Abstract
Inhibition of α-glucosidase and α-amylase delays the digestion of starch and disaccharides to absorbable monosaccharides, resulting in a reduction of postprandial hyperglycemia. Finding effective mammalian α-glucosidase inhibitors from natural sources can be beneficial in the prevention and treatment of diabetes mellitus. We investigated the inhibitory activity of cinnamic acid derivatives against rat intestinal α-glucosidase and porcine pancreatic α-amylase in vitro. Among 11 cinnamic acid derivatives, caffeic acid, ferulic acid, and isoferulic acid were the most potent inhibitors against intestinal maltase with IC50 values of 0.74 ± 0.01, 0.79 ± 0.04, and 0.76 ± 0.03 mM, respectively, whereas ferulic acid (IC50 = 0.45 ± 0.01 mM) and isoferulic acid (IC50 = 0.45 ± 0.01 mM) were effective intestinal sucrase inhibitors. However, all cinnamic acid derivatives were found to be inactive in pancreatic α-amylase inhibition. Kinetic analysis revealed that intestinal maltase was inhibited by caffeic acid, ferulic acid, and isoferulic acid in a mixed-inhibition manner. In addition, ferulic acid and isoferulic acid inhibited intestinal sucrase in a mixed type manner, whereas caffeic acid was a non-competitive inhibitor. The combination of isoferulic acid and acarbose showed an additive inhibition on intestinal sucrase. This study could provide a new insight into naturally occurring intestinal α-glucosidase inhibitors that could be useful for treatment of diabetes and its complications.
Introduction
Diabetes mellitus is in the group of metabolic diseases characterized by hyperglycemia, dyslipidemia, and protein metabolism that result from defects in both insulin secretion and/or insulin actionCitation1. The disease is associated with a reduced quality of life and increased risk factors for mortality and morbidity. Inhibition of α-glucosidase and pancreatic α-amylase is one of the therapeutic approaches for delaying oligosaccharide digestion to absorbable monosaccharides, resulting in reduced postprandial hyperglycemiaCitation2. Inhibitors of these enzymes also have a potential to reduce the progression of diabetes, as well as micro- and macrovascular complications such as retinopathy, nephropathy, diabetic neuropathy, and sexual dysfunctionCitation3.
An intake of plant foods and their ingredients could be a more effective strategy for the management of diabetes mellitus, because this is largely free from side effects. Many studies have focused on the potential biological activities or health effects of phytochemical compounds in animals and humans. In particular, cinnamic acid and its derivatives refer to one of the numerous and widely distributed groups of molecules in the plant kingdom. Interestingly, the focus has been on cinnamic acid derivatives regarding their potential benefits in antioxidativeCitation4, hepatoprotectiveCitation5, and antityrosinase relatedCitation6 as well as antidiabetic activitiesCitation7.
For example, ferulic acid (3-methoxy-4-hydroxycinnamic acid) is an effective scavenger of free radicals, prevents lipid peroxidation, and effectively suppresses the blood glucose level in diabetic miceCitation8. Furthermore, isoferulic acid significantly decreases the levels of plasma glucose concentration in streptozotocin (STZ)-induced diabetic rats by suppression of hepatic gluconeogenesis7. Our previous report has demonstrated the inhibitory activities of cinnamic acid derivatives against yeast α-glucosidaseCitation9. Currently, yeast α-glucosidase is known to be structurally different from those of mammalian origin. Accordingly, the study of α-glucosidase inhibition from mammalian sources may be a better model in the design and development of antihyperglycemic agentsCitation10.
Therefore, it would be interesting to investigate the structure–activity relationships of cinnamic acid and its derivatives with intestinal α-glucosidase (maltase and sucrase) and pancreatic α-amylase inhibition. The present work aimed to carry out a kinetic study of the inhibition of intestinal α-glucosidase by cinnamic acid derivatives in order to evaluate the types of inhibition. Finally, the combined effects of cinnamic acid derivatives and acarbose on α-glucosidase inhibition were investigated.
