Abstract
Milk proteins including casein are sources of peptides with bioactivity. One of these peptides is beta-casomorphin (BCM) which belongs to a group of opioid peptides formed from β-casein variants. Beta-casomorphin 7 (BCM7) has been demonstrated to be enzymatically released from the A1 or B β-casein variant. Epidemiological evidence suggests the peptide BCM 7 is a risk factor for development of human diseases, including increased risk of type 1 diabetes and cardiovascular diseases but this has not been thoroughly substantiated by research studies. High performance liquid chromatography coupled to UV-Vis and mass spectrometry detection as well as enzyme–linked immunosorbent assay (ELISA) has been used to analyze BCMs in dairy products. BCMs have been detected in raw cow's milk and human milk and a variety of commercial cheeses, but their presence has yet to be confirmed in commercial yoghurts. The finding that BCMs are present in cheese suggests they could also form in yoghurt, but be degraded during yoghurt processing. Whether BCMs do form in yoghurt and the amount of BCM forming or degrading at different processing steps needs further investigation and possibly will depend on the heat treatment and fermentation process used, but it remains an intriguing unknown.
INTRODUCTION
Milk protein is a source of peptides that are bioactive (Rizzello et al., Citation2005) including: angiotensin-converting enzyme inhibitory (ACE-I) activity (Minervini et al., Citation2003; Donkor et al., Citation2007); antihypertensive activity (Muguerza et al., Citation2006), antibacterial activity (Minervini et al., Citation2003), and opioid activity (De Noni and Cattaneo, Citation2010). The term “opioid” is used for substances having morphine-like activity that act by binding to opioid receptors. Opioid substances are found in the center nervous system and the gastrointestinal tract. These peptides play a crucial role in the response to pain and stress (Kamiński et al., Citation2007).
Beta-casomorphins (BCMs) are a group of peptides with opioid properties and are formed from proteolytic digestion of β-casein (De Noni and Cattaneo, Citation2010). Among these peptides, only beta-casomorphin 7 (BCM7) has been widely studied. The release of BCM7 during hydrolysis of β-casein depends on the β-casein variant containing a histidine residue at position 67. In recent years, epidemiological evidence has indicated that consumption of milk containing the A1 variant is linked to increased risk of type 1 diabetes and heart disease (Elliott et al., Citation1999; McLachlan, Citation2001; Laugesen and Elliott, Citation2003). The European Food Safety Authority (EFSA) concluded there was insufficient data to determine a causal relationship between BCM7 exposure and non-communicable diseases (EFSA, Citation2009). Nevertheless the presence of these peptides in dairy products needs further research due to their putative link to elevated chronic disease risk.
The BCM7 peptide has been identified in raw milk (Cieślińska et al., Citation2007; Cieślińska et al., Citation2012) and some cheeses (Jarmolowska et al., Citation1999; De Noni and Cattaneo, Citation2010), but was not found in commercial yoghurt (Kahala et al., Citation1993; Schieber and Brückner, Citation2000; De Noni and Cattaneo, Citation2010). Whether BCMs do form and the amount of BCM forming at different processing steps needs further investigation and possibly will depend on the heat treatment and fermentation process but remains an intriguing unknown.
Analytical trends in the analysis of BCMs indicate reverse phase high performance liquid chromatography (RP-HPLC) as the preferred choice for separation of these polar, nonvolatile peptides in a variety of dairy products. For detection, UV-Vis absorbance has been widely used in a number of analytical laboratories around the world (Muehlenkamp and Warthesen, Citation1996). Nowadays, at the forefront of detection techniques, is mass spectrometry coupled to electrospray ionization (ESI) which is by far the most reliable detection technique for small peptides (De Noni and Cattaneo, Citation2010). Alternatively, enzyme–linked immunosorbent assay (ELISA) has been applied to detect and quantify BCMs in dairy products (Sienkiewicz-Szłapka et al., Citation2009; Cieślińska et al., Citation2012).
This review aims to bring together the current knowledge on BCMs, analyzing their possible sources and presence in dairy products such as milk, cheese, and yogurt. The impacts of processing conditions such as thermal treatment, fermentation, and cross link on formation and degradation of BCMs in dairy products are also discussed. Finally, the advantages and limitations of each analytical technique currently in use to identify and quantify BCMs are also reported along with some examples of applications taken from the current scientific literature.
SOURCES OF BETA-CASOMORPHINS
Protein in bovine milk contains four caseins αs1, αs2, β, and κ-casein and two major whey proteins, β-lactoglobulin and α-lactoalbumin (Kamiński et al., Citation2007). In recent years, the 209 amino acid residues in the chains of β-casein () (Swaigood, Citation2003; Truswell, Citation2005) have been studied extensively. It was found that there are 13 variants present in bovine milk including A1, A2, A3, A4, B, C, D, E, F, H1, H2, I, and H (Kamiński et al., Citation2007), of which A1 and A2 are the most common variants. The primary structure of some variants is showed at . Milk from European breeds such as Holstein–Friesian mainly contains A1 β-casein (Tailford et al., Citation2003), while A2 β-casein is commonly detected in milk from Guernsey and Jersey breeds (Bell et al., Citation2006; Merriman, Citation2009). The difference between A1 and A2 variant is a single amino acid at position 67 on the chain; being histidine in A1 and proline in A2 (Truswell, Citation2005; Kamiński et al., Citation2007).
Figure 1. The primary structure of β-casein A2 indicating amino acid substitution of the variants (adapted from Swaigood, 2003).
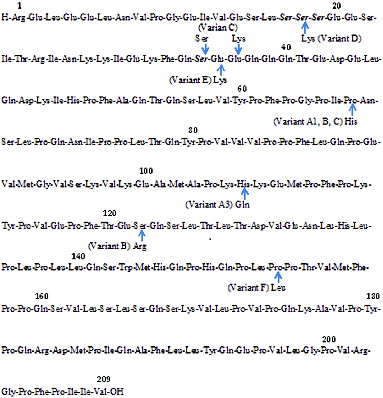
Interestingly, this structural difference leads to different properties when the two β-casein variants are hydrolyzed in the intestine. According to Jinsmaa and Yoshikawa (Citation1999), the peptide bond between proline and isoleucine in the A2 variant has more enzymatic resistance than that between histidine and isoleucine in the A1 variant. So A1 β-casein is more easily hydrolyzed at this residue by gastrointestinal enzymes, resulting in the release of BCM7 (Jinsmaa and Yoshikawa, Citation1999). Consequently, BCM7 is liberated in the digestive tract from β-caseins containing histidine residue at 67 position, including A1 as well as B β-casein ().
BCMs are a group of structurally similar peptides () with a sequence of 4–11 amino acids (Kamiński et al., Citation2007). All BCMs have the same sequence for the first three amino acids, tyrosine, proline, and phenylalanine (Muehlenkamp and Warthesen, Citation1996), and have been identified in and isolated from bovine milk (Kamiński et al., Citation2007; EFSA, Citation2009). These peptides are released from β-casein at position 60 which is the tyrosine residue and the other residues are released from other positions () if the conditions are right.
Table 1 BCMs released from bovine milk
PRESENCE OF BETA-CASOMORPHINS IN DAIRY PRODUCTS
Milk
Bovine milk is a crucial food for humans as a source not only of high quality protein, fat, minerals, and vitamins (Bell et al., Citation2006; Kamiński et al., Citation2007; Nagpal et al., Citation2011), but also of bioactive peptides that are linked to positive health effects on the cardiovascular, nervous, and gastrointestinal systems (Choi et al., Citation2012). Consumption of milk and dairy products may play an important role for prevention of a number of diseases such as hypertension (Jauhiainen and Korpela, Citation2007), osteoporosis (Uenishi, Citation2006), obesity (Jaffiol, Citation2008), dental decay (Shimazaki et al., Citation2008) and cardiovascular disease (Elwood et al., Citation2004a, Citation2004b; Elwood, Citation2005; Elwood et al., Citation2005). In addition, bovine milk also contains peptides with opioid properties, for instance BCM7 that may increase risk of chronic disease (Cieślińska et al., Citation2007; Cieślińska et al., Citation2012). Epidemiologically, however, intake of milk containing A1 β-casein strongly correlates with ischemic heart disease (McLachlan, Citation2001; Laugesen and Elliott, Citation2003) and type 1 diabetes (Elliott et al., Citation1999; McLachlan, Citation2001; Birgisdottir et al., Citation2006). This implies that BCM7 released from A1-β casein may be a significant contributor to the risk of cardiovascular disease and type 1 diabetes.
