Abstract
How cells sense and respond to mechanical forces is attracting considerable attention. We recently demonstrated that mechanical tension originating from one tissue strongly influences the differentiation and morphogenesis of another tissue during C. elegans embryogenesis (Nature 471:99–103). Specifically, we found that the repeated contractions of muscle cells stimulate a signaling cascade involving the Rac GTPase within the epidermis. This pathway ultimately leads to strengthen hemidesmosome-like junctions and promote embryonic morphogenesis. Our work provides further evidence that mechanical inputs impact on development, much like inputs involving growth factors and morphogens. After briefly outlining the pioneering work that inspired us, I will present the mechanotransduction process underlying the response to tension and the key experiments supporting our conclusions.
The notion that mechanical forces could play an essential role in organ development, function and disease, is old.Citation1 However, the mechanisms by which cells sense tension or pressure and measure stiffness remain poorly defined.
Genetic and biochemical studies over the past three decades identified most signaling components mediating signal transduction by growth factors and morphogens during embryonic development.Citation2 We understand to a large extent how signals spread and can be turned off, what is their kinetics. Aside from these studies, recent work has begun to reveal how mechanical forces contribute to shape embryos and organs. Studies on vertebrate embryos have emphasized the role of cortical tension, stiffness and differential adhesion in tissue morphogenesis.Citation3-Citation5 Work on invertebrate embryos has emphasized the key role of myosin II pulses in directing cell shape changes, and how mechanical forces help coordinate the morphogenesis of tissues including different cell types.Citation6-Citation8 It also revealed that compression can upregulate gene expression.Citation9 What remains to be discovered is which cellular processes mechanical forces can influence, and which molecular mechanisms mediate mechanosensing and mechanotransduction in vivo during development.
Experiments conducted with culture cells using well-defined substrates and micro-patterns, or using biophysical methods have outlined some of the principles involved in responding to mechanical forces. The primary receptors to tension are integrins, which are connected to the cytoskeleton and to the ECM. Numerous studies have established that mechanical forces can unfold proteins, expose partially hidden phosphorylation or binding sites, or induce catch bond formation.Citation10 Below, I first outline some critical findings that paved the way to our demonstration that mechanotransduction plays a key role in vivo during embryonic morphogenesis.
Tissue Culture Paradigms
In the late 90s and early 2000s, several papers examined the relationship between tension, the maturation of focal contacts and signaling through small GTPases, some of which indirectly influenced our work. They generally involved well-controlled external manipulation of mechanical force on the development of focal contacts. Choquet, Sheetz and collaborators first showed that moving a fibronectin-covered bead over the dorsal side of a cell induces a local force applied to integrin receptor.Citation11 Riveline, Bershadsky and their coworkers using a related experimental approach could demonstrate that an external mechanical force promotes focal contact elongation in the direction of the forceCitation12 (). Furthermore, these authors observed that focal contact maturation depends on Rho GTPase and ROCK signaling, and demonstrated that their activities can be bypassed by expression of constitutively active form of the formin isoform mDia.Citation12 These papers outlined how a mechanical force applied from the outside can influence the maturation of an adhesion structure and revealed the involvement of small GTPases in the process.