Materials and methods
Reagents
Rat intestinal acetone powder, porcine pancreatic α-amylase, and glucose oxidase kits were from Sigma-Aldrich (St. Louis, MO, USA). Acarbose was obtained from Bayer (Germany). Cinnamic acid, o-hydroxycinnamic acid, m- hydroxycinnamic acid, caffeic acid (3,4-dihydroxycinnamic acid), 3,4-dimethoxycinnamic acid, and p-hydroxycinnamic acid were from Fluka (St. Louis, MO, USA.). o-Methoxycinnamic acid, m-methoxycinnamic acid, and p-methoxycinnamic acid were obtained from ACROS (Pittsburgh, PA, USA). Ferulic acid (3-methoxy-4-hydroxycinnamic acid) and isoferulic acid (4-methoxy-3-hydroxycinnamic acid) were from Chromadex (Laguna Hills, CA, USA). All other chemicals used were of analytical grade.
Assay for intestinal α-glucosidase inhibitory activity
The assessment of intestinal α-glucosidase inhibitory activity was slightly modified, according to a previous methodCitation11. Briefly, 100 mg of rat intestinal acetone powder was homogenized in 3 mL of 0.9% NaCl solution. After centrifugation (12,000g × 30 min), the solution was subjected to assay, and the specific activities of sucrase and maltase were 0.20 and 0.68 units/mg protein, respectively. The crude enzyme solution (as maltase assay, 10 μL; as sucrase assay, 40 μL) was incubated with 70 μL maltose or 40 μL sucrose (final concentration: 25.9 mM maltose; 48 mM sucrose) in 0.1 M phosphate buffer, pH 6.9, and 20 μL of test compound at various concentrations in dimethyl sulfoxide (DMSO) at 37°C for 30 min (maltase assay) or 60 min (sucrase assay). The mixtures were suspended in boiling water for 10 min to stop the reaction. The concentrations of glucose released from the reaction mixtures were determined by the glucose oxidase method.
Assay for porcine α-amylase inhibitory activity
The pancreatic α-amylase inhibition assay was slightly modified, according to the literature procedureCitation12. Porcine pancreatic α-amylase was dissolved in 0.1 M phosphate buffered saline, pH 6.9. Various concentrations of test compound were added to a solution containing 1 g/L starch and phosphate buffer. The reaction was initiated by adding amylase (3 U/mL) to the incubation medium to a final volume of 500 μL. After 10 min the reaction was stopped by adding 0.5 mL dinitrosalicylic (DNS) reagent (1% 3,5-dinitrosalicylic acid, 0.2% phenol, 0.05% Na2SO3, and 1% NaOH in aqueous solution) to the reaction mixture. Mixtures were heated at 100°C for 10 min to develop a yellow-brown color. Thereafter, 500 μL of 40% potassium sodium tartrate solution was added to the mixture to stabilize the color. After cooling the mixtures to room temperature in a cold water bath, absorbance was recorded at 540 nm using a spectrophotometer.
Measurement of kinetic constant
In order to investigate the type of inhibition, enzyme kinetic analysis was performed according to the above reaction. Maintaining the quantity of sucrase constant at 0.20 units/mg protein or 0.68 units/mg protein, test compounds (0 and 0.25 mM) were measured for final concentrations of maltose (6.5, 12.8, 25.9, 51.5 mM) and sucrose (21.6, 32, 48, 64.8 mM). The type of inhibition was calculated on the basis of a Lineweaver–Burk plot using reciprocally plotted data (substrate concentration on horizontal axis and velocity on vertical axis)Citation13.
Combined effect of cinnamic acid derivatives and acarbose
Acarbose (1 μM) was combined with or used without the cinnamic acid derivatives (0.031 mM). The reaction was performed according to the above assay. Results are expressed as percentage inhibition compared to the corresponding control values.
Data analysis
Data are expressed as mean ± SEM (n = 3). IC50 values were determined from plots of log concentration of inhibitor versus percentage inhibition. The type of inhibition and constants were calculated on the basis of Lineweaver–Burk plots and non-linear regression of the replot of slopes using Sigma Plot 10.0 software (IL, USA). Statistical analysis was performed by Student’s t-test (p < 0.01).
