Abstract
Coenzyme Q10 (CoQ10), also known as ubiquinone or ubidecarenone, is a powerful, endogenously produced, intracellularly existing lipophilic antioxidant. It combats reactive oxygen species (ROS) known to be responsible for a variety of human pathological conditions. Its target site is the inner mitochondrial membrane (IMM) of each cell. In case of deficiency and/or aging, CoQ10 oral supplementation is warranted. However, CoQ10 has low oral bioavailability due to its lipophilic nature, large molecular weight, regional differences in its gastrointestinal permeability and involvement of multitransporters. Intracellular delivery and mitochondrial target ability issues pose additional hurdles. To maximize CoQ10 delivery to its biopharmaceutical target, numerous approaches have been undertaken. The review summaries the current research on CoQ10 bioavailability and highlights the headways to obtain a satisfactory intracellular and targeted mitochondrial delivery. Unresolved questions and research gaps were identified to bring this promising natural product to the forefront of therapeutic agents for treatment of different pathologies.
Introduction
Coenzyme Q10 (CoQ10), also identified as ubiquinone or ubidecarenone, is a natural fat-soluble, vitamin-like, ubiquitously existing benzoquinone derivative (). It acts primarily as an antioxidant, a membrane stabilizer and a cofactor in the production of adenosine triphosphate (ATP) in the process well-known as oxidative phosphorylation (Littarru & Tiano, Citation2005). With such essential role, it steers cellular machinery and synthesis (Baker & Tarnopolsky, Citation2003). To the best of our knowledge, previous reviews on this wonder nutrient (∼97 reviews) mainly focused on the therapeutic uses (Kawasaki, Citation1992; Palazzoni et al., Citation1997; Soja & Mortensen, Citation1997; Al-Hasso, Citation2001; Gürkan & Bozdaǧ-Dündar, Citation2005; DiMauro et al., Citation2006; Frankovic, Citation2006; Pepe et al., Citation2007; Singh et al., Citation2007; Storch, Citation2007; Dos Santos et al., Citation2009; Spindler et al., Citation2009; Quinzii & Hirano, Citation2010; Janicki & Buzała, Citation2012; Cordero et al., Citation2013), formulations (Bhagavan & Chopra, Citation2007; Villalba et al., Citation2010; Bank et al., Citation2011; Žmitek et al., Citation2011; Barakat et al., Citation2013), clinical or preclinical trials (Mortensen et al., Citation1990; Hargreaves et al., Citation2005; Marcoff & Thompson, Citation2007; Littarru & Tiano, Citation2010), pharmacokinetics (Bhagavan & Chopra, Citation2007; Žmitek & Žmitek, Citation2009; Villalba et al., Citation2010; Žmitek et al., Citation2011) as well as deficiency and its consequences (Hargreaves, Citation2003; Naini et al., Citation2006; Land et al., Citation2007; Quinzii et al., Citation2007; Quinzii & Hirano, Citation2010; Trevisson et al., Citation2011). Nevertheless, oral absorption and targeting to mitochondria remain a major challenge for optimum antioxidant effect and hence need to be evaluated in light of the multiplicity of barriers that hinder the effective delivery of CoQ10 as a nutraceutical product. This review focus on compiling the various delivery strategies including those based on nanotechnology and mitochondrial targeting. Meanwhile, the review refutes published results on permeability and Biopharmaceutical Classification System (BCS) classification of CoQ10 and identifies research gaps and unanswered questions for future research work.
Therapeutic and clinical merits of CoQ10
CoQ10 is endogenously produced and mainly occurs intracellularly. Biosynthesis of CoQ10 is the highest in the metabolically active tissues such as the heart and immune system. The enzyme hydroxymethylglutaryl-CoA (HMG-CoA) reductase plays a critical role in the regulation of CoQ10 biosynthesis (Langsjoen & Langsjoen, Citation2003). Cholesterol-lowering drugs (statins) which inhibit this enzyme could induce a fall in CoQ10 (Langsjoen & Langsjoen, Citation2003). Based on several studies, patients taking statins are recommended to receive CoQ10 supplementation (Suzuki et al., Citation2008; Hamilton et al., Citation2009; Eussen et al., Citation2010; Wynn, Citation2010; Avis et al., Citation2011; Zlatohlavek et al., Citation2012; Littlefield et al., Citation2013). Exogenous CoQ10 can be produced either extraction from animal tissues, chemical synthesis or microorganism fermentation (Cheng et al., Citation2010). Human CoQ10 levels are modified with age and disease; the reduced CoQ10 (CoQ10H2; ubiquinol) or the ratio of reduced CoQ10 is more crucial than total CoQ10 (Tang et al., Citation2001) in reducing oxidative stress ().