Bovine milk naturally contains somatic cell, endogenous proteases, and unwanted bacteria, which may hydrolyze β-casein into peptides, including BCMs. Cieślińska et al. (Citation2007) detected BCM7 in unprocessed cow milk but this study did not characterize whether somatic cells were present. Therefore, the presence of BCM7 in bovine milk may have been a result of microbial or somatic cell-derived enzyme activity (EFSA, Citation2009) rather than BCM formation in the mammary gland. However, Napoli et al. (Citation2007) showed that BCM7 was not detected in milk from cows with mastitis, where concentration of somatic cells exceeds 500,000 per milliliter (Ginn et al., Citation1985). So high levels of somatic cell may degrade BCM7; but this needs further investigation. Similarly, Cieślińska et al. (Citation2012) investigated the presence of BCM7 in bovine milk collected from healthy cows which did not indicate any subclinical symptom of mastitis and showed that BCM7 was detected in all milk samples with the highest amount in A1 milk, followed by A1A2 milk and A2 milk. Surprisingly, BCM7 was found in the milk containing the A1 variant but also in A2 variant-containing milk. However, the authors did not characterize the number of bacterial cells, the conditions and duration of storage of samples, or the presence of plasmin, an endogenous protease present in milk. Consequently, it is possible that the release of BCM7 may involve hydrolysis by bacterial-derived enzymes or endogenous enzymes of A1 β-casein to form BCM7. This research, however, still contradicts other studies that have not found BCM7 in milk of the A2 β-casein variant.
Findings in human milk may assist in our understanding of the origin and fate of BCMs in bovine milk. For instance, Jarmołowska et al. (Citation2007) reported that beta-casomorphin 5 (BCM5) and BCM7 were found in human colostrums and mature milk; the BCM content of the former being much higher than that of the later. According to the authors, milk samples were selected from healthy women and frozen immediately after expression and stored at −70°C; excluding the possibility of formation of BCMs by somatic cell and bacterial enzyme action. These findings indicate that BCMs may be released from β-casein in the mammary gland by endogenous enzyme activity (Koch et al., Citation1988). Endogenous enzyme activity may also result in peptide formation during the manufacture of cheese and other fermented dairy products as will be described in the following sections.
Cheese
Cheese is a dairy product that is a commonly consumed part of the diet in Western countries, and is commonly produced from bovine milk. Major steps in cheese manufacture include heat treatment of milk, coagulation with rennet and starter culture, separation of curd from whey, and ripening of curd to produce the final cheese. Heat treatment of milk is commonly done by pasteurization at 72°C for 15 seconds (Hayaloglu et al., Citation2010). Cheese studies that used milk heated at above 72°C involving peptide profiles (Hougaard et al., Citation2010) showed denaturation of whey protein, leading to the interaction between whey protein and casein. High temperature pretreatment may significantly affect proteolysis of cheese during ripening as well as its final flavor and texture (Benfeldt and Sørensen, Citation2001). Protelolysis during ripening may involve the formation of large peptides, some of which are subsequently degraded into smaller peptides that may include pro-BCMs and BCMs as well as free amino acids and aroma compounds (Benfeldt and Sørensen, Citation2001).
Currently, only a few studies have investigated the formation of BCMs in cheese varieties (Sienkiewicz-Szłapka et al., Citation2009; De Noni and Cattaneo, Citation2010). In Brie cheese, BCM7 was found in the range 5 to 15 μg/g following treatments of different ripening times by Jarmołowska et al. (Citation1999), whereas De Noni and Cattaneo (Citation2010) detected only 0.15 μg/g and Sienkiewicz-Szłapka et al. (Citation2009) found 6.48 μg/g. BCM7 was found 0.01 to 0.11 μg/g in Gorgonzola, Gouda, Fontina, and Cheddar (De Noni and Cattaneo, Citation2010). Similarly, Sienkiewicz-Szłapka et al. (Citation2009) quantified BCM7 in some locally produced Polish mould cheeses (Brie and Rokpol) and determined that levels were much higher than that of the semi-hard cheeses they analyzed (Edamski, Gouda and Kasztelan). The levels of BCM7 and other related BCMs reported in some cheeses are shown in . In addition to BCM7, BCM5 was found in Brie, Rokpol, Edamski, Gouda, and Kasztelan (Sienkiewicz-Szłapka et al., Citation2009), BCM9 (Saito et al., Citation2000; Toelstede and Hofmann, Citation2008) and BCM10 in Gouda (Toelstede and Hofmann, Citation2008) and BCM 11 in Caprino del Piemonte, an Italian goat cheese (Rizzello et al., Citation2005). In contrast, Muehlenkamp and Warthesen (Citation1996) reported that BCM7 were not detected in Brie and Cheddar. According to the authors, the absence of BCM7 in these products may be due to (a) nonformation of BCMs from β-casein, (b) degradation of BCM7 during ripening, or (c) smaller amounts of BCM7 than the minimum detectable level by RP-HPLC (<2 μg/mL of cheese extract).
Table 2 BCMs in cheeses
The formation of BCM7 in some cheeses is due to the digestion of β-casein with starter culture derived enzymes. By using a HPLC-MS/MS method, De Noni and Cattaneo (Citation2010) found 0.11 μg/g of BCM7 in Cheddar cheese. According to Jarmolowska et al. (Citation1999), the presence of BCM7 in cheese does not originate from that originally present in milk, because milk-derived peptides would be removed from the curd during the drainage of whey. Cheddar cheese making commonly use starter cultures which are a mixture of Lactococcus. lactic ssp. cremoris and L. lactic ssp. lactic (Robinson, Citation1995). Muehlenkamp and Warthesen (Citation1996) showed that enzymes derived from L. lactic ssp. cremoris are capable of reducing the amount of BCM7 found by 50% at pH 5.0 and 1.5% NaCl after 6–15 weeks; conditions which are similar to those in Cheddar cheese making. Thus, the concentration of BCM7 in Cheddar cheese at the beginning period of ripening may be higher than that in the ripened cheese reported by De Noni and Cattaneo (Citation2010), since BCM7 may have been formed from β-casein on the addition of starter culture, but degraded into even smaller peptides during ripening.
As well as through starter cultures, there is potential for bioactive peptides including BCMs to be formed by hydrolysis of β-casein by endogenous proteases and nonstarter culture bacterial proteases from contaminated udder, pipes, and equipment (EFSA, Citation2009). However, the endogenous protease plasmin has been shown not to release BCMs (Gaucher et al., Citation2008) and similarly the rennet enzymes used to coagulate milk protein also do not liberate bioactive peptides (McSweeney, Citation2004); excluding these enzymes activities as sources of BCMs. Hayaloglu et al. (Citation2010) investigated the peptide profile of Malatya cheese made from raw milk without addition of starter culture. The authors demonstrated considerably higher concentration of peptides but did not measure BCMs, although they may have been present in this cheese. Hayaloglu et al. (Citation2010) compared to raw milk cheese to cheese made from pasteurized milk with added L. lactis ssp. lactis and S. Thermophilus and suggested that the resultant peptides were as a result of nonstarter culture derived enzyme-activity. However, to date, there is no published paper investigating formation of BCMs by nonstarter culture-derived proteases.