Results
Screening of cinnamic acid derivatives as intestinal α-glucosidase and porcine α-amylase inhibitors
Eleven compounds of cinnamic acid and its derivatives () were investigated for intestinal α-glucosidase (maltase and sucrase) and α-amylase inhibition. The results in show the percentage enzyme inhibition of test agents at a concentration of 2 mM. Cinnamic acid (1) showed a weak inhibitory activity against intestinal maltase and sucrase. Upon the introduction of a hydroxyl group to cinnamic acid at various positions, it was found that the intestinal maltase and sucrase inhibitory activities increased in the order of 2 > 3 = 4. In addition, replacement of a hydroxyl residue in the cinnamic acid by a methoxyl residue (5–7) resulted in a significant loss of intestinal maltase and sucrase inhibitory activities. The addition of two hydroxyl substituents at meta and para positions in cinnamic acid (8) exhibited higher potency than that of the presence of two methoxyl substituents at the same positions (9). Interestingly, replacement of a hydroxyl residue at the meta or para position of 8 by a methoxyl moiety (10, 11) did not affect the inhibitory activity against intestinal maltase, but it increased the intestinal sucrase inhibitory activity. Likewise, all test compounds did not show any effect on α-amylase inhibition (data not shown).
Determination of IC50 values for intestinal maltase and sucrase activities
As mentioned above, 8, 10, and 11 were effective compounds against α-glucosidase inhibition. Thus, we further examined the IC50 values of these compounds against intestinal maltase and sucrase (). They exhibited selective inhibition of α-glucosidase toward sucrase rather than maltase. The IC50 values for intestinal maltase inhibition by 8, 10, and 11 were 0.74 ± 0.01, 0.79 ± 0.04, and 0.76 ± 0.03 mM, respectively. In addition, IC50 values for intestinal sucrase inhibition by 8, 10, and 11 were 0.49 ± 0.01, 0.45 ± 0.01, and 0.45 ± 0.01 mM, respectively. However, these compounds were less potent than acarbose on intestinal maltase (IC50 = 2.2 ± 0.6 μM) and sucrase inhibition (IC50 = 4.8 ± 1.2 μM).
Table 1. IC50 values for intestinal α-glucosidase (maltase and sucrase) inhibition by cinnamic acid derivatives.
Inhibition kinetic parameters of cinnamic acid derivatives on intestinal maltase and sucrase
To further explore the inhibitory characteristics of cinnamic acid derivatives, kinetic assay was performed using Lineweaver–Burk double reciprocal plots. The inhibition mechanisms on intestinal maltase by 8, 10, and 11 are shown in . Lineweaver–Burk plots for 8, 10, and 11 generated straight lines which had different intersections on the x-axis in the second quadrant, indicating that their inhibitions were of the mixed competitive and non-competitive type.
Figure 3. Lineweaver–Burk plots for inhibitory activity of (A) compound 8, (B) compound 10, and (C) compound 11 on intestinal maltase.
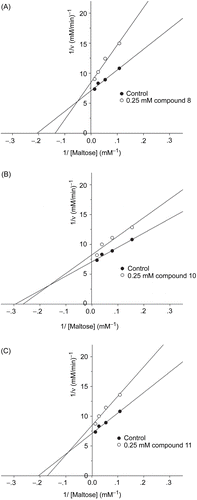
The inhibition mechanisms on intestinal sucrase by 8, 10, and 11 are shown in . Lineweaver–Burk plots for 10 and 11 generated straight lines which had a point of intersection in the second quadrant, suggesting that their inhibitions were of the mixed competitive and non-competitive type, whereas 8 generated straight lines which intersected on the x-axis, indicating that its type of inhibition was non-competitive.
Combined effect of cinnamic acid derivatives and acarbose
It was of interest to establish whether 8, 10, and 11 and acarbose might interact synergistically or additively on α-glucosidase. The assay was then performed using solutions containing acarbose alone or mixtures with a low dose of these compounds (0.03 mM). The effects of 8, 10, and 11 with acarbose on intestinal maltase and sucrase inhibition are shown in . Upon addition of 8, 10, and 11 to the assay system with acarbose (1 μM), the percentage sucrase inhibition was increased when compared with acarbose alone. The percentage inhibition using the mixtures was equal to the summing effect of acarbose and these compounds. These findings indicate that the combination of the compounds and acarbose produced additive inhibition against intestinal sucrase. However, there was no increase in percentage maltase inhibition when these compounds were combined with acarbose, indicating that 8, 10, and 11 did not produce any additive or synergistic inhibition with acarbose in this case.