There is disturbance in the pro-oxidant–antioxidant balance in support of the former results in oxidative stress. The ratio CoQ10 (reduced)/CoQ10 (total) is one of the most reliable markers of oxidative stress, as it is a direct product of redox imbalance (Yamashita & Yamamoto, Citation1997). It is thought to be low in individuals with various types of cancer, heart diseases, diabetes (Miyake et al., Citation1999) and neuromuscular disease (Tang et al., Citation2001). Particularly, reduced form (ubiquinol) increase as an adaptive response to oxidative stress (Yamamoto et al., Citation1998). Aged or diseased persons may have declined ubiquinol and in this case, oral intake would be valuable. Orally administered ubiquinone is converted to ubiquinol in the body by the reducing enzyme, NADPH (nicotinamide adenine dinucleotide phosphate reduced form) to act as an antioxidant. As a potent lipophilic antioxidant, ubiquinol is capable of recycling and regenerating other antioxidants such as tocopherol (vitamin E) and ascorbic acid (vitamin C) (Crane, Citation2001). Evidence-based studies have shown that CoQ10 has plentiful therapeutic and clinical benefits (). Studies reported that it has an anti-fatigue effect (Mizuno et al., Citation2008), ameliorates Huntington’s disease (Littarru & Tiano, Citation2007) atherosclerosis (Stocker & Keaney, Citation2004), some cases of cancer (Zmitek et al., Citation2008), gingivitis and periodontitis (Wilkinson et al., Citation1975, Gawish et al., Citation2011; Zaki, Citation2012), Parkinsonism (Ebadi et al., Citation2001), Alzheimer’s disease (Wadsworth et al., Citation2008), Friedreich’s ataxia (Cooper & Schapira, Citation2003), ischemia–reperfusion injury (Matejikova et al., Citation2008), diabetes (Eriksson et al., Citation1999), retinal damage (Nucci et al., Citation2007; Russo et al., Citation2008), chronic obstructive pulmonary disease (Fujimoto et al., Citation1993) and pulmonary fibrosis (Fujimoto et al., Citation1993). Additionally, it has an immune-stimulating action alone (Sugino et al., Citation1989) or when incorporated into liposomes and archaeosomes (Makabi-Panzu et al., Citation1998) as well as a gastro-protective effect in indomethacin-induced gastric ulcer (El-Abhar, Citation2010).
Pharmacokinetics of CoQ10
CoQ10 exhibits non-linear pharmacokinetics above a concentration 2400 mg/day (Shults et al., Citation2004). This behavior might be a consequence of a carrier-mediated absorption, saturation of transporter protein and/or existence of absorption window (as discussed in permeability barrier). On a single-dose basis, a dose-dependent increase in plasma CoQ10 levels was reported from 30 mg up to a 200 mg, resulting in a 6.1-fold increase in plasma levels (Kaikkonen et al., Citation2002). Divided dosages (2 × 100 mg) produce a larger increase in plasma levels than a single ingested 200 mg dose (Singh et al., Citation2005). Hence, larger daily doses are recommended to be divided into several doses not only to maximize the CoQ10 absorption, but also to reduce the difference between peaks and troughs steady states plasma levels. CoQ10, even at high dosages, has been safe in different studies (Liu & Artmann, Citation2009). A daily dosage up to 3600 mg was found to be tolerated by healthy as well as unhealthy persons (Hyson et al., Citation2010). However, the observed safe level (OSL) risk assessment method indicated that the evidence of safety is strong at intakes up to 1200 mg/day (Hathcock & Shao, Citation2006; Ikematsu et al., Citation2006; Liu & Artmann, Citation2009; Shults et al., Citation2002).
