls Rac GTPase activity in lamellipodium formation.
unc-73(rh40) also affected AQR and PQR migration (). The reversal defects of PQR were weak, and the extent of migration defects were stronger, but not as strong as the mig-2;ced-10 double mutants. These results suggest that UNC-73/Trio might not be the only Rac GEF that controls the activity of both CED-10 and MIG-2 in protrusion and migration of the Q neuroblasts.
PIX-1 is required for QL neuroblast protrusion and migration.
The C. elegans genome encodes 19 DH GEF domain containing proteins, including UNC-73/Trio. Mutations and/or RNAi of each of the 19 were analyzed for AQR and PQR migration defects. The pix-1(ok982) deletion removes four exons of PIX-1, including part of the DH domain, and is likely a null mutation (data not shown and Wormbase). pix-1(ok982) was found to have weak defects in PQR migration (6%; ). PIX-1 is similar to the PAK (p21-activated kinase) Interacting Exchange Factor, and has been shown previously to act in a Rac-independent pathway with GIT and PAK in C. elegans gonadal distal tip cell migration.Citation18 As described below, pix-1(ok982) alone caused weak defects in protrusion of the QL and QR neuroblasts and QL migration defects. The V cells were similar to wild type in position and morphology in pix-1 mutants.
At 1–1.5 h after hatching, pix-1(ok982) mutants displayed weak defects in protrusion for QL and QR (), with all neuroblasts polarized in the correct directions. The most common defect was weak protrusion that did not extend as far as wild type. pix-1(ok982) animals did not display the thin-finger-like protrusions as did unc-73(rh40).
At 4–4.5 h after hatching, migration defects were observed for only the QL neuroblast, and not the QR neuroblast (): 30% of QL failed to fully migrate posteriorly over V5L before dividing, and 1/44 did not migrate at all (i.e., divided at its birthplace).
As described above, pix-1(ok982) caused 6% of PQR neurons to not complete their migrations (). Migration of AQR was mostly wild-type (), but 1 out of 100 animals scored had a reversal in the direction of migration for AQR, with the final location of migration just anterior to the anus near the location of the PQR neuron (). In sum, these results indicate that PIX-1 contributes to Q neuroblast protrusion and migration.
PIX-1 and UNC-73/Trio act synergistically in controlling protrusion and migration of the Q neuroblasts.
To determine if UNC-73/Trio and PIX-1 have redundant roles in Q neuroblast migration, a double mutant of pix-1(ok982) and unc-73(rh40) was constructed. The Rac double mig-2(mu28);ced-10(n1993) was maternal-effect lethal, whereas the pix-1(ok982);unc-73(rh40) double mutant was viable and fertile.
Examination of the protrusions of the Q neuroblasts at 1–1.5 h after hatching indicated that PIX-1 and UNC-73 act in parallel pathways to control protrusion of these cells. The defects in protrusion of both QR and QL were much stronger than either single mutant alone (Compare with and ). At 1–1.5 h after hatching, many of the Q cells in unc-73(rh40);pix-1(ok982) had no visible protrusions. No defects in direction of protrusion were observed. In unc-73(rh40);pix-1(ok982), 37% of QL neuroblasts and 44% of QR neuroblasts had long protrusions similar to wild type. This is still much less severe than the mig-2(mu28);ced-10(n1993M+) double mutant, with a mere 4% of QL and 8% of QR neuroblasts extending robust protrusions. Many of the unc-73(rh40);pix-1(ok982) mutants displayed thin and finger-like protrusions in a proportion similar to that of unc-73(rh40) alone.
At 4–4.5 h after hatching, a synergistic increase in Q neuroblast migration defects was seen in the pix-1(ok982);unc-73(rh40) double mutants as compared to the single mutants alone (). As seen for the pix-1(ok982) single mutants, the QL neuroblast was affected much more strongly than QR, with QL migration defects observed more than twice as often as in the QR neuroblast (). That unc-73(rh40);pix-1(ok982) animals display a synergistic increase in Q protrusion and migration defects indicates that UNC-73/Trio and PIX-1 might act in parallel to control Q protrusion and migration.
PIX-1 acts in parallel to MIG-2/RhoG to control protrusion and migration of the Q neuroblasts.
The finding that PIX-1 and UNC-73 act in parallel to control Q protrusion and migration raises the question of whether PIX-1 is acting with or in parallel to CED-10 and MIG-2 in the Q neuroblasts, as UNC-73 has been shown to act with both CED-10 and MIG-2.Citation12,Citation13 To determine the genetic relationships between PIX-1 and the Rac GTPases CED-10 and MIG-2, double mutants were constructed and the Q neuroblasts were examined.
Double mutants of pix-1(ok982) with mig-2(mu28) display synergistic increases in defects in both protrusion and migration of the Q neuroblasts as compared to the defects observed for the single mutants combined ( and B). Examination of the Q neuroblast protrusions found that 43% of QL and 35% of QR neuroblasts either failed to extend protrusions or only extended small protrusions in the mig-2 pix-1 double mutant, a significant increase in defects as compared to a combined percentages of defects (15.5% for QL and 2.5% for QR) seen for the single mutants alone (compare to and ). As observed for the single mutants alone, the protrusions that were present in the mig-2 pix-1 double mutants were always in the proper directions. Defects in the location of divisions of the Q neuroblasts in the mig-2 pix-1 double mutant again showed a significant increase in defects as compared to the combination of defects seen in both of the single mutants, with 56% of QL and 20% of QR neuroblasts failing to fully migrate to their proper locations before dividing in the double mutant as compared to the combined defects observed for 35% of QL neuroblasts scored and no defects seen for QR migration in either single mutant (compare to and ). This significant increase in migration defects was also observed for the migrations of AQR and PQR, with the mig-2 pix-1 double mutant again showing an increase in defects as compared to the combination of defects observed for the single mutants (compare to and ). These results suggest that PIX-1 and MIG-2 are working in parallel pathways to control the migrations of the Q neuroblasts and their descendants.
PIX-1 might act with CED-10/Rac to control protrusion and migration of the Q neuroblasts.