Discussion
This is the first study to investigate the structure–activity relationships of cinnamic acid and its derivatives with regard to intestinal α-glucosidase inhibition (maltase and sucrase). In the present study, it was found that cinnamic acid is a weak intestinal α-glucosidase inhibitor. Our findings indicate that the presence of one substituent by a hydroxyl moiety is important for inducing potent inhibition against intestinal maltase and sucrase. Moreover, the findings also suggest that the presence of two hydroxyl groups at the meta and para positions of cinnamic acid can increase the potency of intestinal maltase inhibition. The conversion of one hydroxyl group at the meta or para position to a methoxyl group did not affect inhibitory activity on intestinal maltase; in contrast, it dramatically enhanced the potency of intestinal sucrase inhibition. The explanation for interaction phenomena between cinnamic acid derivatives and intestinal α-glucosidases is still unknown. It can be assumed that the compounds may form hydrogen bonds with the polar groups (amide, guanidine, peptide, amino, and carboxyl groups) of amino acid residues in the active site of intestinal α-glucosidase by covalent and/or non-covalent interaction. Further investigation is needed using X-ray crystallography and computer modeling to evaluate the binding activity of these compounds on the enzymes.
In general, α-glucosidases are classified into two families according to their substrate specificities. The first family consists of bacterial, yeast (Saccharomyces cerevisiae), and insect enzymes, named α-glucosidase I, which hydrolyze heterogeneous substrates or glucosyl structures, such as p-nitrophenyl α-glucosideCitation14,Citation15. Those from mold, plant, and mammalian tissues are called α-glucosidase II, and recognize maltooligosaccharides or maltosyl structuresCitation16. By analysis of the partial amino acid sequence containing the catalytic regions, the family I α-glucosidases are composed of four catalytic regions, whereas the family II α-glucosidases contain two catalytic regions responsible for the enzyme reactionCitation17. The difference in structure of the catalytic regions on yeast and mammalian α-glucosidases may directly affect the binding affinity of cinnamic acid derivatives. Our recent published data showed that the presence of hydroxyl residue in cinnamic acid gave less potent yeast α-glucosidase inhibition than that of methoxyl residue at the same positionCitation10. In addition, it has been found that p-methoxycinnamic acid is the most potent inhibitor among cinnamic acid derivatives against yeast α-glucosidase. However, the present study revealed that p-methoxycinnamic acid was inactive in intestinal α-glucosidase inhibition. Our findings support that the inhibitor of yeast α-glucosidase may not necessarily inhibit small-intestinal α-glucosidases.
Ferulic acid, a potent intestinal sucrase inhibitor, is commonly found in fruits and vegetables such as potatoCitation18, sweetcornCitation19, and rice branCitation20. The daily intake for a person who has a regular consumption of cereal products can be up to 100 mg of ferulic acidCitation21. Recent studies have reported that oral administration of ferulic acid (50 mg/kg) or the ethyl acetate fraction of rice bran (200 mg/kg) significantly decreases blood glucose levels and increases plasma insulin levels in type 2 diabetic mice by elevating hepatic glycogen synthesis and glucokinase activityCitation21. From phytochemical analysis, the ethyl acetate fraction of rice bran contains a large quantity of phenolic acids (526 mg%), of which ferulic acid is the most abundant (303 mg%). Moreover, it has been reported that oral administration of rice bran (20 g/day for an 8-week period) decreases the levels of glycosylated hemoglobin and fasting glucose and increases the serum insulin concentration in type 2 diabetic patientsCitation22. Isoferulic acid, an isomer of ferulic acid, is a main active ingredient of the rhizoma of Cimicifuga beracleifolia, which is used frequently in Japanese traditional medicine as an anti-inflammatory drug. It has been recently reported that isoferulic acid exhibits antihyperglycemic activity in streptozotocin (STZ)-induced diabetic ratsCitation7. It activates α1-adrenoceptors to enhance the secretion of β-endorphin, which stimulates the opioid μ-receptors to increase glucose use and/or reduce hepatic gluconeogenesis, resulting in a reduction of the plasma glucose concentrationCitation23. Our previous studies demonstrated that ferulic acid, but not isoferulic acid, directly stimulates insulin secretion from the pancreas, leading to decreased plasma glucose concentrations in ratsCitation24. Caffeic acid is a natural phenolic compound widely distributed in plant-derived materials such as tea, wine, and olive oil. It is also found in many types of fruit, and coffee, in high concentrations, and has exhibited pharmacological properties such as antioxidant, anticancer, and antimutagenic activitiesCitation25. On the basis of the caffeic acid content of brewed coffee measured after hydrolytic treatment, an intake of about 166 mg of caffeic acid can be calculated per cup of coffeeCitation26. Oral administration of caffeic acid decreases blood glucose and glycosylated hemoglobin levels in diabetic rats via its ability to enhance insulin secretion and to decrease hepatic glucose output along with the increased level of adipocyte glucose disposal in type 2 diabetic animalsCitation27. The abovementioned literature suggests that cinnamic acid derivatives act as insulin secretagogs and insulinomimetic agents. Considering the data obtained from this investigation, it is concluded that cinnamic acid derivatives contribute another mechanism in the control of hyperglycemia by inhibiting intestinal α-glucosidase, which contributes to the decrease of hemoglobin A1c (HbA1c). The decrease of HbA1c could reduce the incidence of chronic vascular complications in diabetic patientsCitation3.