Barriers to oral and target-specific delivery of CoQ10
The chemical instability of CoQ10 in pharmaceutical preparations upon exposure to air, ultraviolet (UV) light or high temperature (Matsuda & Masahara, Citation1983) and the low oral bioavailability of ubiquinone hamper its adequate oral delivery (Bhagavan & Chopra, Citation2006). The underlying causes for the low bioavailability are the large molecular weight (863), high lipophilicity (log P = 21) (Persson et al., Citation2005) and poor aqueous solubility (0.7 ng/ml at 37 °C) (Persson et al., Citation2005) (). The slow dissolution rate of CoQ10 as a consequence of poor hydration sorts it as a grease ball compound. Although one study has categorized it as a Class II compound according to the BCS (Palamakula, Citation2004a; Wajda et al., Citation2007), this is questionable and contradicted by other published data will be discussed later. Regional variation in permeability across gastrointestinal tract (GIT) and reliance on multiple transporters also contribute to its low absorption (Palamakula et al., Citation2005). Cellular and sub-cellular pose challenges toward an effective targeted delivery of CoQ10 to its main site of action: the cell mitochondrion (Milagros Rocha & Victor, Citation2007). Various approaches were attempted to enhance the bioavailability and mitochondrial targeting of CoQ10 by surmounting the abovementioned barriers.
Figure 3. Physicochemical and physiological factors barriers against oral and targeted delivery of CoQ10.
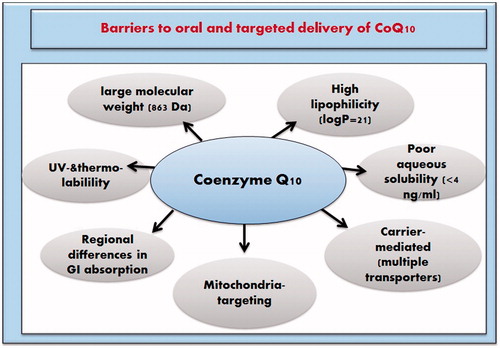
Strategies for surmounting stability barriers
CoQ10 in pharmaceutical products undergo appreciable degradation (Uekaji et al., Citation2010). The formulation strategies adopted to promote thermal and photostabilization CoQ10 include: nanoliposomes encapsulation (Xia et al., Citation2006), self-emulsifiable systems (Nazzal et al., Citation2002c) (using limonene rather than essential oils with a capsule formulation) (Palamakula et al., Citation2004), solid dispersion formation (Nazzal et al., Citation2002a), addition of ascorbic acid and chelation by EDTA (Kommuru et al., Citation1999) and cyclodextrin (CD) complexation (Prosek et al., Citation2008). Nanocarriers also increased the UV and temperature stability in case of CoQ10-loaded poly(methyl methacrylate) nanoparticles, in dispersion and oil-based formulations (Kwon, Citation2002). Recently, nanostructured lipid carriers (NLC) formulation prepared by high-pressure homogenization was able to stabilize CoQ10 in natural daylight for 1 and 5 months with 80 and 65% unchanged drug, respectively (Wang et al., Citation2012). It has been argued that bioavailability improvement of CoQ10 is hampered by the higher photodegradation in liquid formulations than in the solid ones (Matsuda & Masahara, Citation1983; Hatanaka et al., Citation2008). To address this issue, Hatanaka et al. (Citation2008) proposed dry emulsion formulations to circumvent the liquid formulations-associated instability. Solid self-emulsifying drug delivery systems (SEDDS) were presented (Tang et al., Citation2008). SEDDS powder containing CoQ10 in an amorphous state was developed by spray drying (Onoue et al., Citation2012). Solution, crystalline, nanoemulsified (NE) and s-SEDDS of CoQ10 were compared regarding their degradation kinetics and ranked in the following decreasing order: CoQ10 solution >>> CoQ10/NE > CoQ10/s-SEDDS > crystalline CoQ10 (Onoue et al., Citation2012). Hence, the dry emulsion and solid SEDDS were successful in overcoming degradation pre-existed in liquid formulations.