The pix-1;ced-10 double mutants were developmentally delayed as compared to wild-type animals, based on the observation that the divisions of the Q neuroblasts occurred approximately 30 minutes later than wild type at 4.5–5 h after hatching. Thus, Q neuroblast protrusions were also examined later, at 2–2.5 h after hatching. Q protrusion and migration defects in ced-10; pix-1 were not significantly stronger than either single mutant alone, though 7% of QL neuroblasts remained unpolarized, a phenotype not observed for either pix-1 or ced-10 single mutants. Defects in QL migration and division were not significantly different, but 7% QR showed migration defects () compared to none in ced-10 and pix-1 alone.
The migration defects for the AQR neuron were not significantly different than the combination of the defects observed for both of the single mutants (p = 0.3). As for the PQR neuron, the migration defects in the pix-1;ced-10 double mutant were slightly increased compared to the combination of defects observed for the pix-1 and ced-10 single mutants (p = 0.03). Some defects were weakly enhanced in the ced-10;pix-1 double mutant (; QR migration and PQR migration), but the general trend was no significant enhancement, especially when compared to pix-1 mig-2. Based on this lack of a synergistic increase in defects seen for the Q neuroblast and descendant migrations, these results suggest that PIX-1 and CED-10 might be acting in the same pathway to control the migrations of the Q descendants. These results together with previous findings that UNC-73 serves as a GEF for CED-10 and MIG-2,Citation12–Citation14 suggest that CED-10 might act with both UNC-73 and PIX-1, whereas MIG-2 might act with UNC-73 in parallel to the PIX-1/CED-10 pathway to control the migrations of the Q neuroblast descendants.
One point of interest in these data was that of the Q neuroblasts observed during the division stage in the pix-1;unc-73 double mutants, 2/28 of the QL neuroblasts had divided on the posterior edge of V4L, a phenotype that was not observed for any of the single mutants examined here. Though this could indicate an anterior migration of the QL neuroblasts before division in this double mutant, another possible explanation could be the behavior of the V4 seam cell after division of ABp l/r apapaa to produce V5 and the Q cells. We found that the V4 seam cells extend posteriorly to make contact with the V5 seam cells beneath the Q cells after the initiation of migration of the Q neuroblasts. This could cause neuroblasts that had failed to migrate to appear to have migrated anteriorly, even if these cells had not migrated at all. This could also partially explain the differences between the QL and QR migration defects seen in many of the mutants observed here, as the defects in protrusion extension are mostly similar between the QL and QR neuroblasts for all mutants, whereas the defects in migration were typically stronger for the QL neuroblasts in the same mutants.
Discussion
The RAC GTPases CED-10/Rac and MIG-2/RhoG have opposite effects on the protrusion extension and migration of the Q neuroblasts.
Loss of function mutations in the Rac GTPases ced-10 and mig-2 do not cause defects in the direction of polarization of the Q neuroblasts. Of the Q neuroblasts that do extend protrusions, these processes are always oriented in the proper direction of polarization, with QR extending protrusions anteriorly and QL extending protrusions posteriorly. These mutants do however affect the size of the protrusions, with ced-10(n1993) mutants observed to extend protrusions that are increased in length as compared with wild-type Q cell protrusions. The positions at which Q cells divided were similarly affected by ced-10 mutation (i.e., they divided between the V3R and V4R seam cells for QR and between the V5L and V6L seam cells for QL). Opposite defects were observed for mig-2(mu28), with a small percentage of the Q neuroblasts failing to extend any protrusions at 1–1.5 h after hatching. Some Q cells also failed to migrate completely before dividing in mig-2(mu28). Taken together, these data suggest that CED-10/Rac normally plays a minor inhibitory role in the protrusions and migrations of the Q neuroblasts and that MIG-2/RhoG is required for full extension of protrusions and for complete migrations of the Q neuroblasts. These results suggest that the Q cells use the initial protrusion as a pathway for subsequent migration. CED-10/Rac and MIG-2/RhoG might affect the size of the initial protrusions or the speed of outgrowth of the initial protrusions and/or migrations. The latter is consistent with results from Ou and Vale, 2009,Citation29 who found that activated MIG-2/RhoG slowed Q cell migration speed. Thus, the consequence of activated MIG-2/RhoG was similar to loss of MIG-2/RhoG, highlighting the caveats of using activated and dominant negative mutations to study Rho GTPase function.
The Q cell migration defects and AQR/PQR Q descendant migration defects we observed in ced-10(n1993) and mig-2(mu28) alone were rather weak, consistent with the weak effects of mig-2 and ced-10 alone on cell migration described in previous studies. For example, mig-2(mu28) weakly perturbed migrations of the QR descendants AVM and SDQR, and the activated mig-2(rh17) mutation more strongly affected Q descendant migration as well as migration of the CAN and HSN neurons.Citation27 mig-2 and ced-10 are required for proper migration of the gonadal distal tip cells in development of the somatic gonad,Citation12 and have roles in the ventral migrations of the P cell nuclei.Citation12,Citation14 mig-2 and ced-10 are also required for short-range migrations of the vulval cells in vulval development.Citation38 Additionally, activated mig-2(rh17) causes a reduction in speed of Q cell migration.Citation29 In these studies, mig-2 and ced-10 generally had weak effects alone but had very strong, synergistic effects in double mutants, similar to what we observed here for Q and Q descendant migrations.
CED-10/Rac and MIG-2/RhoG have redundant roles in directing the Q neuroblast protrusion and migration.
Though CED-10/Rac and MIG-2/RhoG appear to have opposite roles in controlling protrusion extension and migration in the Q neuroblasts, they also have redundant roles in extension of protrusions and migration. ced-10;mig-2 double mutants show a synergistic increase in protrusion and migration defects, with many Q neuroblasts not extending any protrusions and failing to migrate away from their birthplace between V4 and V5. Functional redundancy of CED-10/Rac and MIG-2/RhoG has previously been observed in axon pathfinding.Citation12,Citation28 Q neuroblast protrusion, Q neuroblast migration and axon pathfinding all might be controlled by the same signaling pathway in which CED-10/Rac and MIG-2/RhoG function redundantly. This pathway might control lamellipodial protrusion in each case. CED-10/Rac and MIG-2/RhoG might also act in independent signaling pathways with different molecules, with CED-10/Rac limiting Q neuroblast protrusion and MIG-2/RhoG stimulating neuroblast protrusion. These results indicate that CED-10/Rac is required for both inhibition and stimulation of protrusion and might explain why dominant negative and constitutively active versions of Racs can cause similar phenotypes, possibly by acting via different GEFs.