The combination of acarbose and ferulic acid, isoferulic acid, and caffeic acid could produce more effective intestinal sucrase inhibition. This may lead to a reduction of postprandial hyperglycemia and hyperinsulinemia in type 2 diabetes. Further in vivo experiments should be carried out in order to investigate the effect of the combination of these compounds and acarbose on lowering blood glucose in type 2 diabetes. In addition, the most common adverse effect of acarbose is a gastrointestinal disturbance induced by gas production upon fermentation of unabsorbed carbohydrates in the bowelCitation28. Acarbose has rarely been associated with systemic adverse effects, but in some cases acute severe hepatotoxicity has been reportedCitation29. These adverse effects tend to increase with higher doses. Our findings suggest that the three compounds may enable a lower dose of acarbose to be used, resulting in diminishment of the adverse effect.
Although ferulic acid, isoferulic acid, and caffeic acid have been investigated regarding the possible mechanisms of antidiabetic activities, the present study shows that these compounds also inhibit intestinal α-glucosidase, which is one of the therapeutic approaches for treatment of diabetes mellitus. From this point of view, the intake of cinnamic acid derivative-enriched foods by diabetic patients may suppress postprandial hyperglycemia, helping in prevention of diabetic complications. Further comprehensive pharmacological investigations of these compounds are required to evaluate their toxicity and clinical efficacy for potential applications in the prevention and treatment of diabetes mellitus.
Acknowledgements
This research was financially supported by the Faculty of Allied Health Sciences, Chulalongkorn University.
Declaration of interest: The authors report no conflicts of interest.
References
- Altan VM. The pharmacology of diabetic complications. Curr Med Chem 2003;10:1317–27.
- Bischoff H. The mechanism of alpha-glucosidase inhibition in the management of diabetes. Clin Invest Med 1995;18:303–11.
- Baron AD. Postprandial hyperglycaemia and alpha-glucosidase inhibitors. Diabetes Res Clin Pract 1998;40(Suppl):S51–5.
- Natella F, Nardini M, Di Felice M, Scaccini C. Benzoic and cinnamic acid derivatives as antioxidants: structure-activity relation. J Agric Food Chem 1999;47:1453–9.
- Lee EJ, Kim SR, Kim J, Kim YC. Hepatoprotective phenylpropanoids from Scrophularia buergeriana roots against CCl4-induced toxicity: action mechanism and structure-activity relationship. Planta Med 2002;68:407–11.
- Lee HS. Tyrosinase inhibitors of Pulsatilla cernua root-derived materials. J Agric Food Chem 2002;50:1400–3.
- Liu IM, Hsu FL, Chen CF, Cheng JT. Antihyperglycemic action of isoferulic acid in streptozotocin-induced diabetic rats. Br J Pharmacol 2000;129:631–6.
- Ohnishi M, Matuo T, Tsuno T, Hosoda A, Nomura E, Taniguchi H, Sasaki H, Morishita H. Antioxidant activity and hypoglycemic effect of ferulic acid in STZ-induced diabetic mice and KK-Ay mice. Biofactors 2004;21(1–4):315–19.
- Adisakwattana S, Sookkongwaree K, Roengsumran S, Petsom A, Ngamrojnavanich N, Chavasiri W, Deesamer S, Yibchok-anun S. Structure-activity relationships of trans-cinnamic acid derivatives on alpha-glucosidase inhibition. Bioorg Med Chem Lett 2004;14:2893–6.