Strategies for surmounting solubility barriers
Size and lipophilicity are among the physicochemical properties that influence aqueous solubility and hence the dissolution rate. The tight water structure should open up a large enough cavity to accommodate the molecule. From a thermodynamic viewpoint the larger the cavity, the more the energy expenses required. Additionally, large size molecules are usually more lipophilic and hence interact poorly with water. CoQ10 exhibits an extremely low aqueous solubility (0.7 ng/ml) due to its large molecular weight (863) and highly (log P = 21) lipophilic nature (i.e. a grease ball nature). The formulation strategies for poorly soluble compounds are revised elsewhere (Kawabata et al., Citation2011; Zaki, 2014a). Many of these have been applied for formulation of oral CoQ10 as follows.
Particle size reduction (nanonization of pure CoQ10)
By adopting the supercritical solution process in the absence of any polymer carrier, a 1.96-fold increase in oral bioavailability of nanosized CoQ10 resulted over unprocessed CoQ10 powder in rats (Meng et al., Citation2012). The process was successful in obtaining a pure, nanosized, less crystalline structure with larger effective surface area and superior dissolution performance in contact with gastrointestinal fluids. Nanosuspensions of CoQ10 were also developed to improve the different physicochemical, pharmaceutical and dissolution-related properties using high-pressure homogenization at mild conditions in presence of Tween-80 and polyvinyl alcohol as stabilizers (Mauludin, Citation2009). Amorphization is a valid approach for solid state-limited solubility drugs that is with high melting point or the so-called “brick dust” nature (Wassvik et al., Citation2008; Mahlin & Bergstrom, Citation2013). Such an approach might not be beneficial for CoQ10 that has a low melting point (48 °C) and a “grease ball” nature (Zaki et al., Citation2010).
Solid dispersion
Solid dispersion (SD) of water-insoluble drugs in an inert hydrophilic carrier improves aqueous drug solubility by virtue of increased wettability, dispersibility and amorphous drug formation. A SD of CoQ10 and Eudragit L100-55 was prepared by the solvent-evaporation method and demonstrated enhanced solubility (100% in 12 h) over physical mixture (26.5% in 12 h) and pure drug (12.5% in 12 h) in a medium composed of 4% Labrasol and 2% Cremophor EL (Nazzal et al., Citation2002a). A scalable low temperature melting was used to form a binary SD of CoQ10 and poloxamer 188 (Bhandari et al., Citation2007). The Gibbs free energy was negative indicating a spontaneous dissolution. The solubility-enhancement effect increased as the concentration of poloxamer 188 increased despite that CoQ10 was not brought to the molecular level. Nepal et al. (Citation2010) formulated stable SD using poloxamer 407 as a polymeric carrier and surfactant whereas Aerosil® 200 imparted free flowing properties to the SD powder and acted as a recrystallization inhibitor and stabilizer. A US patent publication disclosed a SD composition using Kollidon® VA 64 as a stable polymeric carrier (Rosenberg & Breitenbach, Citation2004).
Emulsifiable systems
Microemulsion (ME) formulations as well as self-emulsifying or self-micro/nano emulsifying drug delivery systems (SEDDS, SMEDDS and SNEDDS) have gained increased awareness as a valuable tactic to improve the oral bioavailability of lipophilic drugs (Gursoy & Benita, Citation2004; Araya et al., Citation2005a,Citationb; Dokania & Joshi, Citation2014; Sharma et al., Citation2014; Singh & Pai, Citation2014). The biopharmaceutical merits of ME and SEDDs include faster drug release by virtue of the small droplet sizes and large interfacial area promoting pancreatic lipase hydrolysis of lipids and fast drug dissolution with/without bile salts-mixed micelles formation (Tarr & Yalkowsky, Citation1989). A remarkably improved bioavailability was observed in these formulations by different modus operandi including: (i) improved drug dissolution (Costantinides, Citation1985; Sharma et al., Citation2014), (ii) increased intestinal epithelial permeability (Swenson & Curatolo, Citation1992; Singh & Pai, Citation2014), (iii) increased tight junction permeability (Lindmark et al., Citation1995), (iv) hampered protein efflux (Eaimtrakarn et al., Citation2002) and (v) improved lymphatic absorption mediated by long-chain oils, with preferential access to the intestinal lymph than the blood (Porter & Charman, Citation1997; Caliph et al., Citation2000). On the other hand, digested lipids