Figure 1. Comparison between FA maturation/stress fiber reorientation and CeHD maturation. (A) Pulling on the dorsal side of a fibroblast with a fibronectin-covered pipet triggers the growth of focal adhesions (red) along the direction of pulling (from ref. Citation13). (B) Uniaxial cyclic stretching of fibroblasts (double-headed blue arrow) triggers the reorientation of stress fibers (green) perpendicular to the direction of the stretch (from ref. Citation14). (C) Anatomy of the C. elegans embryo (a small portion shown; the intestine is not depicted for clarity). Note that muscles are found basally to the epidermis, but shown above the epidermis to outline their anterior-posterior orientation (A-P). (D) Immunofluorescence pattern of C. elegans embryonic muscles (red) and hemidesmosomes (CeHD, green); top, early contraction stage; bottom, late elongation stage. Regions boxed by a dotted rectangle are magnified on the side; note how CeHDs adopt a dorsal-ventral orientation (bottom). Images reprinted with permission from Zhang et al.Citation15
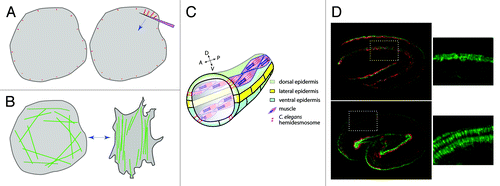
At about the same time, several laboratories reported that cyclic uniaxial stretching of fibroblasts promotes a reorientation of the cell and of its stress fibers perpendicular to the direction of stretchingCitation13 (). Subsequently, several laboratories found that reorientation required specific small GTPases or kinases and that stress fiber reorientation modified Rac activity in specific areas of the cell relative to the direction of the stretch.Citation14,Citation16,Citation17 These papers underlined that cyclic stretch can reorient stress fibers and modify signaling.
The C. elegans Elongation Process and Background Anatomy
C. elegans embryos elongate 4-fold along their anterior/posterior (A/P) axis within less than 3 h, with a concomitant reduction of their diameter. Elongation does not involve cell intercalation as in the fly germband nor cell division.Citation18 Instead, each epidermal cell shrinks along the circumference and lengthens along the A/P axis, while maintaining contacts with the same cells.
Genetic analysis in the past 20 y has established that epidermal cells play a major role in driving embryonic elongation; cytoskeletal remodelling, cell-cell junctions as well as epidermal cell-ECM junctions are required to achieve elongation.Citation18 Twenty years ago, Waterston and colleagues reported that embryonic elongation also requires intact muscles,Citation19 since embryos with defective muscles arrest midway in elongation. However, how muscles could contribute to elongation has long remained a mystery.
Muscle cells are separated from the dorsal and ventral epidermal cells by an extracellular matrix enriched in perlecan (and). Muscle contractions generate body movements because muscles are fastened to the external exoskeleton (cuticle) through trans-epidermal attachments. These correspond to two hemidesmosome-like units at the apical and basal epidermal plasma membranes, which are bridged by intermediate filaments (called IFA-3/IFB-1).Citation20 Reasons to believe that they correspond to hemidesmosome-like units are 3-fold: (1) they form electron-dense plaques; (2) a central component of these units, called VAB-10A, is the C. elegans Plectin and BPAG1e homolog; (3) loss of VAB-10A, of intermediate filaments or of other hemidesmosome components leads to defects reminiscent of human epidermolysis bullosa simplex.Citation20 Besides VAB-10A and intermediate filaments, these hemidesmosomes include distinct ECM-receptors at the apical and basal plasma membrane (both different from α6β4-integrin), and homologs of EPS8 and Kank1.Citation20 Their loss causes embryos to arrest elongation before the 2-fold stage.Citation20 These hemidesmosome-like junctions mature and reorganize during embryonic morphogenesis: they initially form a narrow dotted pattern, and progressively form short circumferential fibers above muscles oriented perpendicular to the anterior-posterior direction of muscle contraction ().