CDC-42 has little effect on q neuroblast protrusion but acts redundantly with cEd-10/rac and miG-2/rhoG in q descendant migration.
Loss of function cdc-42 mutants displayed weak defects in the protrusions and migrations of the Q neuroblasts, similar to the defects observed for mig-2 mutants, suggesting that CDC-42 is required for protrusion and migration in the Q cells. Double mutants of cdc-42 with ced-10 and mig-2 did not display a strong increase in defects in protrusion and migration of the Q cells as compared to the defects of the single mutants combined, suggesting that CDC-42 might be working in the same pathway or in independent pathways to control Q neuroblast protrusion and migration. In any case, the role of CDC-42 in Q protrusion and migration is surprisingly weak, given the central role that Cdc42 plays in other protrusive events. In contrast, mig-2;cdc-42 and cdc-42;ced-10 double mutants displayed a synergistic increase in migration defects for the Q descendants AQR and PQR, suggesting that MIG-2/RhoG, CED-10/Rac and CDC-42 might act redundantly in Q descendant migration.
One caveat of these experiments is that the null cdc-42 mutant used here is maternal effect lethal and homozygotes have wildtype maternal contribution. Indeed, RNAi of cdc-42 results in embryonic lethality.Citation40 Therefore, maternal contribution of cdc-42 RNA could be rescuing any defects that might be present in the Q neuroblasts. If the maternal contribution of cdc-42 RNA is rescuing Q migration defects, it might be expected that an increase in migration defects would be observed for the later Q descendant migration events as compared to the early Q neuroblast migrations as the cdc-42 RNA is depleted. This was not the case as cdc-42 mutants did not display strong differences in the amount of defects in the early Q neuroblast migrations versus those observed for the later Q descendant migrations. However, when mutation in cdc-42 was introduced into the ced-10 and mig-2 mutant backgrounds, the proportion of defects of the AQR and PQR migrations was increased (i.e., cdc-42 enhanced ced-10 and mig-2 in Q descendant migrations but not in earlier Q neuroblast protrusion and migration). However, we cannot rule out a role of CDC-42 in Q neuroblast protrusion and migration that was not revealed by these studies.
While direction of Q protrusion and migration was not affected in mig-2;ced-10, the direction of PQR migration was often reversed. Furthermore, misdirected PQR neurons sometimes migrated for a greater distance anteriorly that they normally would posteriorly. We interpret this as an effect of failing to activate mab-5 expression in QL due to initial QL migration defects, similar to what was observed for mig-15 mutants.Citation21 QL′s failure to migrate posteriorly results in a failure of the posterior EGL-20/Wnt signal to activate MAB-5 expression in QL. Thus, the later migratory differences of PQR can be attributed to different response to guidance cues mediated by MAB-5.
UNC-73/Trio controls robust lamellipodial protrusion.
Previous studies have determined UNC-73 to act as a guanine nucleotide exchange factor (GEF) for the CED-10 and MIG-2 Rac GTPases.Citation12,Citation13 Thus, the unc-73(rh40) allele, a mutation thought to attenuate the GEF activity for Rac GTPases, was examined for Q neuroblast protrusion and migration defects.Citation13 Protrusions in unc-73(rh40) were much reduced in size as compared to wild type: they were often as long as wild type but were highly branched and thinner, more filopodial-like protrusions. This result suggests that UNC-73 is required for robust lamellipodial extensions. The filopodial-like, thin Q neuroblast protrusions were not observed in the mig-2;ced-10 double mutants, though the lack of these structures could be due to the Rac GTPase double mutant causing much more severe defects in protrusion extension, with the majority of the Q neuroblasts not sending out any protrusions at 1–1.5 h after hatching. In other words, UNC-73/Trio might control one aspect of Rac function in Q protrusion, the formation of robust lamellipodial protrusions. Possibly, UNC-73 controls a distinct population of Rac molecules that mediate lamellipodia formation. In addition to the unc-73 protrusion defects being less severe than those seen for the mig-2;ced-10 double mutants, a similar relationship was observed for the Q neuroblast and Q neuroblast descendant migration defects, in which the unc-73 mutants displayed migration defects that were much weaker than observed for the mig-2;ced-10 double mutant. These results suggest Rac has multiple, discrete roles in Q protrusion and migration and other GEFs might also control Rac function in Q protrusion and migration.
PIX-1 acts redundantly with UNC-73/Trio in Q neuroblast protrusion and migration.
Examination of all 19 C. elegans DH-GEF genes revealed that PIX-1 has a role in the protrusion extension and migration of the Q neuroblasts and in the migration of the Q descendants. pix-1 mutants alone had weak but significant effects on protrusion and migration of the Q neuroblasts as well as in the migration of the AQR and PQR neurons. The thin, filopodial-like protrusions observed in unc-73(rh40) were not observed in pix-1, indicating that UNC-73/Trio and PIX-1 might have distinct roles in Rac regulation in Q neuroblast protrusion and migration. However, double mutants of unc-73 with pix-1 displayed a synergistic increase in protrusion and migration defects in the Q neuroblasts, with many Q cells failing to extend any noticeable protrusions and failing to migrate completely. These results suggest that PIX-1 and UNC-73 act in parallel pathways to control the protrusion and migration of the Q neuroblasts. Thus, UNC-73/Trio and PIX-1 have individual, unique roles as well as overlapping, redundant roles in Q neuroblast polarization and migration, similar to the Rac GTPases themselves. The defects in unc-73;pix-1 double mutants were not as severe as those in mig-2;ced-10 double mutants. This might suggest that other regulators of MIG-2/RhoG and CED-10/Rac are also involved in Q migration, possibly other GEFs that act redundantly with UNC-73/Trio and PIX-1.
PIX-1 might act in the CED-10/Rac pathway in Q neuroblast protrusion and migration.
UNC-73 has been shown to act with both MIG-2/RhoG and CED-10/Rac.Citation12–Citation14 Here we show that UNC-73/Trio and PIX-1 act in parallel to control Q neuroblast protrusion and migration. PIX-1 could be acting in parallel to the UNC-73/CED-10/MIG-2 pathway, as PIX-1 has been shown to act in parallel to Rac signaling in distal tip cell migration.Citation18 Alternatively, PIX-1 could be acting in one or both of the CED-10/Rac and MIG-2/RhoG pathways.