The presence of BCM7 in cheese may also be due to the activity of enzymes derived from mould during ripening. The level of BCM7 in Brie (Sienkiewicz-Szłapka et al., Citation2009; De Noni and Cattaneo, Citation2010) and Rokpol ripened with fungi and lactic acid bacteria (LAB) (Sienkiewicz-Szłapka et al., Citation2009) was considerably higher than that in cheese ripened with only LAB. Similarly, Jarmolowska et al. (Citation1999) also observed a high level of BCM7 in Brie. Consequently, the formation of BCM7 in Brie and Rokpol cheese may be due to proteolytic digestion of protein by both enzymes derived from fungi (De Noni and Cattaneo, Citation2010) and LAB (Jarmolowska et al., Citation1999).
The stability of BCM7 may depend on the type of enzymes present in cheese. Haileselassie et al. (Citation1999) investigated potentially bioactive peptides including BCM7 in Cheddar cheese extract with the addition of the enzyme, Neutrase isolated from B. subtilis. BCM7 was identified but the amount was not reported. However, no BCM7 was detected in samples: (a) prepared from the Cheddar cheese extract with added Neutrase plus a crude extract of L. casei isolated from mild Cheddar cheese or (b) the Cheddar cheese extract with added Neutrase plus a peptidase isolated from Lactococcus lactic and Asperillus oryzae. Haileselassie et al. (Citation1999) suggested that the presence of BCM7 in the cheddar cheese extract with Neutrase was as a result of β-casein hydrolysis by the Neutrase. The absence of BCM7 in the two latter samples may have been as a result of BCM7 hydrolysis by proteases to a level lower than the threshold level of the analysis. Thus, the formation and degradation of BCM7 may involve proteases derived from bacteria used in the starter culture.
The characterization of BCM precursors (i.e. longer peptide chains containing the BCM amino acid sequence) in cheese may support our understanding of the steps involved in BCM formation. In general, proteases digest β-casein into larger peptides including BCM precursors, and subsequently some of precursors are degraded into BCMs by specific peptidases. One BCM precursor found in Crescenza (Smacchi and Gobbetti, Citation1998) and in Cheddar and Jarlsberg cheese (Stepaniak et al., Citation1995) after ripening for 8–10 days was β-CN f58-72 (Smacchi and Gobbetti, Citation1998). This peptide may be further digested into BCM13 (β-CN f60-72) as reported by Jinsmaa and Yoshikawa (Citation1999) and other related BCMs if cheese is aged for a longer period.
As previously described, the formation of BCM7 in cheese depends on β-casein variants present in the milk used for producing the cheese; A1 and B β-casein variants being those most likely to contribute to release of BCM7. Most studies above investigated BCM7 and other related BCMs in commercial cheeses that were not characterized for the β-casein variant in the milk used, except for the study of De Noni and Cattaneo (Citation2010). According to De Noni and Cattaneo (Citation2010), A1, A2, and B variant of β-casein were detected in all cheese samples, so it was unclear which of the variants contributed to the occurrence of BCM7 following fermentation. For elucidating which variant(s) is (are) the source of BCM7 during cheese making, it will be necessary to investigate the formation of BCM7 and other related BCMs in cheese produced from milk of single β-casein variants in future research.
Yoghurt
Yogurt is a popular dairy product usually fermented by two lactic acid bacteria, L. delbrueckii ssp. bulgaricus and S. thermophilus (Tamime and Robinson, Citation1999). Major steps in yoghurt processing include thermal treatment, fermentation, and chilled storage. In addition to inducing changes in pH and viscosity, the yoghurt culture can hydrolyze the milk proteins into peptides with bioactivity. A number of bioactive peptides have been isolated from yoghurt, such as ACE-I peptides (Donkor et al., Citation2007; Kunda et al., Citation2012) and antihypertensive peptides (Schieber and Brückner, Citation2000; Muguerza et al., Citation2006; Kunda et al., Citation2012). However, no study has reported on the formation or degradation of BCMs in yoghurt. The lack of reliable information about BCMs in yoghurt may be in part due to difficulty in identification and quantification of the low levels of these peptides within the complex yoghurt matrix (EFSA, Citation2009).
As reported by Donkor et al (Citation2007), β-casein-derived peptides were isolated from yoghurt inoculated with yoghurt starter culture and probiotic organisms (L. casei L26, L. acidophilus L10 and B. lactis B94). These peptides included different β-CN fragments (β-CN f193-198, f25–29, f69–73 and f84–86) but neither BCMs nor precursors of BCM were identified. Similarly, Schieber and Brückner (Citation2000) identified 30 peptides in yoghurt fermented with L. delbrueckii ssp. bulgaricus and S. salivarius ssp. thermophilus, mainly produced from A1 β-casein. These authors did observe two precursors of BCM, β-CN f57–68, and β-CN f57–72, containing histidine at position 67 of the parent β-casein. This finding suggested that precursors of BCM are more likely to be formed from A1 and B β-casein variant than A2 β-casein (Schieber and Brückner, Citation2000).
According to Kunda et al. (Citation2012), a large number of peptides were formed from both A1 and A2 β-casein variants during yoghurt manufacture, among which a peptide containing the BCM7 sequence, namely β-CN f58–72 was identified. In addition, some peptides identified in yoghurt were released from only the A2 β-casein variant, namely β-CN f59–68 and β-CN f59–70 and these peptides may be further degraded into BCM13, BCM9, and BCM10, respectively. Furthermore, the authors found β-CN f60–72 was liberated from A1 β-casein variant, indicating that yoghurt starter cultures may hydrolyze A1 β-casein variant into BCMs. Several di-peptides have been also identified in yoghurt, such as β-CN f60–61 and β-CN f62–63. Both di-peptides were released from A1 and A2 β-casein variants. As previously described in , amino acids at position 61 and 63 on the chain of parent β-casein are proline residues. Therefore, this finding indicated that yoghurt bacteria-derived enzymes are likely to digest the peptide bonds between proline and other amino acid residues, leading to degradation of BCMs. This degradation of BCMs during yoghurt processing may involve the activity of X-prolyl dipeptidyl aminopeptidase (PepX). PepX is derived from LAB and is specifically hydrolyzes peptide bonds between proline and other residues (Gobbetti, 2002). Similarly, Sabeena Farvin et al. (Citation2010) identified peptide fragments in commercial yogurt within 24 hours of production. Peptides detected included fragments of αs1, αs1, κ-casein, with a majority of fragments from β-casein, from which BCM9 was released from A2 β-casein but BCM7 was not found. The formation of BCMs in cheese processing suggests BCMs may be formed and then degraded during yoghurt processing. Kunda et al. (Citation2012) and Sabeena Farvin et al. (Citation2010) did not isolate and characterize starter cultures present in the yoghurt they investigated, which is a key factor influencing the production and degradation of peptides, including the final BCMs content of the product.
Yogurt inoculated with a mixture of L. delbrueckii ssp. bulgaricus and S. thermophilus was isolated and characterized by De Noni and Cattaneo (Citation2010) in a BCM7 study. The yoghurts did not contain BCM7, although A1, A2, and B β-CN variants were identified in yoghurts. The same results were reported by Schieber and Brückner (Citation2000) investigating yoghurt kept 21 days at 4°C. Thus, it has been suggested that the mixture of starter culture L. delbrueckii ssp. bulgaricus and S. thermophilus is not capable of proteolysis of β-casein to form BCMs. This finding was also supported by work on Taleggio cheese using L. delbrueckii ssp. bulgaricus and S. thermophiles as starter culture, that did not contain BCM7 (De Noni and Cattaneo, Citation2010). However, both the yoghurt and Taleggio cheese used for the analysis of BCM7 were kept for 30 days and 35 days, respectively (De Noni and Cattaneo, Citation2010). As reported by Atlan et al. (Citation1990) PepX is located in cytoplasm, so these long periods of aging possibly resulted in cell lysis, leading to release of PepX that during storage of yoghurt degraded and BCMs formed. In addition, during fermentation, the proteases associated with the cell wall of the starter culture or contaminating bacteria can hydrolyze caseins into large peptides and possibly even BCM7, which subsequently are transported into the bacteria cell and then hydrolyzed into a large number of smaller peptides, e.g., di and tri-peptides (Nielsen et al., Citation2009). Consequently, L. delbrueckii ssp. bulgaricus and S. thermophilus used for producing yoghurt may digest β-casein into BCM7 and other related BCMs; however, these may be subsequently degraded into smaller peptides.