Figure 2. Anatomy of CeHD and mechanotransduction model (A) anterior-posterior transverse section along muscles and CeHDs. There are two CeHDs, one basal in contact with the extacellular matrix (ECM) separating muscles from the epidermis, the other apical in contact with the cuticle. Major components are shown on the right. Apical and basal ECM-receptors have no vertebrate counterparts. (B) Repeated contractions of muscles stretch and compress the epidermis. Genetic studies have outlined two consequences: one, well-characterized except for a predicted conformational change, leads to CeHD strenghtening; the other, poorly characterized (see question mark), promotes non-muscle myosin II activity. Both concur to promote embryonic elongation. Adapted from Zhang et al.Citation15
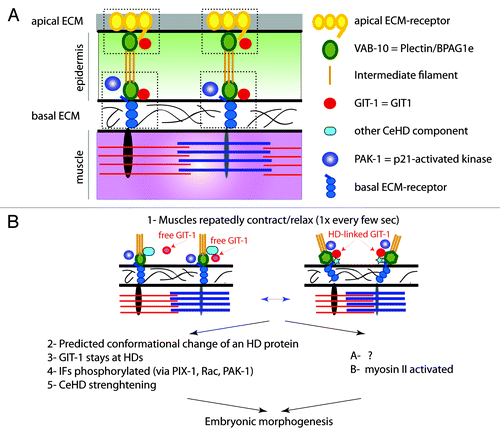
Signaling Between Tissues through a GIT/PIX/Rac Mechanotransduction Pathway
Our goal was to define why mutants with defective muscles arrest elongation at the 2-fold stage. Given the prominent role played by the epidermis during elongation, we assumed that muscles are likely to send a signal to the epidermis. The question was which signal and how is it sensed.
Comparison with tissue culture cells suggested a potential scenario: (1) muscle contractions might induce a tensile force on the epidermis, which could in turn promote hemidesmosome maturation, much like localized pulling on focal adhesions promotes their maturation; (2) furthermore, as observed for fibroblast stress fibers during cyclic stretch, the repeated muscle contractions could promote hemidesmosomes to organize perpendicularly to the direction of contraction. To test these ideas, we first examined whether muscle contractions indeed stretch or compress epidermal cells, and second we performed a genetic screen to identify proteins that might respond to stretch.
Using fiducial landmarks, we could indeed demonstrate that muscle contractions locally stretch and compress the epidermis along the anterior-posterior axis. Quantification showed that the local magnitude of compression is up to 50% the distance observed when muscles are relaxed, and that it can last for up to 2 sec.Citation15 Typical stretching protocols induce a 5–18% planar strain at a frequency of 0.5–1 Hz.Citation14,Citation16,Citation17 Hence, a mechanical signal from muscles to the epidermis was plausible.
To identify proteins involved in sensing and transducing this mechanical input, we reasoned that such proteins would likely associate with the hemidesmosome-like junction, given its anatomical and physiological roles. We thus searched for novel components of these junctions, starting from a mutation in the Plectin/BPAG1e component that only partially compromised the hemidesmosomes. We looked in a systematic RNAi screen for gene knockdowns that would further damage hemidesmosomes.Citation21 One protein identified in this screen corresponded to the C. elegans homolog of the p21-activated kinase PAK-1.
After establishing that PAK-1 is primarily located at hemidesmosome-like junctions, we showed that loss of PAK-1 kinase activity combined with the weak mutation in the Plectin/BPAG1e strongly hampers intermediate filament recruitment to hemidesmosomes.Citation15 A key observation was our demonstration that PAK-1 activity mediates intermediate filament phosphorylation, and that intermediate filament phosphorylation requires muscle contractility, strongly suggesting that muscle tension activates PAK-1 activity.
From there on, we aimed at defining how PAK-1 is activated. Since PAK is a classical Rac/ Cdc42 effector, and since Rac can respond to stretch,Citation14,Citation16,Citation17 we tested their implication in relaying muscle tension. We established in three ways that the Rac GTPase (CED-10 in C. elegans) is involved: (1) combined with the weak vab-10A allele, a ced-10 mutation mimics the phenotype of pak-1 mutations; (2) conversely, a constitutively active CED-10(G12V) form rescues the lack of intermediate filament phosphorylation observed in muscle mutants; (3) GTP-bound Rac/CED-10 is about twice less abundant in the epidermis of muscle mutants. Looking for the probable guanine nucleotide exchange factor (GEF) acting in the pathway, we proved that homologs of β-PIX and of its interacting partner GIT1 (GRK-interacting protein 1) activate CED-10 in response to muscle tension.Citation15 Both β-PIX and GIT1 homologs (PIX-1 and GIT-1, respectively), like PAK-1, are located at hemidesmosomes. Two recent studies found that PIX-1 and GIT-1 promotes gonad tip cell migration in a Rac-independent manner, whereas PIX-1 stimulates the migration of certain neurons through CED-10/Rac.Citation22,Citation23 Thus, depending on the tissue and probably the input, GIT-1/PIX-1 act in the same pathway or in parallel to Rac.