We found that mig-2 pix-1 but not pix-1;ced-10 double mutants displayed a strong, synergistic increase in Q neuroblast and Q descendant migration defects (). The proportions of defective Q protrusions and migrations as well as AQR and PQR migrations were significantly different in the pix-1 mig-2 double mutant. These results suggest that PIX-1 might act specifically in the CED-10/Rac pathway in parallel to MIG-2/RhoG to control Q neuroblast protrusion and migration. Interestingly, previous studies have found that PIX-1 functions in a pathway that is parallel to the Rac GTPases CED-10/Rac and MIG-2/RhoG in gonad morphogenesis.Citation18 The results shown here suggest that PIX-1 might also function in a linear pathway with the Rac GTPase CED-10, indicating that the relationship of PIX-1 with the Rac GTPases is tissue specific.
PIX molecules have Rac-specific GEF activity,Citation17 but the activity of PIX-1 in C. elegans has not been determined. PIX-1 also has Rac-independent functions.Citation18 Thus, the role of PIX-1 in Q protrusion and migration might involve control of Rac activity via the GEF domain or might be in a parallel, Rac-independent pathway. That PIX-1 appears to act genetically in the CED-10/Rac pathway suggests that it might indeed control CED-10 activity in this process.
A summary of our results is presented in . UNC-73/Trio and PIX-1 act redundantly in Q neuroblast protrusion and migration. UNC-73/Trio might act in both the CED-10/Rac and MIG-2/RhoG pathways, whereas PIX-1 might act in the CED-10/Rac pathway in parallel to MIG-2/RhoG. UNC-73/Trio also is required for robust lamellipodial protrusion, a function that is distinct from PIX-1. MIG-2/RhoG alone is required for Q neuroblast protrusion and migration, whereas CED-10/Rac alone is required to limit Q neuroblast protrusion and migration. CED-10/Rac and MIG-2/RhoG act redundantly in Q neuroblast protrusion and migration. Thus, Rac GTPases and their GEF regulators have both individual and redundant roles in Q neuroblast protrusion and migration. A future challenge will be to determine the discrete signaling complexes of Rac GTPases and GEFs that control these distinct aspects of neuroblast migration.
Materials and Methods
Genetic methods.
All experiments were performed at 20°C using standard techniques.Citation19,Citation20 The following mutations and transgenic constructs were used: X: mig-2(mu28), pix-1(ok982); I: lqIs40[Pgcy-32::gfp], unc-73(rh40); II: cdc-42(gk388)/mIn1[mIs14 dpy-10(e128)]; IV: ced-10(n1993), lqIs80[scm promoter::gfp::caax].Citation21 Extrachromosomal arrays were generated by germ line microinjection and integrated into the genome by standard techniques.Citation22 All mutations were outcrossed to wild type N2 animals at least three times.
Double mutants were constructed by standard genetic methods. In some cases balancer chromosomes were used to maintain lethal or sterile combinations (e.g., cdc-42(gk388) and ced-10;mig-2). Double mutants were first identified phenotypically (Ced, Mig, Unc or lethal/sterile) and were confirmed by geno-typing PCR on deletion alleles cdc-42(gk388) and pix-1(ok982) and DNA sequencing on point mutations (ced-10(n1993), mig-2(mu28), unc-73(rh40)). Homozygous mutant progeny from balanced stocks will have a wild-type maternal contribution, indicated by an "M+" in the genotype.
Synchronization of L1 larvae to visualize Q cell polarization and migration.
Methods used for synchronization of L1 larvae were described previously.Citation16,Citation21 Synchronized L1 larvae were obtained by washing NGM plates containing eggs with M9 buffer at 30-minute intervals. The collected larvae were placed on NGM plates with a bacterial lawn to allow for continued development until the specified time of imaging. Eggs were isolated from egg-laying-defective worms by bleach treatment of gravid adults.Citation20
Scoring of Q neuroblast protrusion and migration.
The protrusions and migrations of the Q neuroblasts were visualized in L1 larvae using the transgene lqIs80[scm promoter::gfp::caax].Citation21 Animals were analyzed using a Leica DMR compound microscope with a BD CARV II spinning disk confocal or wide-field fluorescence. Images were captured using IPlab software and a QImaging Rolera mGi camera.
Q neuroblast protrusion and migration were quantified as described previously.Citation21 Unless otherwise noted, Q cell protrusion was scored at 1–1.5 h after hatching; and Q neuroblast migration and division was scored at 4–4.5 h after hatching. For protrusion at 1–1.5 h: strong anterior/posterior protrusion was scored as the Q protrusion extending completely over the V4/5 seam cells similar to wild type; weak anterior/posterior protrusion was scored as protrusions extending less than half the distance over the seam cell; and unpolarized was scored as Q cells that emanated no robust protrusion in any direction. For migration/division at 4–4.5 h, the position at which the Q neuroblasts first divided relative to the seam cells is indicated on the X axis of the graph. Statistical significances were determined by Fisher Exact Analysis.
Scoring and analysis of AQR and PQR migration defects.
The location of the AQR and PQR neurons were visualized in L4 larvae to young adults using the transgene lqIs40[Pgcy-32::gfp].Citation21 AQR and PQR were distinguished by the side of the animal they occupied using the microscope focus and the dorsal-ventral orientation of the animal (AQR was on the right and PQR was on the left). The locations of the neurons after completion of migration were classified into 5 different anterior-posterior positions of the worm as described previously,Citation21 with position 1 = head of the animal to just posterior to the second pharyngeal bulb, 2 = just posterior of the second pharyngeal bulb to slightly anterior of the vulva, 3 = the anterior and posterior regions surrounding the vulva, 4 = slightly posterior to the vulva to just anterior to the anus, 5 = posterior to the anus to the tail of the animal. Significances of difference were determined by Fisher Exact Analysis.