The stability of BCMs in yoghurt may be influenced by the symbiotic growth of L. delbrueckii ssp. bulgaricus and S. thermophilus. It is well known that the growth of L. delbrueckii ssp. bulgaricus and S. thermophilus is strongly related, because products released by each organism stimulate the growth of the other (Tamime and Robinson, Citation1999). For instance, some peptides and amino acid formed by L. delbrueckii ssp. bulgaricus stimulate the growth of S. thermophilus (Courtin et al., Citation2002). Therefore, BCMs may be released by L. delbrueckii ssp. bulgaricus protease activity and subsequently degraded by S. thermophilus, and vice versa. Nevertheless, Gobbetti et al. (Citation2000) have demonstrated that only the strain of L. delbrueckii ssp. bulgaricus SS1, when used for fermentation of yoghurt, produced several fractions originating from β-CN such as β-CN f6–14, β-CN f7–14, β-CN f73–82, β-CN f74–82, and β-CN f75–82 but no BCMs. Similarly, Papadimitriou et al. (Citation2007) identified β-CN f176–180 and β-CN f1–8 in sheep yoghurt inoculated with only L. delbrueckii ssp. bulgaricus ϒ 10.13. It is unknown when and if BCMs are released or degraded in yoghurt processing as using starter cultures L. delbrueckii ssp. bulgaricus and S. thermophilus but this needs further investigation.
IMPACT OF DAIRY PROCESSING ON FORMATION AND DEGRADATION OF BETA-CASOMORPHINS
Effect of Thermal Treatment
Thermal treatment is an important step in dairy processes from a food safety and quality point of view. In addition to killing both spoilage and pathogenic bacteria, thermal treatments result in the modification of the milk protein conformation that beneficially affects the texture and viscosity of fermented dairy products (Krasaekoopt, Citation2003; Hattem et al., Citation2011). In yoghurt production, secondary heating in addition to pasteurization is commonly performed at 90–95°C for five minutes (EFSA, Citation2009) causing denaturation of whey proteins, and promotion of the interaction between caseins and whey proteins; particularly κ-casein and β-lactoglobulin. The formation of this complex influences proteolysis during fermentation (El-Zahar et al., Citation2003). Furthermore, heat treatments contribute to the breakdown of parent proteins to form lower molecular weight peptides (Gaucheron et al., Citation1999).
Formation of peptides from caseins is not only by enzymatic (Lotfi, Citation2004; Meltretter et al., Citation2008) but also through fragmentation of the protein backbone during heat treatment of milk (Meltretter et al., Citation2008). Meltretter et al. (Citation2008) identified five new peptides not present in the raw milk, after it was heated at 120°C for 30 minutes. Unknown peptides were identified by matrix-assisted laser desorption/ionization time of flight mass spectrometry (MALDI-TOF-MS) at mass-to-charge (m/z) ratios 974.4, 2218.7, 3730.1, 4297.8, and 4436.8 and they were reported to have originated from caseins. None of the m/z values matched those of BCMs indicating that no BCMs were formed by simply heating raw milk. During heat treatment of milk at 120°C for 30 minutes, enzymes are inactivated and the pH of the milk is unlikely to change greatly, therefore the formation of peptide by enzyme and acid hydrolysis can be excluded. On the other hand, during thermal treatment of milk the Maillard reaction, which is a reaction between a reducing sugar (lactose) with amino acids, may form radicals that can attack the peptide bonds within the protein to cleave the bonds and yield new peptides (Meltretter et al., Citation2008).
The formation of new peptides during heat treatment of milk will depend on the thermal strength of peptide bonds of β-casein. Gaucheron et al. (Citation1999) reported the formation of low molecular weight peptides in raw skim milk heated at 120°C for 30 minutes. Ten peptides, not present in raw milk, were identified; two of which were liberated from β-casein, including β-CN f1–14 and β-CN f1–16 but neither BCMs nor precursors of BCMs were found. According to the authors, peptides released after heat treatment are mainly a result of the hydrolysis of peptide bonds involving either aspartic acid (Asp), asparagine (Asn), or glutamic acid (Glu) bound to any other amino acid; bonds known to be more susceptible to hydrolysis during heat treatment (Gaucheron et al., Citation1999). Gaucher et al. (Citation2008) found Asn containing peptide bonds were also susceptible to thermally induced breakdown. Gaucher et al. (Citation2008) identify several pro-BCMs in UHT milk stored for six months at 20°C, namely; β-CN f54–68, β-CN f54–69, β-CN f55–65, β-CN f55–68, and β-CN f57–68 derived from the action of the enzymes elastase and cathepsins. Peptides, β-CN f54–68, β-CN f55–68, and β-CN f57–68, resulted from hydrolysis of a peptide bond involving Asn. Therefore, it appears that peptide bonds containing Asn are more susceptible to hydrolysis than those not containing this amino acid residue during heat treatment of milk. Schieber and Brückner (Citation2000) also found β-CN f57–68 in yoghurt which may have been a result of milk heat treatment which is a common processing step in yogurt production.
Recently, the formation and stability of BCM7 in heated milk has attracted attention of researchers (De Noni and Cattaneo Citation2010; Cieślińska et al. Citation2012). De Noni and Cattaneo (Citation2010) were the first to measure BCM7 content in commercial pasteurized, UHT, and in-bottle heat-treated milk. The authors showed that BCM7 was not detected in these products, even though the products contained A1 and B β-casein variants which contain the BCM7 sequence. Similarly, Cieślińska et al. (Citation2012) quantified the content of BCM7 by ELISA in varieties of milk containing pure A1, A2 and mixture of A1 and A2 β-casein variants after pasteurization (95°C/20 minutes) and sterilization (117°C/5 minutes). BCM7 content tended to decrease after heating of the milk but not due to the breaking down the peptide bonds of BCM7 but because of the reaction between lactose and amino acids residues of BCM7, which reduced the binding affinity of the modified BCM7 to the antibody used in the ELISA assay, leading to underestimated results for BCM7 levels (Cieślińska et al., Citation2012). Thus, thermal treatment may modify the β-casein and influence formation of BCM7 in further processing of dairy products.
The previous thermal treatment history of the milk plays a crucial role in proteolysis during subsequent fermentation and aging of fermented dairy products (El-Zahar et al., Citation2004). Hayaloglu et al. (Citation2010) investigated the peptide profile of Malatya cheese made from raw milk and from milk pasteurized at 72°C for 30 seconds. The authors suggested that pasteurization of milk results in a decrease in peptide content during ripening, with considerably higher concentration of peptides being reported in cheese made from raw milk than from pasteurized milk; however, the actual peptides produced were not identified. The release of peptides may be due primarily to proteolysis by nonstarter bacteria-derived protease activity in the raw milk as pasteurization of milk at 72°C for 30 seconds inactivated nonstarter culture bacteria. In contrast, Mendia et al. (Citation2000) reported that the level of proteolysis, mainly of β-casein, was higher in cheese made from pasteurized ewe milk than that made from raw milk.. The action of plasmin which is more active in milk that has been pasteurized than in unheated milk may be the reason for the contrast in results (Mendia et al., Citation2000; Benfeldt and Sørensen, Citation2001), although plasmin will be inactivated upon severe heat treatment of milk. Although a number of studies have investigated the effect of thermal treatment of milk on proteolysis (Rynne et al., Citation2004; Hayaloglu et al., Citation2010; Hougaard et al., Citation2010), its effect on modification of protein, leading to formation of BCMs in the final fermented dairy product is still unknown.
Effect of Fermentation Process
Fermentation is an extremely important step in the processing of milk into cheese and yoghurt. In addition to a drop in pH, the fermentation process causes a series of changes in chemical composition of milk resulting from proteolysis, glycolysis, and lipolysis. These reactions are dependent on the type of starter culture, incubation temperature and time, and maturation time.