Interestingly, GIT-1 localization to hemidesmosomes depends on muscle contractions, since the protein becomes cytoplasmic in mutants with defective muscles (). Moreover, we could restore the hemidesmosomal localization of GIT-1 in a muscle mutant by cyclically compressing the embryo from the outside.Citation15 Our data also suggest that in a parallel process tensile stress promotes myosin II activity in the epidermis.
Our biochemical, genetic and cellular data together suggest a model whereby muscle-induced shear stress on the epidermis induces the recruitment of GIT-1 to hemidesmosomes (). In turn, it activates PIX-1, Rac and PAK-1 to mediate intermediate filament phosphorylation and junction maturation. It seems unlikely that GIT-1 is the direct stress sensor. Instead, much like pulling on FAs partially unfolds talin to induce vinculin recruitment and FA maturation,Citation24 we predict that a hemidesmosomal component undergoes a conformational change under tension to create a binding pocket for GIT-1 and initiate signaling. Intriguingly, during FA maturation β-PIX appears recruited to nascent focal adhesions when tension is lower.Citation25 It is unclear whether the different behaviors of β-PIX/PIX-1 in vertebrate FAs and C. elegans hemidesmosomes, respectively, are just apparent or reflect differences of junction and species.
Our general conclusions are that the C. elegans hemidesmosome-like junction can mediate a mechanotransduction process.Citation15 By extension, we propose that vertebrate hemidesmosomes should also be endowed with similar properties. Consistent with this notion, the dystroglycan-plectin complex plays a role in relaying mechanical stress in alveolar epithelial cells to activate ERK1/2 and AMPK (adenosine 5′-monophosphate-activated protein kinase) activities.Citation26
Conclusion: A Broader Outlook at Mechanotransduction
The classical view in developmental biology has long held that patterning involves biochemical cues, such as morphogen gradients or ion fluxes. The importance of mechanical cues in patterning embryonic patterning is beginning to surface.Citation27 We are still far from having a complete picture of the cellular processes or entities that can be affected by mechanical forces. Our work reveals that intermediate filaments can represent a downstream target of mechanotransduction. Our study also reveals that repeated, cyclic tensile stress can locally pattern junctions and promote epithelial morphogenesis. We would expect that in other situations in which contractile cells are juxtaposed to epithelial cells, the contractions of the former promote the morphogenesis and/or the repair of the latter. Such situations are numerous, since many organs involve an epithelial layer and a smooth muscle or a myoepithelial layer. For instance, myofibroblasts are essential to complete tissue repairCitation28 and myoepithelial cells contribute to regulate mammary gland branching and elongation.Citation29 It will be important to define whether these contractile cells signal through mechanical inputs, in addition to their role in releasing specific growth factors and remodelling the extracellular matrix.
Acknowledgments
I thank the Agence Nationale pour la Recherche and the Association pour la Recherche contre le Cancer for funding, as well as the CNRS, the INSERM and Université de Strabsourg for institutional funding.
References
- Thompson DAW. On growth and form. 1917, Cambridge [Eng.]: University press. xv, 793 p.
- Gilbert SF. Developmental biology. 9th ed. 2010, Sunderland, Mass.: Sinauer Associates. xxi, 711, [80] p.