of medium-chain triglycerides with bile salts form lipophilic particles, and overcome the barrier of aqueous diffusion layer in the GIT (New & Kirby, Citation1999). The efficiency of SEDDS, SMEDDS or SNEDDS formulation is drug-dependent necessitating careful exploration of a successful working composition. Pre-assessment of CoQ10 solubility in the lipid components is mandated followed by construction of a phase diagram to determine the area of the self-emulsifying region and determination of the droplet size distribution. The design of experiments and the interaction of ingredients at different levels were attempted in the literature. Many investigators progressed in preparing a solid dosage form of the SEDDS to counteract the photoreactivity and enhance stability. The toxicity and bioavailability were important aspects to be investigated for CoQ10 SEDDS formulations. Kommuru et al. (Citation2001) developed SEDDS of CoQ10 using polyglycolyzed glycerides and assessed its bioavailability in dogs. Results motivated the use of medium-chain oils and Myvacet 9-45 over long-chain oils due to higher CoQ10 solubility while Labrafac CM-10 proved more competent than Labrasol in affording an efficient and a better self-emulsification process. Moreover, the spontaneity of self-emulsification was improved by the use of a co-surfactant. The optimized formulation achieved using Myvacet 9-45 (40%); Labrasol (50%) and lauroglycol (10%) afforded a 2-fold increase in the bioavailability compared to a CoQ10 powder formulation in dogs. A eutectic-based SNEDDS of CoQ10 was pioneered by Nazzal et al. (Citation2002c) to overcome the low solubility and irreversible precipitation of CoQ10 in various oils. A melting point depression (<37 °C) occurred upon mixing CoQ10 with menthol and/or various essential oils like spearmint oil, peppermint oil, lemon oil and anise oil. At a 50% w/w composition of lemon oil and CoQ10, the mixture was melted in body fluids within 5 min and dispersed to form a nanosized emulsion. The eutectic was incorporated into a tablet dosage form using a suitable blend of excipients meanwhile monitoring the emulsion release rate (Nazzal et al., Citation2002d). The levels of the tablet excipients, namely: maltodextrin, copolyvidone and microcrystalline cellulose (MCC) were optimized based on a response surface methodology and the interactions between different factors were evaluated for producing the optimum dry tablet (Nazzal et al., Citation2002b). The amounts of copolyvidone, maltodextrin and MCC showed a significant effect on the dissolution and release rate of SEDDS as well as on the physical and compaction properties of the dry emulsion-based tablet. By predicting the quantitative effect of these factors at different levels, an optimum formulation was achieved with a minimum weight, friability and disintegration time but with a maximum tensile strength and flowability index value. A Box–Behnken statistical design was adopted to optimize a limonene-based self-nanoemulsified capsule dosage form of CoQ10 in terms of the cumulative drug released at 5 min, release at 15 min, turbidity, particle size and zeta potential. An optimized formula was achieved with a fast drug release rate, low turbidity and small particle size (Palamakula et al., Citation2004). Markedly improved bioavailability in rats was obtained using the nanoemulsions. The enhancement in bioavailability by the primary and homogenized dry emulsion formulations of CoQ10 was attributed to its amorphization in the lipid vehicles and the subsequent dissolution enhancement meanwhile CoQ10 photodegradation was hampered for the dry emulsion forms (Hatanaka et al., Citation2008). Onoue et al. (Citation2012) prepared s-SEDDS of CoQ10 using medium-chain triglyceride, sucrose ester of fatty acid, and hydroxypropyl cellulose and tested it in rats. The absolute bioavailability was extremely low in case of the crystalline CoQ10 (0.44%) but it was improved to 2.2% by the oral administration of s-SEDDS, i.e. 5-fold enhancement. It is noteworthy that other studies showed that liquid SEDDS furnished a 2-fold increase in bioavailability over powder CoQ10 in rats and dogs (Kommuru et al., Citation2001; Balakrishnan et al., Citation2009). d-α-Tocopheryl polyethylene glycol succinate (TPGS) nanoemulsions improved the aqueous solubility of the phenolic biflavonoid (rutin) and increased its permeability across the everted gut sac studies while maintaining a high reactive oxygen species (ROS) scavenging and anti-inflammatory activity (Sharma et al., Citation2014).