The choice of starter culture is a crucial factor in determining the attributes of fermented dairy products. During fermentation, different strains of starter culture can release different bioactive peptides from milk protein, including BCMs liberated from β-caseins (). Some BCMs isolated from cheeses have been described in ; however, these studies did not characterize the starter cultures used for producing the cheeses. As previously described, the use of L. casei L26, L. acidophilus L10, and B. lactis B94, for producing probiotic yoghurt (Donkor et al., Citation2007) and L. delbrueckii ssp. bulgaricus and S. salivarius ssp. thermophilus for fermentation of traditional yoghurt (Schieber and Brückner, Citation2000; De Noni and Cattaneo, Citation2010) does not result in the formation of BCM7 and other related BCMs. According to Atlan et al. (Citation1990) and Meyer and Jordi (Citation1987), a mixture of starter cultures, L. delbrueckii ssp. bulgaricus and S. thermophilus used for traditional yoghurt making contains a high level of PepX that may degrade proline-rich BCMs into a large number of smaller peptides. Meyer and Jordi (Citation1987) reported that the pH optimum of PepX isolated from S. thermophilus was in the range of 6.5–8.2 and that this enzyme is inactivated at pH below 5.0. For PepX isolated L. delbrueckii ssp. bulgaricus optimal conditions were pH 7.0 and 50°C (Atlan et al., Citation1990). Yoghurt fermentation also involves a drop in pH from 6.8 to 6.3 during first stage of fermentation and if BCM7 is released at this stage, it may be degraded by PepX. Consequently, when BCM7 is formed or degraded in yoghurt inoculated with L. delbrueckii ssp. bulgaricus and S. thermophilus remains unknown.
Table 3 BCMs released by different bacteria
In addition to type of starter culture, the pH at which the fermentation stops is a key factor affecting proteolysis subsequent during cold storage. In fermented milk manufacture, fermentation commonly ends at pH 3.5–4.6 before subsequent cold storage. According to Nielsen et al. (Citation2009), milk inoculated with L. lactis strains and L. helviticus strains fermented to pH 4.6 and 4.3 resulted in large peptides being released from β-casein after 7-day cold storage, but no BCMs. The authors also observed no change in peptide profile during storage for milk inoculated with S. thermophilus fermented to pH 4.6 and 4.3. This study indicated that protease derived from S. thermophilus was not active during cold storage. In another study, Paul and Somkuti (Citation2009) showed that peptides were slightly degraded by S. thermophilus and moderately hydrolyzed by L. delbrueckii spp. bulgaricus at pH 4.5 indicating that any BCMs formed during yogurt fermentation might also be slightly degraded at pH 4.5 during storage. Currently, there is very little published research on the effect of pH, especially a pH of 3.5, on the release or degradation during cold storage of BCMs in yoghurt inoculated with L. delbrueckii ssp. bulgaricus and S. thermophilus.
Effect of Cross Linking
In dairy technology, it is well-known that heat treatment (85–90°C for five minutes) increases the viscosity of yoghurt due to the interaction between κ-casein and whey protein by di-sulphide bonding. Due to the lack of cysteine in β-casein, inter/intra-molecular disulphide bonds formation during heat treatment are not possible for this protein. However, modification of β-casein can be performed by enzymatic covalent cross-linking (Monogioudi et al., Citation2009) via tyrosine, lysine, and glutamine residues through the addition of tyrosinase and transglutaminase (TGase) (Monogioudi et al., Citation2009).
TGase affects the texture and proteolysis of yoghurt (Yuksel and Erdem, Citation2010; Sanli et al Citation2011). Yuksel and Erdem (Citation2010) reported that peptide content of yoghurt decreases when TGase is added to the milk, but there was no difference in the peptide profile between TGase-treated and untreated milk. Furthermore, peptide content in milk treated with TGase that was still active during fermentation was less than that in milk treated with Tgase that had been inactivated during fermentation. The formation of inter-molecular cross-linking during fermentation of yoghurt is related to the increase in lactic acid that leads to a decrease in stability of casein micelles (Yuksel and Erdem, Citation2010). As described in , BCMs contain tyrosine residues that have the potential to form enzyme-catalyzed cross-linking, therefore, treatment of milk with cross-linking enzymes may reduce the production of BCMs from β-casein.
METHODS FOR IDENTIFICATION AND QUANTIFICATION OF BETA-CASOMORPHINS
Milk and dairy products are complex matrices, in which proteins, lactose, and lipids can interfere with separation and detection of target peptides, leading to erroneous quantitative and qualitative results. Reversed-phase high performance liquid chromatography (RP-HPLC) has been used widely in separation of peptides and amino acids. For example, RP-HPLC-UV (Muehlenkamp and Warthesen, Citation1996) and ion-exchange chromatography (Jarmolowska et al., Citation1999) have been used for the separation and quantification of BCM7 present in cheese while HPLC-UV has been also used for determining BCM7 content in human milk (Jarmołowska et al., Citation2007). A limitation of RP-HPLC-UV is that peptides with similar physico-chemical and spectrophotometric absorption properties can co-elute with BCMs, increasing the absorption values (Muehlenkamp and Warthesen, Citation1996; Sienkiewicz-Szłapka et al., Citation2009), resulting in an overestimation of the BCM7 content (Cass et al., Citation2008). In addition, methods employing UV-Vis adsorption may lack the sensitivity required to quantify low levels of BCM7 found in some dairy products. The limit of quantification for BCM7 analyzed by RP-HPLC-UV is approximately 2 μg/mL of cheese extract making accurate quantification often not possible (Muehlenkamp and Warthesen, Citation1996). Despite these deficiencies, RP-HPLC-UV is a well-established, relatively cheap, and user-friendly analytical technique. These characteristics all contribute to make the technique very popular among others.
Currently, HPLC coupled with mass spectrometry (MS) represents “the-state-of-the-art” method for identification and quantification of peptides in complex matrices such as dairy product extracts. Different mass spectrometry techniques such as tandem mass spectrometry (MS/MS), quadrupole ion-trap mass spectrometry (QIT-MS), and time of flight mass spectrometry (TOF-MS) have been applied successfully to assess BCMs content in dairy products (). Each technique has its own and unique advantages. The selectivity and sensitivity of tandem mass spectrometry, especially when operated in multiple reaction monitoring (MRM) mode, allows accurate quantification of BCM5 and BCM7 at low levels (low μg/g) in the presence of multiple co-eluting interferences. For example, using LC-MS/MS, Haileselassie et al. (Citation1999) identified several potentially bioactive peptides, including BCM7, from an enzyme-modified Cheddar cheese (EMC) prepared using a neutral protease produced from B. subtilis. Using LC-MS, Rizzello et al. (Citation2005) analyzed the water-soluble extracts of an Italian goat cheese. BCM9 was identified in the extract. Formation of BCM7 following 10–60 minutes digestion of milk with pepsin at pH 2.0 and β-casein degradation have also been monitored using LC-MS (Schmelzer et al., 2007). More recently, applications of HPLC-MS/MS for quantifying BCM5 and BCM7 in dairy products (De Noni, 2008; De Noni and Cattaneo, Citation2010) have been also reported. By using HPLC-MS/MS, De Noni and Cattaneo (Citation2010) quantitatively determined BCM5 and BCM7 in cheese, milk, fermented milk, infant powder, and extracts of these products after in vitro simulated gastro-intestinal digestion. The authors showed that the level of BCM7 that could be detected was as low as 0.01 μg/g.
Table 4 Methods for analysis of BCMs
Quadrupole ion trap mass spectrometry features multiple tandem MS spectra (MSn), allowing, to some extent, structural elucidation of unknown peptides. In a similar fashion to tandem mass spectrometry, QIT-MS can be also used for quantitative assessment of BCM5 and BCM7 in single reaction monitoring (SRM) mode. For example Juan-Garcia et al. (Citation2009) examined BCMs in cheese and milk using a nanoelectrospray quadrupole ion-trap mass spectrometer providing a fast and easy means of analysis of these compounds in different matrices. The main fragmentation pathways for BCMs and structures for the major fragment ions that were recorded in the MSn spectra, were also reported.