- Keller R, Davidson LA, Shook DR. How we are shaped: the biomechanics of gastrulation. Differentiation 2003; 71:171 - 205; http://dx.doi.org/10.1046/j.1432-0436.2003.710301.x; PMID: 12694202
- Krieg M, Arboleda-Estudillo Y, Puech PH, Käfer J, Graner F, Müller DJ, et al. Tensile forces govern germ-layer organization in zebrafish. Nat Cell Biol 2008; 10:429 - 36; http://dx.doi.org/10.1038/ncb1705; PMID: 18364700
- Steinberg MS. On the mechanism of tissue reconstruction by dissociated cells. I. Population kinetics, differential adhesiveness. and the absence of directed migration. Proc Natl Acad Sci USA 1962; 48:1577 - 82; http://dx.doi.org/10.1073/pnas.48.9.1577; PMID: 13916689
- Zhang H, Gally C, Labouesse M. Tissue morphogenesis: how multiple cells cooperate to generate a tissue. Curr Opin Cell Biol 2010; 22:575 - 82; http://dx.doi.org/10.1016/j.ceb.2010.08.011; PMID: 20822890
- Butler LC, Blanchard GB, Kabla AJ, Lawrence NJ, Welchman DP, Mahadevan L, et al. Cell shape changes indicate a role for extrinsic tensile forces in Drosophila germ-band extension. Nat Cell Biol 2009; 11:859 - 64; http://dx.doi.org/10.1038/ncb1894; PMID: 19503074
- Solon J, Kaya-Copur A, Colombelli J, Brunner D. Pulsed forces timed by a ratchet-like mechanism drive directed tissue movement during dorsal closure. Cell 2009; 137:1331 - 42; http://dx.doi.org/10.1016/j.cell.2009.03.050; PMID: 19563762
- Desprat N, Supatto W, Pouille PA, Beaurepaire E, Farge E. Tissue deformation modulates twist expression to determine anterior midgut differentiation in Drosophila embryos. Dev Cell 2008; 15:470 - 7; http://dx.doi.org/10.1016/j.devcel.2008.07.009; PMID: 18804441
- Moore SW, Roca-Cusachs P, Sheetz MP. Stretchy proteins on stretchy substrates: the important elements of integrin-mediated rigidity sensing. Dev Cell 2010; 19:194 - 206; http://dx.doi.org/10.1016/j.devcel.2010.07.018; PMID: 20708583
- Choquet D, Felsenfeld DP, Sheetz MP. Extracellular matrix rigidity causes strengthening of integrin-cytoskeleton linkages. Cell 1997; 88:39 - 48; http://dx.doi.org/10.1016/S0092-8674(00)81856-5; PMID: 9019403
- Riveline D, Zamir E, Balaban NQ, Schwarz US, Ishizaki T, Narumiya S, et al. Focal contacts as mechanosensors: externally applied local mechanical force induces growth of focal contacts by an mDia1-dependent and ROCK-independent mechanism. J Cell Biol 2001; 153:1175 - 86; http://dx.doi.org/10.1083/jcb.153.6.1175; PMID: 11402062
- Shirinsky VP, Antonov AS, Birukov KG, Sobolevsky AV, Romanov YA, Kabaeva NV, et al. Mechano-chemical control of human endothelium orientation and size. J Cell Biol 1989; 109:331 - 9; http://dx.doi.org/10.1083/jcb.109.1.331; PMID: 2545727
- Katsumi A, Milanini J, Kiosses WB, del Pozo MA, Kaunas R, Chien S, et al. Effects of cell tension on the small GTPase Rac. J Cell Biol 2002; 158:153 - 64; http://dx.doi.org/10.1083/jcb.200201105; PMID: 12105187
- Zhang H, Landmann F, Zahreddine H, Rodriguez D, Koch M, Labouesse M. A tension-induced mechanotransduction pathway promotes epithelial morphogenesis. Nature 2011; 471:99 - 103; http://dx.doi.org/10.1038/nature09765; PMID: 21368832
- Kaunas R, Nguyen P, Usami S, Chien S. Cooperative effects of Rho and mechanical stretch on stress fiber organization. Proc Natl Acad Sci USA 2005; 102:15895 - 900; http://dx.doi.org/10.1073/pnas.0506041102; PMID: 16247009
- Shikata Y, Rios A, Kawkitinarong K, DePaola N, Garcia JG, Birukov KG. Differential effects of shear stress and cyclic stretch on focal adhesion remodeling, site-specific FAK phosphorylation, and small GTPases in human lung endothelial cells. Exp Cell Res 2005; 304:40 - 9; http://dx.doi.org/10.1016/j.yexcr.2004.11.001; PMID: 15707572
- Chisholm AD, Hardin J. Epidermal morphogenesis WormBook, ed. e.T.C.e.R.C. WormBook. 2005: WormBook, doi/10.1895/wormbook.1.7.1, http://www.wormbook.org.