Figures and Tables
Figure 1 Protrusions and migrations of the Q neuroblasts and migrations of the AQR and PQR neurons in wild-type animals. (A–D) Confocal fluorescent micrographs of Q neuroblasts in wild-type L1 larvae visualized with scm::gfp::caax expression. Asterisks mark the position of the Q neuroblasts and the Q neuroblast descendants. Tracings of the Q neuroblasts and the Q neuroblast descendants are located beneath each micrograph. The scale bar in (A) represents 5 µm for (A–D). (A) A QR neuroblast polarizes, sending out protrusions anteriorly over the V4R seam cell at 1–1.5 h after hatching. (B) A QL neuroblast polarizes, sending out protrusions posteriorly over the V5L seam cell at 1–1.5 h after hatching. (C) A QR neuroblast divides over the V4R seam cell after migrating anteriorly at 4–4.5 h after hatching. (D) AQL neuroblast divides over the V5L seam cell after migrating posteriorly at 4–4.5 h after hatching. (E) Quantitation of the direction and extent of protrusions during the polarization stage of the Q neuroblasts at 1–1.5 h after hatching in wild-type animals. The x-axis represents the direction and extent of protrusion of the Q neuroblasts. The y-axis represents the percentage of Q neuroblasts protrusions in each of the categories along the x-axis. For both QR and QL protrusions, n = 55. (F) Quantitation of the location of the Q neuroblasts at division with respect to the adjacent seam cells in wild-type animals. The x-axis represents the location of the Q neuroblasts at division with respect to the adjacent seam cells. The y-axis represents the percentage of Q neuroblasts located at those positions. For both QR and QL migrations, n ≥ 29. (G) Epifluorescent micrograph of the AQR and PQR neurons in a wild-type L4 larvae visualized with Pgcy-32::gfp. The scale bar in (G) represents 10 µm. (H) Quantitation of the final migratory positions of the AQR and PQR neurons in wild-type animals. The x-axis represents the final position of the AQR and PQR neurons along the anterior-posterior axis of the animal as described in the Materials and Methods section. The y-axis represents the percentage of AQR and PQR neurons located at those positions along the anterior-posterior axis. See Materials and Methods for scoring criteria. For both cases, n = 100.
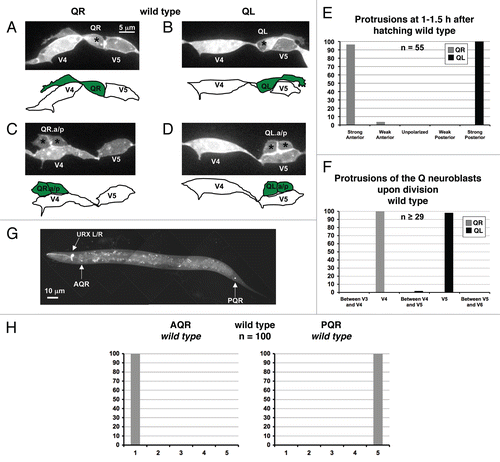
Figure 2 Quantitation of protrusion defects of the Q neuroblasts in mutants. (A–F) Quantitation of the direction and extent of protrusions during the polarization stage of the Q neuroblasts at 1–1.5 h after hatching. The graphs are organized as described in and Materials and Methods. The asterisks in (D) represent statistically significant differences (p < 0.001) as compared to the ced-10(n1993) and mig-2(mu28) single mutants alone. For all cases, n ≥ 25.
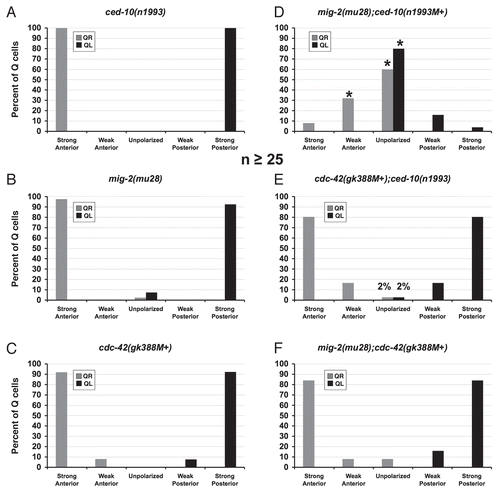
Figure 3 Q neuroblast protrusion defects in mutants. (A–C) Confocal fluorescent micrographs of Q neuroblasts in L1 larvae visualized with scm::gfp::caax expression at 1–1.5 h after hatching. Asterisks mark the position of the Q neuroblasts. Tracings of the Q neuroblasts are located beside each micrograph. The scale bar in (A) represents 5µm for (A–C). (A) A QL neuroblast in a ced-10(n1993) mutant extends protrusions posteriorly that are longer in size than in wild type, with the protrusion extending well onto the V6L seam cell. (B) A QL neuroblast in a cdc-42(gk388M+) mutant fails to send out robust protrusions posteriorly, though small protrusions extending in the proper posterior direction can be observed. (C) A QL neuroblast in a mig-2(mu28);ced-10(n1993M+) mutant fails to extend protrusions in either direction.
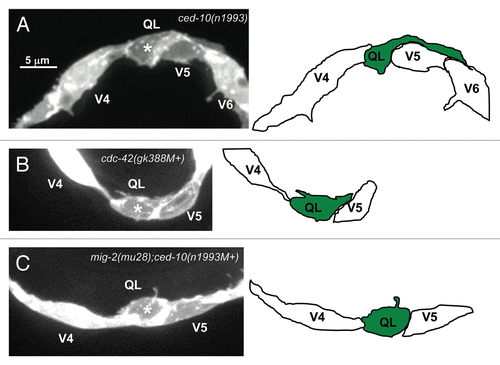
Figure 4 Quantitation of migration and division defects of the Q neuroblasts in mutants. (A–F) Quantitation of the location of the Q neuroblasts at division (4–4.5 h after hatching for A–D and F; 5–5.6 h after hatching for E). The graphs are organized as described in and Materials and Methods. The p values for the Q neuroblasts that were located between the V4 and V5 seam cells upon division are indicated. For all cases, n ≥ 25.