Time of flight mass spectrometry (TOF-MS), being a high accuracy and high resolving power mass spectrometry technique, is of particular value for confirmation and when unknown compounds need to be unequivocally identified in complex matrices. This is usually achieved by combining elemental formula information (i.e. CxHyOzNj…) from high mass accuracy experiments with structural information from fragmentation experiments. For example, using HPLC TOF-MS, Toelstede et al. (2008) found BCM9 and BCM10 in the water-soluble extract of a matured Gouda cheese. The possibility of interfacing a matrix-assisted laser desorption ion source (MALDI) to a TOF-MS allowed Cass et al. (Citation2008) to analyze opioid-derived exogenous or endogenous peptides in urines of children with autism, and found that peaks previously analyzed by HPLC-UV were erroneously identified as opioid peptides. Hence the need of high resolution for identification and confirmation work. Righetti et al. (1997) confirmed the identity of opioid peptides by N-terminal sequencing followed by MALDI-TOF mass spectrometry.
One alternative method for BCMs determination is ELISA (Sienkiewicz-Szłapka et al., Citation2009; Cieślińska et al., Citation2012). Using ELISA, levels of BCM7 and BCM5 in cheese as low as 0.04 and 0.05 μg/g, respectively, could be accurately quantified. However, heat treatment of milk may modify the conformation of BCM7 by interaction between lactose and amino acid residues, leading to reduction in the binding affinity of the modified BCM7 to the antibody and underestimation of the BCM level (Cieślińska et al., Citation2012). This may be a reason why Cieślińska et al. (Citation2012) observed the decrease of BCM7 content when determined by ELISA during heat treatment of milk.
Thus, HPLC coupled to different ranges of mass spectrometry technologies is a suitable method for quantifying BCMs in complex dairy matrices at low level as well as for confirmation and identification of unknown peptides in the same matrices. The possibility to extend the application of HPLC-MS to determine BCMs content in biological fluids such as urine does exist (Cass et al., Citation2008) as this technique has been proven rather flexible, accommodating the analysis of a wide range of matrices.
CONCLUSION
BCM is a group of opioid peptides released from β-casein by proteolysis, among which BCM7 is a peptide released from β-casein variants containing histidine residue at position 67 (β-casein A1 and B variants). Consumption of milk containing variant A1 and B β-casein may increase the risk of human disease such as type 1 diabetes and heart disease but an important precursor to clinical studies is the an understanding of the conditions that cause BCM formation and degradation. BCMs may be formed in all fermented dairy products, cheese, and yoghurt, but may be degraded during processing so as not detectable in the product as consumed. Processing factors that might affect the formation and/or degradation of BCMs include heat treatment, fermentation, ripening, and cold storage. Due to the lack of knowledge on the presence of BCM7 and other related BCMs in fermented milks, especially yoghurt, it is now necessary to further study the effects of combinations of processing factors on the formation and/or degradation of BCM7 and other related BCMs in these popular products. Whether BCMs do form in yoghurt and the amount of BCM forming or degrading at different processing steps needs further investigation and possibly will depend on the heat treatment and fermentation process used, but it remains an intriguing unknown. The application of analytical methods employing tandem mass spectrometry or high resolution mass spectrometry is strongly recommended to confidently identify and accurately quantify BCMs in complex dairy product matrices.
REFERENCES
- Atlan, D., Laloi, P. and Portalier, R. (1990). X-prolyl-dipeptidyl aminopeptidase of lactobacillus delbrueckii subsp. bulgaricus: Characterization of the enzyme and isolation of deficient mutants. Appl. Environ. Microbiol. 56(7):2174–2179.
- Bell, S., Grochoski, G. and Clarke, A. (2006). Health implications of milk containing beta-casein with the A2 genetic variant. Crit. Rev. Food Sci. Nutr. 46(1):93–100.
- Benfeldt, C. and Sørensen, J. (2001). Heat treatment of cheese milk: Effect on proteolysis during cheese ripening. Int. Dairy J. 11(4–7):567–574.
- Birgisdottir, B. E., Hill, J. P., Thorsson, A. V. and Thorsdottir, I. (2006). Lower consumption of cow milk protein A1 beta-casein at 2 years of age, rather than consumption among 11- to 14-year-old adolescents, may explain the lower incidence of type 1 diabetes in Iceland than in Scandinavia. Ann. Nutr. Metabol. 50(3):177–183.
- Cass, H., Gringras, P., March, J., McKendrick, I., O’Hare, A. E., Owen, L. and Pollin, C. (2008). Absence of urinary opioid peptides in children with autism. Arch. Dis. Chil. 93(9):745–750.
- Choi, J., Sabikhi, L., Hassan, A. and Anand, S. (2012). Bioactive peptides in dairy products. Int. J. Dairy Technol. 65(1):1–12.
- Cieślińska, A., Kaminski, S., Kostyyra, E. and Sienkiewicz-Szłapka, E. (2007). Beta-casomorphin 7 in raw and hydrolyzed milk derived from cows of alternative β-casein genotypes. Milchwissenschaft 62(2):125–127.
- Cieślińska, A., Kostyra, E., Kostyra, H., Oleński, K., Fiedorowicz, E. and Kamiński, S. (2012). Milk from cows of different β-casein genotypes as a source of β-casomorphin-7. Int. J. Food Sci. Nutr. 63(4):426–430.
- Courtin, P., Monnet, V. and Rul, F. (2002). Cell-wall proteinases PrtS and PrtB have a different role in Streptococcus thermophilus/Lactobacillus bulgaricus mixed cultures in milk. Microbiology 148(11):3413–3421.
- De Noni, I. (2008). Release of [beta]-casomorphins 5 and 7 during simulated gastro-intestinal digestion of bovine [beta]-casein variants and milk-based infant formulas. Food Chemistry 110(4):897–903.
- Donkor, O. N., Henriksson, A., Singh, T. K., Vasiljevic, T. and Shah, N. P. (2007). ACE-inhibitory activity of probiotic yoghurt. Int. Dairy J. 17(11):1321–1331.
- EFSA. (2009). Review of the potential health impact of β-casomorphins and related peptides. EFSA Science Report 231:1–107, Available from http://www.efsa.europa.eu/en/efsajournal/pub/231r.htm
- Elliott, R. B., Harris, D. P., Hill, J. P., Bibby, N. J. and Wasmuth, H. E. (1999). Type I (insulin-dependent) diabetes mellitus and cow milk: Casein variant consumption. Diabetologia 42(3):292.
- Elwood, P. C. (2005). Milk and cardiovascular disease: A review of the epidemiological evidence. Aus. J. Dairy Technol. 60(1):58.
- Elwood, P. C., Pickering, J. E., Fehily, A. M., Hughes, J. and Ness, A. R. (2004a). Milk drinking, ischaemic heart disease and ischaemic stroke I. Evidence from the Caerphilly cohort. Eur. J. Clin. Nutr. 58(5):711–717.
- Elwood, P. C., Pickering, J. E., Hughes, J., Fehily, A. M. and Ness, A. R. (2004b). Milk drinking, ischaemic heart disease and ischaemic stroke II. Evidence from cohort studies. Eur. J. Clin. Nutr. 58(5):718–724.
- Elwood, P. C., Strain, J. J., Robson, P. J., Fehily, A. M., Hughes, J., Pickering, J. and Ness, A. (2005). Milk consumption, stroke, and heart attack risk: Evidence from the Caerphilly cohort of older men. [Research Support, Non-U.S. Gov’t]. J. Epidemiol. Commun. Health 59(6):502–505.
- El-Zahar, K., Chobert, J.-M., Dalgalarrondo, M., Sitohy, M. and HaertlÉ, T. (2004). Proteolysis of ewe's caseins and whey proteins during fermentation of yogurt and storage. Effect of the starter cultures used. J. Food Biochem. 28(4):319–335.