- Williams BD, Waterston RH. Genes critical for muscle development and function in Caenorhabditis elegans identified through lethal mutations. J Cell Biol 1994; 124:475 - 90; http://dx.doi.org/10.1083/jcb.124.4.475; PMID: 8106547
- Zhang H, Labouesse M. The making of hemidesmosome structures in vivo. Dev Dyn 2010; 239:1465 - 76; PMID: 20205195
- Zahreddine H, Zhang H, Diogon M, Nagamatsu Y, Labouesse M. CRT-1/calreticulin and the E3 ligase EEL-1/HUWE1 control hemidesmosome maturation in C. elegans development. Curr Biol 2010; 20:322 - 7; http://dx.doi.org/10.1016/j.cub.2009.12.061; PMID: 20153198
- Dyer JO, Demarco RS, Lundquist EA. Distinct roles of Rac GTPases and the UNC-73/Trio and PIX-1 Rac GTP exchange factors in neuroblast protrusion and migration in C. elegans. Small GTPases 2010; 1:44 - 61; http://dx.doi.org/10.4161/sgtp.1.1.12991; PMID: 21686119
- Lucanic M, Cheng HJ. A RAC/CDC-42-independent GIT/PIX/PAK signaling pathway mediates cell migration in C. elegans. PLoS Genet 2008; 4:e1000269; http://dx.doi.org/10.1371/journal.pgen.1000269; PMID: 19023419
- del Rio A, Perez-Jimenez R, Liu R, Roca-Cusachs P, Fernandez JM, Sheetz MP. Stretching single talin rod molecules activates vinculin binding. Science 2009; 323:638 - 41; http://dx.doi.org/10.1126/science.1162912; PMID: 19179532
- Kuo JC, Han X, Hsiao CT, Yates JR 3rd, Waterman CM. Analysis of the myosin-II-responsive focal adhesion proteome reveals a role for beta-Pix in negative regulation of focal adhesion maturation. Nat Cell Biol 2011; 13:383 - 93; http://dx.doi.org/10.1038/ncb2216; PMID: 21423176
- Takawira D, Budinger GR, Hopkinson SB, Jones JC. A dystroglycan/plectin scaffold mediates mechanical pathway bifurcation in lung epithelial cells. J Biol Chem 2011; 286:6301 - 10; http://dx.doi.org/10.1074/jbc.M110.178988; PMID: 21149456
- Farge E. Mechanotransduction in development. Curr Top Dev Biol 2011; 95:243 - 65; http://dx.doi.org/10.1016/B978-0-12-385065-2.00008-6; PMID: 21501754
- Hinz B. The myofibroblast: paradigm for a mechanically active cell. J Biomech 2010; 43:146 - 55; http://dx.doi.org/10.1016/j.jbiomech.2009.09.020; PMID: 19800625
- Ewald AJ, Brenot A, Duong M, Chan BS, Werb Z. Collective epithelial migration and cell rearrangements drive mammary branching morphogenesis. Dev Cell 2008; 14:570 - 81; http://dx.doi.org/10.1016/j.devcel.2008.03.003; PMID: 18410732