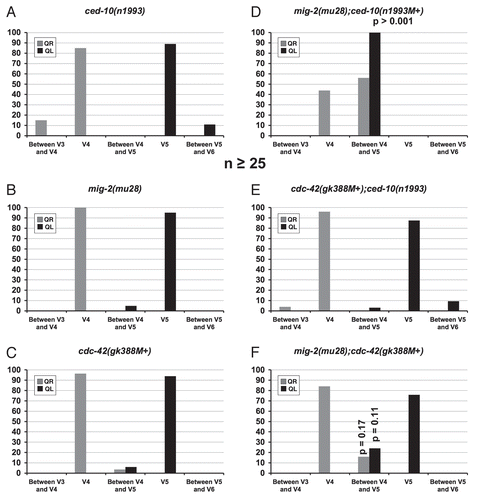
Figure 5 Failure of the Q neuroblasts to migrate before dividing in mutants. (A–C) Confocal fluorescent micrographs of Q neuroblasts upon division in L1 larvae 4–4.5 h after hatching visualized with scm::gfp::caax expression. Asterisks mark the positions of the Q neuroblast descendants. Tracings of the Q neuroblast descendants are located beneath each micrograph. The scale bar in (A) represents 5 µm for (A–C). (A) A QR neuroblast migrates past the normal stopping point over the V4R seam cell and divides between the V3R and V4R seam cells in ced-10(n1993) mutants. (B) A QL neuroblast fails to fully migrate before dividing, with the division occurring on the anterior edge of the V5L seam cell in mig-2(mu28);ced-10(n1993M+) mutants. (C) A QR neuroblast fails to migrate at all and divides at its birthplace between the V4R and V5R seam cells in mig-2(mu28);ced-10(n1993M+) mutants.
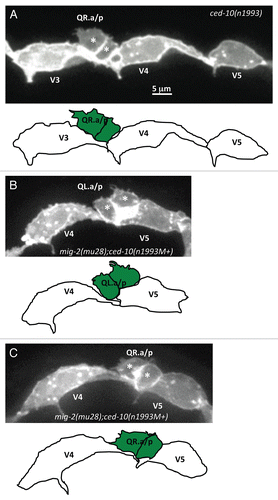
Figure 6 AQR and PQR, Q neuroblast descendants, migration defects in mutants. (A–F) Quantitation of the final migratory positions of the AQR and PQR neurons. The graphs are organized as described in and Materials and Methods. The asterisks in (D–F) represent statistically significant differences for the double mutants as compared to the combination of the defects observed in the two single mutants. For all cases, n = 100.
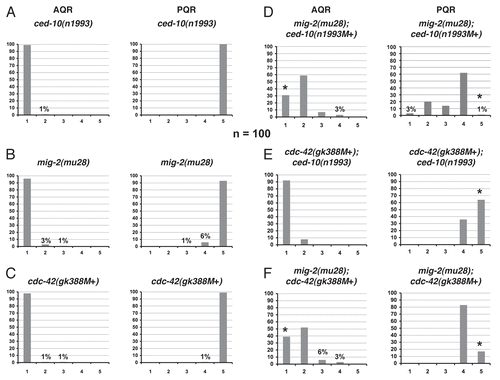
Figure 7 UNC-73/Trio is required for robust lamellipodial protrusion. (A and B) Confocal fluorescent micrographs of Q neuroblasts in unc-73(rh40) mutants in L1 larvae visualized with scm::gfp::caax expression at 1–1.5 h after hatching. Asterisks mark the position of the Q neuroblasts. Tracings of the Q neuroblasts are located beneath each micrograph. The scale bar in (A) represents 5µm for (A and B). QL (A) and QR (B) neuroblasts polarized in the correct directions, but the sizes of the protrusions were reduced and resembling filopodial rather than robust lamellipodial protrusion seen in wild type. (C) Quantitation of the direction and extent of protrusions during the polarization stage of the Q neuroblasts at 1–1.5 h after hatching in unc-73(rh40) mutants. The graphs are organized as described in and Materials and Methods. For Q neuroblast polarizations, n = 38. (D) Quantitation of the location of the Q neuroblasts at division with respect to the adjacent seam cells in unc-73(rh40) mutants. The graphs are organized as described in and Materials and Methods. For Q neuroblast migrations, n ≥ 31. (E) Quantitation of the final migratory positions of the AQR and PQR neurons in unc-73(rh40) mutants. The graphs are organized as described in and Materials and Methods. For AQR and PQR migrations, n = 100.
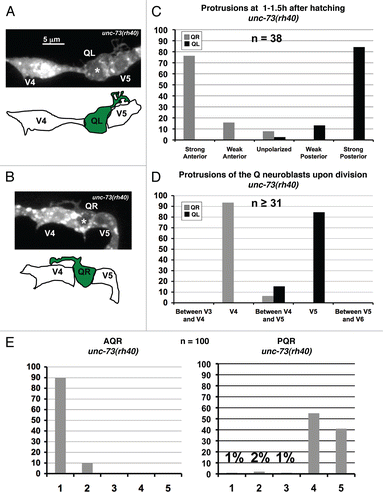
Figure 8 PIX-1 is required for the proper migrations of the QL neuroblast and the Q neuroblast descendants. (A) Quantitation of the direction and extent of protrusions during the polarization stage of the Q neuroblasts at 1–1.5 h after hatching in pix-1(ok982) mutants. The graphs are organized as described in and Materials and Methods. For Q neuroblast polarizations, n = 25. (B) Quantitation of the location of the Q neuroblasts at division with respect to the adjacent seam cells in pix-1(ok982) mutants. The graphs are organized as described in and Materials and Methods. For Q neuroblast migrations, n ≥ 25. (C) Quantitation of the final migratory positions of the AQR and PQR neurons in pix-1(ok982) mutants. The graphs are organized as described in and Materials and Methods. For AQR and PQR migrations, n = 100.