- El-Zahar, K., Chobert, J.-M., Sitohy, M., Dalgalarrondo, M. and Haertlé, T. (2003). Proteolytic degradation of ewe milk proteins during fermentation of yoghurts and storage. Food/Nahrung 47(3):199–206.
- Enright, E., Patricia Bland, A., Needs, E. C. and Kelly, A. L. (1999). Proteolysis and physicochemical changes in milk on storage as affected by UHT treatment, plasmin activity and KIO3 addition. Int. Dairy J. 9(9):581–591.
- Gaucher, I., Mollé, D., Gagnaire, V. and Gaucheron, F. (2008). Effects of storage temperature on physico-chemical characteristics of semi-skimmed UHT milk. Food Hydrocol. 22(1):130–143. doi: 10.1016/j.foodhyd.2007.04.007
- Gaucheron, F., Mollé, D., Briard, V. and Léonil, J. (1999). Identification of low molar mass peptides released during sterilization of milk. Int. Dairy J. 9(8):515–521.
- Ginn, R. E., Packard, V. S., Mochrie, J. R. D., Kelley, W. N. and Schultz, L. H. (1985). Methods to detect abnormal milk. In: Standard Methods for the Examination of Dairy Products (15 ed.), G. H. Richardson, Ed. Taylor & Francis, Washington, D.C.
- Gobbetti, M., Ferranti, P., Smacchi, E., Goffredi, F. and Addeo, F. (2000). Production of angiotensin-I-converting-enzyme-inhibitory peptides in fermented milks started by lactobacillus delbrueckiisubsp. bulgaricus SS1 and Lactococcus lactissubsp. cremoris FT4. Appl. Environ. Microbiology 66(9):3898–3904.
- Gobbetti, M., Stepaniak, L., De Angelis, M., Corsetti, A. and Di Cagno, R. (2002). Latent bioactive peptides in milk proteins: Proteolytic activation and significance in dairy processing. Critical Reviews in Food Science and Nutrition 42:223–239.
- Gómez-Ruiz, J. Á., Ramos, M. and Recio, I. (2004). Identification and formation of angiotensin-converting enzyme-inhibitory peptides in Manchego cheese by high-performance liquid chromatography—tandem mass spectrometry. J. Chromatogr. A 1054(1–2):269–277.
- Haileselassie, S. S., Lee, B. H. and Gibbs, B. F. (1999). Purification and identification of potentially bioactive peptides from enzyme-modified cheese. J. Dairy Sci. 82(8):1612–1617.
- Hattem, H. E., Manal, A. N., Hanaa, S. S. and Elham, H. A. (2011). A study on the effect of thermal treatment on composition and some properties of camel milk. J. Brew. Distill. 2(4):51–55.
- Hayaloglu, A., Deegan, K. and McSweeney, P. (2010). Effect of milk pasteurization and curd scalding temperature on proteolysis in Malatya, a Halloumi-type cheese. Dairy Sci. Technol. 90(1):99–109.
- Hougaard, A. B., Ardö, Y. and Ipsen, R. H. (2010). Cheese made from instant infusion pasteurized milk: Rennet coagulation, cheese composition, texture and ripening. Int. Dairy J. 20(7):449–458.
- Jaffiol, C. (2008). Milk and dairy products in the prevention and therapy of obesity, type 2 diabetes and metabolic syndrome. Bull. de l’Académie nationale de médecine 192(4):749–758.
- Jarmołowska, B., Sidor, K., Iwan, M., Bielikowicz, K., Kaczmarski, M., Kostyra, E. and Kostyra, H. (2007). Changes of β-casomorphin content in human milk during lactation. Peptides 28(10):1982–1986.
- Jarmolowska, B., Kostyra, E., Krawczuk, S. and Kostyra, H. (1999). Beta-casomorphin-7 isolated from Brie cheese. J. Sci. Food Agr. 79(13):1788.
- Jauhiainen, T. and Korpela, R. (2007). Milk peptides and blood pressure. J. Nutr. 137(3):825S–829S.
- Jinsmaa, Y. and Yoshikawa, M. (1999). Enzymatic release of neocasomorphin and [beta]-casomorphin from bovine [beta]-casein. Peptides 20(8):957–962.
- Juan-García, A., Font, G., Juan, C. and Picó, Y. (2009). Nanoelectrospray with ion-trap mass spectrometry for the determination of beta-casomorphins in derived milk products. Talanta 80(1):294–306.
- Kahala, M., Pahkala, E. and Pihlato, A. (1993). Peptides in fermented Finnish milk products. Agr. Food Sci. Finland 2:379–386.
- Kamiński, S., Cieślińska, A. and Kostyra, E. (2007). Polymorphism of bovine beta-casein and its potential effect on human health. J. Appl. Genet. 48(3):189–198.
- Koch, G., Wiedemann, K., Drebes, E., Zimmermann, W., Link, G. and Teschemacher, H. (1988). Human β-casomorphin-8 immunoreactive material in the plasma of women during pregnancy and after delivery. Regulat. Peptides 20(2):107–117.
- Krasaekoopt, W. (2003). Yogurt from UHT milk: A review. Aust. J. Dairy Technol. 58(1):26.
- Kuchroo, C. N. and Fox, P. F. (1982). Soluble nitrogen in Cheddar cheese: Comparison of extraction procedures. Milchwissenschaft 37(6):331–335.
- Kunda, P. B., Benavente, F., Catalá-Clariana, S., Giménez, E., Barbosa, J. and Sanz-Nebot, V. (2012). Identification of bioactive peptides in a functional yogurt by micro liquid chromatography time-of-flight mass spectrometry assisted by retention time prediction. J. Chromatogr. A 1229(0):121–128.
- Laugesen, M. and Elliott, R. (2003). Ischaemic heart disease, Type 1 diabetes, and cow milk A1 b-casein. NZ Med. J. 116(1168):1–19.
- Lefier, D., Lamprell, H. and Mazerolles, G. (2000). Evolution of Lactococcus strains during ripening in Brie cheese using Fourier transform infrared spectroscopy. Lait 80(2):247–254.
- Lotfi, B. (2004). Optimization study for the production of an opioid-like preparation from bovine casein by mild acidic hydrolysis. Int. Dairy J. 14(6):535–539.
- Matar, C. and Goulet, J. (1996). β-casomorphin 4 from milk fermented by a mutant of Lactobacillus helveticus. Int. Dairy J. 6(4):383–397.
- McLachlan, C. N. S. (2001). [beta]-casein A1, ischaemic heart disease mortality, and other illnesses. Med. Hypotheses 56(2):262–272.
- McSweeney, P. L. H. (2004). Biochemistry of cheese ripening. Int. J. Dairy Technol. 57(2–3):127–144.
- Meltretter, J., Schmidt, A., Humeny, A., Becker, C. and Pischetsrieder, M. (2008). Analysis of the peptide profile of milk and its changes during thermal treatment and storage. J. Agr. Food Chem. 56(9):2899.
- Mendia, C., Ibañez, F. J., Torre, P. and Barcina, Y. (2000). Effect of pasteurization and use of a native starter culture on proteolysis in a ewes’ milk cheese. Food Control 11(3):195–200.
- Merriman, T. R. (2009). Type 1 diabetes, the A1 milk hypothesis and vitamin D deficiency. Diabetes Res. Clin. Practice 83(2):149–156.
- Messens, W., Estepar-Garcia, J., Dewettinck, K. and Huyghebaert, A. (1999). Proteolysis of high-pressure-treated Gouda cheese. Int. Dairy J. 9(11):775–782.
- Meyer, J. and Jordi, R. (1987). Purification and characterization of X-prolyl-dipeptidyl-aminopeptidase from Lactobacillus lactis and from Streptococcus thermophilus. J. Dairy Sci. 70(4):738–745.
- Minervini, F., Algaron, F., Rizzello, C. G., Fox, P. F., Monnet, V. and Gobbetti, M. (2003). Angiotensin I-converting-enzyme-inhibitory and antibacterial peptides from lactobacillus helveticus PR4 proteinase-hydrolyzed caseins of milk from six species. Appl. Environ. Microbiol. 69(9):5297–5305.