- Oki T, Matsui T, Osajima Y. Inhibitory effect of alpha-glucosidase inhibitors varies according to its origin. J Agric Food Chem 1999;47:550–3.
- Pluempanupat W, Adisakwattana S, Yibchok-Anun S, Chavasiri W. Synthesis of N-phenylphthalimide derivatives as alpha-glucosidase inhibitors. Arch Pharm Res 2007;30:1501–6.
- Kandra L, Zajácz A, Remenyik J, Gyémánt G. Kinetic investigation of a new inhibitor for human salivary alpha-amylase. Biochem Biophys Res Commun 2005;334:824–8.
- Adisakwattana S, Charoenlertkul P, Yibchok-Anun S. alpha-Glucosidase inhibitory activity of cyanidin-3-galactoside and synergistic effect with acarbose. J Enzyme Inhib Med Chem 2009;24:65–9.
- Matsusaka K, Chiba S, Shimomura T. Purification and substrate specificity of brewer’s yeast α-glucosidase. Agric Biol Chem 1977;41:1917–23.
- Ziak M, Meier M, Etter KS, Roth J. Two isoforms of trimming glucosidase II exist in mammalian tissues and cell lines but not in yeast and insect cells. Biochem Biophys Res Commun 2001;280:363–7.
- Kimura A. Molecular anatomy of α-glucosidase. Trends Glycosci Glycotechnol 2000;12:373–80.
- Okuyama M, Okuno A, Shimizu N, Mori H, Kimura A, Chiba S. Carboxyl group of residue Asp647 as possible proton donor in catalytic reaction of alpha-glucosidase from Schizosaccharomyces pombe. Eur J Biochem 2001;268:2270–80.
- Nara K, Miyoshi T, Honma T, Koga H. Antioxidative activity of bound- form phenolics in potato peel. Biosci Biotechnol Biochem 2006;70:1489–91.
- Dewanto V, Wu X, Liu RH. Processed sweet corn has higher antioxidant activity. J Agric Food Chem 2002;50:4959–64.
- Jung EH, Kim SR, Hwang IK, Ha TY. Hypoglycemic effects of a phenolic acid fraction of rice bran and ferulic acid in C57BL/KsJ-db/db mice. J Agric Food Chem 2007;55:9800–4.
- Kroon PA, Williamson G. Hydroxycinnamates in plants and food: current and future perspectives. J Sci Food Agric 1999;79:355–61.
- Qureshi AA, Sami SA, Khan FA. Effects of stabilized rice bran, its soluble and fiber fractions on blood glucose levels and serum lipid parameters in humans with diabetes mellitus types I and II. J Nutr Biochem 2002;13:175–87.
- Liu IM, Chen WC, Cheng JT. Mediation of beta-endorphin by isoferulic acid to lower plasma glucose in streptozotocin-induced diabetic rats. J Pharmacol Exp Ther 2003;307:1196–204.
- Adisakwattana S, Moonsan P, Yibchok-Anun S. Insulin-releasing properties of a series of cinnamic acid derivatives in vitro and in vivo. J Agric Food Chem 2008;56:7838–44.
- Okutan H, Ozcelik N, Yilmaz HR, Uz E. Effects of caffeic acid phenethyl ester on lipid peroxidation and antioxidant enzymes in diabetic rat heart. Clin Biochem 2005;38:191–6.
- Nardini M, Cirillo E, Natella F, Scaccini C. Absorption of phenolic acids in humans after coffee consumption. J Agric Food Chem 2002;50:5735–41.
- Jung UJ, Lee MK, Park YB, Jeon SM, Choi MS. Antihyperglycemic and antioxidant properties of caffeic acid in db/db mice. J Pharmacol Exp Ther 2006;318:476–83.
- Kelley DE, Bidot P, Freedman Z, Haag B, Podlecki D, Rendell M, Schimel D, Weiss S, Taylor T, Krol A, Magner J. Efficacy and safety of acarbose in insulin-treated patients with type 2 diabetes. Diabetes Care 1998;21:2056–61.
- Coniff R, Krol A. Acarbose: a review of US clinical experience. Clin Ther 1997;19:16–26.