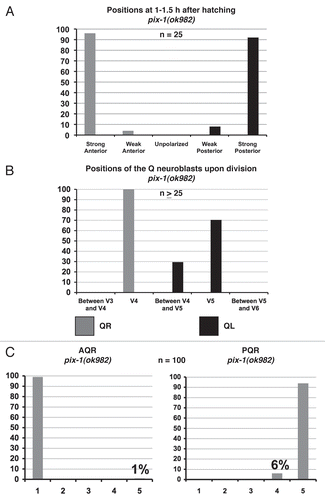
Figure 9 pix-1;unc-73 double mutants display a synergistic increase in defects in protrusion extension and migration of the Q neuroblasts and the migrations of the Q neuroblast descendants. (A) Quantitation of the direction and extent of protrusions during the polarization stage of the Q neuroblasts at 1–1.5 h after hatching in pix-1(ok982);unc-73(rh40) mutants. The graphs are organized as described in and Materials and Methods. For Q neuroblast polarizations, n ≥ 34. (B) Quantitation of the location of the Q neuroblasts at division with respect to the adjacent seam cells in pix-1(ok982);unc-73(rh40) mutants. The graphs are organized as described in and Materials and Methods. For Q neuroblast migrations, n ≥ 28. (C) Quantitation of the final migratory positions of the AQR and PQR neurons in pix-1(ok982);unc-73(rh40) mutants. The graphs are organized as described in and Materials and Methods. For AQR and PQR migrations, n = 100. Asterisks indicate that differences between the pix-1;unc-73 double mutant and the single mutants combined were significant (p < 0.001).
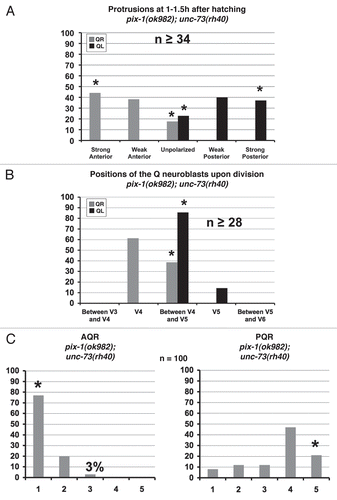
Figure 10 pix-1 mig-2 but not pix-1;ced-10 double mutants exhibit a synergistic increase in defects in Q neuroblast protrusion and migration and Q neuroblast descendant migration. (A and D) Quantitation of the direction and extent of protrusions during the polarization stage of the Q neuroblasts at 1–1.5 h after hatching for (A) and 2–2.5 h after hatching for (D). The graphs are organized as described in and Materials and Methods. For Q neuroblast polarizations, n ≥ 25. (B and E) Quantitation of the location of the Q neuroblasts at division with respect to the adjacent seam cells. The graphs are organized as described in and Materials and Methods. For Q neuroblast migrations, n ≥ 26. (C and F) Quantitation of the final migratory positions of the AQR and PQR neurons. The graphs are organized as described in and Materials and Methods. For AQR and PQR migrations, n = 100. Asterisks indicate that differences between the pix-1 mig-2 double mutant and the single mutants combined were significant (p < 0.001).
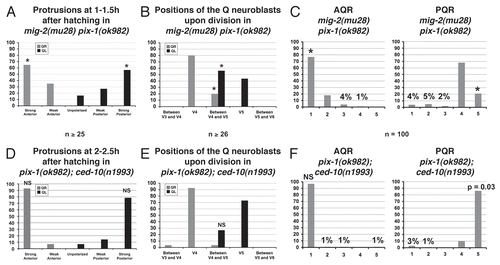
Figure 11 Rac GTPases and their regulators UNC-73/Trio and PIX-1 have unique and redundant roles in Q neuroblast protrusion and migration. (A) Unique roles of the Rac GTPases, CDC-42 and GEF regulators. MIG-2/RhoG, CDC-42 and PIX-1 all are required for Q neuroblast protrusion and migration and UNC-73/Trio is required for robust lamellipodial protrusion. CED-10 is required to limit Q neuroblast protrusion and migration. (B) Redundant roles of the Rac GTPases and GEF regulators. PIX-1 and UNC-73/Trio have redundant roles in Q neuroblast protrusion and migration. PIX-1 might act specifically in the CED-10/Rac pathway in parallel to MIG-2/RhoG.
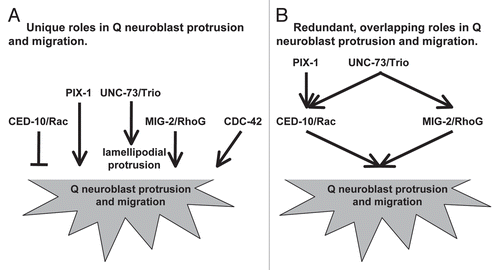
Acknowledgements
The authors wish to thank members of the Lundquist and Ackley labs for helpful discussion, E. Struckhoff for technical assistance and the C. elegans Gene Knockout Consortium for pix-1(ok982). Some nematode strains used in this work were provided by the Caenorhabditis Genetics Center, which is funded by the NIH National Center for Research Resources (NCRR). This work was supported by NIH R01 NS040945 and NSF IOB 93192 to E.A.L. and NIH P20 RR16475 (PI: J.S. Hunt).