- Monogioudi, E., Creusot, N., Kruus, K., Gruppen, H., Buchert, J. and Mattinen, M.-L. (2009). Cross-linking of β-casein by Trichoderma reesei tyrosinase and Streptoverticillium mobaraense transglutaminase followed by SEC–MALLS. Food Hydrocoll. 23(7):2008–2015.
- Muehlenkamp, M. R. and Warthesen, J. J. (1996). [beta]-Casomorphins: Analysis in cheese and susceptibility to proteolytic enzymes from Lactococcus lactis ssp. cremoris. J. Dairy Sci. 79(1):20–26.
- Muguerza, B., Ramos, M., Sánchez, E., Manso, M. A., Miguel, M., Aleixandre, A., … and Recio, I. (2006). Antihypertensive activity of milk fermented by Enterococcus faecalis strains isolated from raw milk. Int. Dairy J. 16(1):61–69.
- Nagpal, R., Behare, P. V., Kumar, M., Mohania, D., Yadav, M., Jain, S., Menon, S., Parkash, F., Marotta, E., Minelli, C., Henry, J. K. and Yadav, H. (2011). Milk, milk products, and disease free health: An updated overview. Crit. Rev. Food Sci. Nutr. 52(4):321–333.
- Napoli, A., Aiello, D., Di Donna, L., Prendushi, H. and Sindona, G. (2007). Exploitation of endogenous protease activity in raw mastitic milk by MALDI-TOF/TOF. Anal. Chem. 79(15):5941–5948.
- Nielsen, M. S., Martinussen, T., Flambard, B., Sørensen, K. I. and Otte, J. (2009). Peptide profiles and angiotensin-I-converting enzyme inhibitory activity of fermented milk products: Effect of bacterial strain, fermentation pH, and storage time. Int. Dairy J. 19(3):155-
- De Noni, I. and Cattaneo, S. (2010). Occurrence of [beta]-casomorphins 5 and 7 in commercial dairy products and in their digests following in vitro simulated gastro-intestinal digestion. Food Chem. 119(2):560–566.
- Papadimitriou, C. G., Vafopoulou-Mastrojiannaki, A., Silva, S. V., Gomes, A.-M., Malcata, F. X. and Alichanidis, E. (2007). Identification of peptides in traditional and probiotic sheep milk yoghurt with angiotensin I-converting enzyme (ACE)-inhibitory activity. Food Chem. 105(2):647–656.
- Parrot, S., Degraeve, P., Curia, C. and Martial-Gros, A. (2003). In vitro study on digestion of peptides in Emmental cheese: Analytical evaluation and influence on angiotensin I converting enzyme inhibitory peptides. Die Nahrung 47(2):87–94.
- Paul, M. and Somkuti, G. A. (2009). Degradation of milk-based bioactive peptides by yogurt fermentation bacteria*. Lett. Appl. Microbiol. 49(3):345–350.
- Righetti, P. G., Nembri, F., Bossi, A., & Mortarino, M. (1997). Continuous Enzymatic Hydrolysis of β-Casein and Isoelectric Collection of Some of the Biologically Active Peptides in an Electric Field. Biotechnology Progress 13(3):258–264.
- Rizzello, C. G., Losito, I., Gobbetti, M., Carbonara, T., De Bari, M. D. and Zambonin, P. G. (2005). Antibacterial activities of peptides from the water-soluble extracts of Italian cheese varieties. J. Dairy Sci. 88(7):2348–2360.
- Robinson, R. K. (1995). A Colour Guide to Cheese and Fermented Milks. Taylor & Francis, London, UK.
- Rynne, N. M., Beresford, T. P., Kelly, A. L. and Guinee, T. P. (2004). Effect of milk pasteurization temperature and in situ whey protein denaturation on the composition, texture and heat-induced functionality of half-fat Cheddar cheese. Int. Dairy J. 14(11):989–1001.
- Sabeena Farvin, K. H., Baron, C. P., Nielsen, N. S., Otte, J. and Jacobsen, C. (2010). Antioxidant activity of yoghurt peptides: Part 2—Characterisation of peptide fractions. Food Chem. 123(4):1090–1097.
- Saito, T., Nakamura, T., Kitazawa, H., Kawai, Y. and Itoh, T. (2000). Isolation and structural analysis of antihypertensive peptides that exist naturally in gouda cheese. J. Dairy Sci. 83(7):1434–1440.
- Sanli, T., Sezgin, E., Deveci, O., Senel, E. and Benli, M. (2011). Effect of using transglutaminase on physical, chemical and sensory properties of set-type yoghurt. Food Hydrocoll. 25(6):1477–1481.
- Schieber, A. and Brückner, H. (2000). Characterization of oligo- and polypeptides isolated from yoghurt. Eur. Food Res. Technol. 210(5):310–313.
- Schmelzer, C. E., Schöps, R., Reynell, L., Ulbrich-Hofmann, R., Neubert, R. H., & Raith, K. (2007). Peptic digestion of β-casein: Time course and fate of possible bioactive peptides. Journal of Chromatography A 1166(1):108–115.
- Shimazaki, Y., Shirota, T., Uchida, K., Yonemoto, K., Kiyohara, Y., Iida, M., . . Yamashita, Y. (2008). Intake of dairy products and periodontal disease: The Hisayama study. J. Periodontol. 79(1):131–137.
- Sienkiewicz-Szłapka, E., Jarmołowska, B., Krawczuk, S., Kostyra, E., Kostyra, H. and Iwan, M. (2009). Contents of agonistic and antagonistic opioid peptides in different cheese varieties. Int. Dairy J. 19(4):258–263.
- Singh, T. K., Fox, P. F. and Healy, A. (1997). Isolation and identification of further peptides in the diafiltration retentate of the water-soluble fraction of Cheddar cheese. J. Dairy Res. 64(03):433–443.
- Smacchi, E. and Gobbetti, M. (1998). Peptides from several Italian cheeses inhibitory to proteolytic enzymes of lactic acid bacteria, pseudomonas fluorescens ATCC 948 and to the angiotensin I-converting enzyme. Enzyme Microbial Technol. 22(8):687–694.
- Stepaniak, L., Fox, P. F., Sorhaug, T. and Grabska, J. (1995). Effect of peptides from the sequence 58–72 of .beta.-Casein on the activity of endopeptidase, aminopeptidase, and X-prolyl-dipeptidyl aminopeptidase from Lactococcus lactis ssp. lactis MG1363. J. Agr. Food Chem. 43(3):849–853.
- Swaigood, H. E. (2003). Chemistry of the caseins. In: Advanced Dairy Chemistry (3 ed., Vol. 1: Proteins, pp. 165), P. F. Fox and P. L. H. McSweene, Eds., Taylor & Francis, NY.
- Tailford, K. A., Berry, C. L., Thomas, A. C. and Campbell, J. H. (2003). A casein variant in cow's milk is atherogenic. Atherosclerosis 170(1):13–19.
- Tamime, A. Y. and Robinson, R. K. (1999). Yoghurt: Science and Technology. Taylor & Francis, Cambridge, England.
- Toelstede, S. and Hofmann, T. (2008). Sensomics mapping and identification of the key bitter metabolites in gouda cheese. J. Agr. Food Chem. 56(8):2795–2804.
- Truswell, A. S. (2005). The A2 milk case: A critical review. Eur. J. Clin. Nutr. 59(5):623–631.
- Uenishi, K. (2006). Prevention of osteoporosis by foods and dietary supplements. Prevention of osteoporosis by milk and dairy products. Clin. Calcium 16(10):1606–1614.
- Woodford, K. (2009). Devil in the Milk: Illness, Health, and the Politics of A1 and A2 Milk. Chelsea Green, Taylor & Francis, Vermont.
- Yuksel, Z. and Erdem, Y. (2010). The influence of transglutaminase treatment on functional properties of set yoghurt. Int. J. Dairy Technol. 63(1):86–96.