A novel technology by Remedia termed the nanoemulsified composite system (NEC™) based on the incorporation of a double o/w/o microemulsion into a powder solid microporous carrier was patented and used for developing (Cardium™) as a highly bioavailable formulation of CoQ10 (Carli et al., Citation2005). The microemulsion loaded on the carrier yielded a powder with good flowability, dispersibility but more importantly, improved biopharmaceutical properties revealed by 10 times superior solubilization kinetics in aqueous buffers and three times higher bioavailability than commercial capsules. The enhanced biopharmaceutical properties were attributed to the very fine nanosized droplets (200–400 nm) released from the carrier. This size remained stable during storage at room temperature for 12 months.
To reduce the production cost, fats and emulsifiers accepted in the food industry in Japan were employed (Thanatuksorn et al., Citation2009). Olive oil, safflower oil, coconut oil, butter and cocoa butter together emulsifiers with variable HLBs (hydrophilic–lipophilic balance) namely: lecithin (HLB 3–4), monoglycerides (HLB 3–4), calcium stearoyl-2-lactate (CSL; HLB 7–9) and diacetyl tartaric acid esters of monoglycerides (DATEM; HLB 8–10) were used. Distilled water, with or without 8% skim milk as a stabilizer, constituted the aqueous phase of the emulsion. The emulsion with coconut oil, 8% skim milk and CSL provided efficient emulsification, good emulsion stability and small homogeneous droplet size. The formula improved the bioavailability from 2.2 - to 2.7-fold compared to a commercial encapsulated CoQ10 solubilized in olive oil and 2-fold significantly higher area under the curve (AUC) compared to CoQ10 powder. The findings were attributed to dual mechanisms pertaining to the medium-chain lauric acid, the major component in coconut oil: the high solubility of CoQ10 in lauric acid, as well as the existence of a special absorption route. The interplay of the effects of the HLB of the surfactants in emulsion formulations and the bile salts on the intestinal absorption of CoQ10 is particularly interesting. Sato et al. (Citation2013) screened surfactants with different HLBs namely: Tween-20, Tween-80, Tween-65 and Span-20 with HLBs 16.7, 15, 10.5 and 8.6, respectively in rats with or without ligation of the bile ducts. Higher absorption [AUC and Cmax (maximum drug concentration in blood/plasma)] was significantly displayed by Tween-20 and Tween-80 (higher HLB) than by Tween-65 and Span-20 owing to better permeation of the unstirred water layer on the intestinal apical membrane meanwhile negligible plasma levels of CoQ10 were recorded in the absence of bile salts. Based on these findings, a warning should be given to patients with cholestasis and cholelithiasis who receive a supplementary CoQ10. The cytotoxicity of SEDDS components is a crucial aspect that needs to be studied. The oils used in SEDDS were dispersed in Dulbecco’s modified Eagle’s Media (DMEM) by three different methods, namely: suspension, homogenizate and nanoemulsion. Caco-2 cells viability and monolayer integrity [mannitol permeability and transepithelial electrical resistance (TEER)] revealed that the nanoemulsions exhibited the least cytotoxicity (Palamakula & Khan, Citation2004).