References
- Insall RH, Machesky LM. Actin dynamics at the leading edge: from simple machinery to complex networks. Dev Cell 2009; 17:310 - 322
- Hall A. Rho GTPases and the actin cytoskeleton. Science 1998; 279:509 - 514
- Raftopoulou M, Hall A. Cell migration: Rho GTPases lead the way. Dev Biol 2004; 265:23 - 32
- Ridley AJ, Schwartz MA, Burridge K, Firtel RA, Ginsberg MH, Borisy G, et al. Cell migration: integrating signals from front to back. Science 2003; 302:1704 - 1709
- Kolluri R, Tolias KF, Carpenter CL, Rosen FS, Kirchhausen T. Direct interaction of the Wiskott-Aldrich syndrome protein with the GTPase Cdc42. Proceedings of the National Academy of Sciences of the United States of America 1996; 93:5615 - 5618
- Higgs HN, Pollard TD. Activation by Cdc42 and PIP(2) of Wiskott-Aldrich syndrome protein (WASp) stimulates actin nucleation by Arp2/3 complex. J Cell Biol 2000; 150:1311 - 1320
- Welch MD. The world according to Arp: regulation of actin nucleation by the Arp2/3 complex. Trends Cell Biol 1999; 9:423 - 427
- Aspenstrom P, Lindberg U, Hall A. Two GTPases, Cdc42 and Rac, bind directly to a protein implicated in the immunodeficiency disorder Wiskott-Aldrich syndrome. Curr Biol 1996; 6:70 - 75
- Machesky LM, Insall RH. Scar1 and the related Wiskott-Aldrich syndrome protein, WASP, regulate the actin cytoskeleton through the Arp2/3 complex. Curr Biol 1998; 8:1347 - 1356
- Heasman SJ, Ridley AJ. Mammalian Rho GTPases: new insights into their functions from in vivo studies. Nat Rev Mol Cell Biol 2008; 9:690 - 701
- Nobes CD, Hall A. Rho, rac and cdc42 GTPases regulate the assembly of multimolecular focal complexes associated with actin stress fibers, lamellipodia and filopodia. Cell 1995; 81:53 - 62
- Lundquist EA, Reddien PW, Hartwieg E, Horvitz HR, Bargmann CI. Three C. elegans Rac proteins and several alternative Rac regulators control axon guidance, cell migration and apoptotic cell phagocytosis. Development 2001; 128:4475 - 4488
- Steven R, Kubiseski TJ, Zheng H, Kulkarni S, Mancillas J, Ruiz Morales A, et al. UNC-73 activates the Rac GTPase and is required for cell and growth cone migrations in C. elegans. Cell 1998; 92:785 - 795
- Wu YC, Cheng TW, Lee MC, Weng NY. Distinct rac activation pathways control Caenorhabditis elegans cell migration and axon outgrowth. Dev Biol 2002; 250:145 - 155
- Kubiseski TJ, Culotti J, Pawson T. Functional analysis of the Caenorhabditis elegans UNC-73B PH domain demonstrates a role in activation of the Rac GTPase in vitro and axon guidance in vivo. Mol Cell Biol 2003; 23:6823 - 6835
- Honigberg L, Kenyon C. Establishment of left/right asymmetry in neuroblast migration by UNC-40/DCC, UNC-73/Trio and DPY-19 proteins in C. elegans. Development 2000; 127:4655 - 4668
- Manser E, Loo TH, Koh CG, Zhao ZS, Chen XQ, Tan L, et al. PAK kinases are directly coupled to the PIX family of nucleotide exchange factors. Mol Cell 1998; 1:183 - 192
- Lucanic M, Cheng HJ. A RAC/CDC-42-independent GIT/PIX/PAK signaling pathway mediates cell migration in C. elegans. PLoS Genet 2008; 4:1000269
- Brenner S. The genetics of Caenorhabditis elegans. Genetics 1974; 77:71 - 94
- Sulston J, Hodgkin J. Wood WB. Methods. The Nematode Caenorhabditis elegans 1988; Cold Spring Harbor, New York Cold Spring Harbor Laboratory Press 587 - 606
- Chapman JO, Li H, Lundquist EA. The MIG-15 NIK kinase acts cell-autonomously in neuroblast polarization and migration in C. elegans. Developmental biology 2008; 324:245 - 257
- Mello C, Fire A. DNA transformation. Methods Cell Biol 1995; 48:451 - 482
- Chalfie M, Sulston J. Developmental genetics of the mechanosensory neurons of Caenorhabditis elegans. Dev Biol 1981; 82:358 - 370
- Sulston JE, Horvitz HR. Post-embryonic cell lineages of the nematode, Caenorhabditis elegans. Dev Biol 1977; 56:110 - 156
- White JG, Southgate E, Thomson JN, Brenner S. The structure of the nervous system of the nematode Caenorhabditis elegans. Philos Trans R Soc Lond 1986; 314:1 - 340
- Reddien PW, Horvitz HR. CED-2/CrkII and CED-10/Rac control phagocytosis and cell migration in Caenorhabditis elegans. Nat Cell Biol 2000; 2:131 - 136
- Zipkin ID, Kindt RM, Kenyon CJ. Role of a new Rho family member in cell migration and axon guidance in C. elegans. Cell 1997; 90:883 - 894
- Shakir MA, Gill JS, Lundquist EA. Interactions of UNC-34 Enabled with Rac GTPases and the NIK kinase MIG-15 in Caenorhabditis elegans axon path-finding and neuronal migration. Genetics 2006; 172:893 - 913
- Ou G, Vale RD. Molecular signatures of cell migration in C. elegans Q neuroblasts. J Cell Biol 2009; 185:77 - 85
- Salser SJ, Kenyon C. Activation of a C. elegans Antennapedia homologue in migrating cells controls their direction of migration. Nature 1992; 355:255 - 258
- Kenyon C. A gene involved in the development of the posterior body region of C. elegans. Cell 1986; 46:477 - 487
- Chalfie M, Thomson JN, Sulston JE. Induction of neuronal branching in Caenorhabditis elegans. Science 1983; 221:61 - 63
- Eisenmann DM, Kim SK. Protruding vulva mutants identify novel loci and Wnt signaling factors that function during Caenorhabditis elegans vulva development. Genetics 2000; 156:1097 - 1116
- Harris J, Honigberg L, Robinson N, Kenyon C. Neuronal cell migration in C. elegans: regulation of Hox gene expression and cell position. Development 1996; 122:3117 - 3131
- Herman M. C. elegans POP-1/TCF functions in a canonical Wnt pathway that controls cell migration and in a noncanonical Wnt pathway that controls cell polarity. Development 2001; 128:581 - 590
- Korswagen HC, Herman MA, Clevers HC. Distinct beta-catenins mediate adhesion and signalling functions in C. elegans. Nature 2000; 406:527 - 532
- Whangbo J, Kenyon C. A Wnt signaling system that specifies two patterns of cell migration in C. elegans. Mol Cell 1999; 4:851 - 858
- Kishore RS, Sundaram MV. ced-10 Rac and mig-2 function redundantly and act with unc-73 trio to control the orientation of vulval cell divisions and migrations in Caenorhabditis elegans. Dev Biol 2002; 241:339 - 348
- Mackay DJ, Hall A. Rho GTPases. J Biol Chem 1998; 273:20685 - 20688
- Kay AJ, Hunter CP. CDC-42 regulates PAR protein localization and function to control cellular and embryonic polarity in C. elegans. Curr Biol 2001; 11:474 - 481
- Debant A, Serra-Pages C, Seipel K, O'Brien S, Tang M, Park SH, et al. The multidomain protein Trio binds the LAR transmembrane tyrosine phosphatase, contains a protein kinase domain and has separate rac-specific and rho-specific guanine nucleotide exchange factor domains. Proc Natl Acad Sci USA 1996; 93:5466 - 5471