CD complexes
CD inclusion complexes for CoQ10 were sought-after as a non-lipid based solubilizate with enhanced dissolution rate and improved stability against heat, oxidation and UV (Sharma & Baldi, Citation2014). Different complexation methods were explored including the kneading, heating and solubility methods at different molar ratios of CoQ10 and various substituted and non-substituted CDs (Lutka & Pawlaczyk, Citation1995, Citation1996a,Citationb). Complexes were made at a molar ratio 1:1 by kneading in the presence of 1.5 mM CDs in a mixture of ethanol/water, the phase solubility experiments showed an increase in the apparent solubility of CoQ10 in the order of α-CD < β-CD < γ-CD (Gao et al., Citation2006). Designed to be mixed with the food, an inclusion CoQ10/β-CD complex was patented to disintegrate and release CoQ10 in a pH < 3 with enhanced dissolution rate (Prosek et al., Citation2005). The, γ-CD and methyl-β-CD increased CoQ10 solubility in an aqueous solution and stabilized CoQ10 in the solid state (Lutka & Pawlaczyk, Citation1995, Citation1996a). The fitting of CoQ10 into the CDs cavity was investigated by appropriate calculations and/or analytical techniques. The strictly linear shape of CoQ10 molecule has been refuted and calculations were presented indicating that a folded conformation of CoQ10 must exist to enable its fitting into the β-CD cavity (Prosek et al., Citation2008). On the other hand, Nishimura et al. (Citation2008) verified that CoQ10, which has an isoprenoid unit similar in structure to the PEO-based surfactants, can form pseudorotaxane-like supramolecular complexes with γ-CD same as those formed between γ-CD and polyethylene glycol (PEG) (Yang et al., Citation2009) and polypropylene glycol (PPG) (Ooya & Yui, Citation1999; Wenz et al., Citation2006; Yang et al., Citation2007). Complexes were prepared by the solubility method and examined by X-ray diffraction (XRD), differential scanning calorimeter (DSC), Fourier transform infrared spectroscopy (FT-IR) and proton nuclear magnetic resonance (1H-NMR). Moreover, their dispersion rate and size distribution were determined. Results showed that the XRD pattern of the CoQ10/γ-CD complex was different from that of the physical mixture but was almost identical to PPG/γ-CD polypseudorotaxane. The interaction between CoQ10 and γ-CD in the solid state was confirmed by DSC and FT-IR. The stoichiometry ratio of the supramolecular complex was 5:1 (γ-CD:CoQ10) as indicated by 1H-NMR. The dispersion rate of CoQ10 was markedly increased due to the formation of nanosized supramolecular particles (Nishimura et al., Citation2008). Molecular mechanics and molecular dynamics proposed several complex structures for the CoQ10/γ-CD inclusion complexes with one of them having two molecules of CoQ10 and five molecules of γ-CD (Miyamoto et al., Citation2009). Structural studies and simulations showed that the isoprenoid chain of CoQ10 is shrouded in the γ-CD cavity while the quinone part is positioned outside, the later could be converted and stabilized by a reductant like vitamin C, to the quinol form due to hydrogen bonding between the hydroxyl group on the quinol and the one of the γ-CD. The finding on the generation of the quinol form of CoQ10 by the inclusion of vitamin C was particularly interesting and was further explored. The stability CoQ10–CDs complexes and vitamin C co-formulations was investigated at drastic storage conditions: 60 °C and 75% relative humidity. The free radical scavenging efficacy was tested using 1,1-diphenyl-2-picrylhydrazyl (DPPH) (Uekaji et al., Citation2010). Analysis of the oxidized and reduced CoQ10 by high-performance liquid chromatography (HPLC) revealed a significantly more ubiquinol (80%) formed in the γ-CD inclusion complex compared to the non-complexed CoQ10 (only 30%). The complex was stable with a little decomposition and displayed a higher free radical scavenging potential than un-complexed CoQ10. This simple and economic approach is a privilege in producing ubiquinol instead of the expensive and oxygen-free conditions. Clinically, it is promising for elderly patients who suffer a compromised reducing capacity as a result of diseases such as arteriosclerosis, stroke and diabetes. Many studies proceeded from the preparation and dissolution rate enhancement in vitro to the bioavailability testing in vivo with very promising results. At a molar ratio 1:10, CoQ10/γ-CD displayed significantly higher plasma levels as compared to CoQ10 and CoQ10/γ-CD physical mixture after oral administration to dogs. These results were attributed to the nanosized particles with enhanced aqueous dissolution rate (Gao et al., Citation2006). The intestinal absorptions of CoQ10/β-CD inclusion and a commercially available oil-based CoQ10 soft-gel capsules were compared in a group of beagle dogs (n = 3). A validated liquid chromatography–mass spectrometry (LC–MS) method was developed, the baseline CoQ10 plasma level was first determined then re-assessed after the oral dosing. The plasma profiles revealed the superiority of the inclusion complex over the commercial soft-gel capsules by ∼3-and 2-fold for AUC0–48h and Cmax, respectively whereas the Tmax (time to reach maximum drug concentration in blood/plasma) was shortened from 6 to 4 h (Prosek et al., Citation2008). The dimethyl β-CD as well as γ-CD members improved the dissolution rate and oral absorption of CoQ10 complexes in dogs (Lutka & Pawlaczyk, Citation1996b). In human volunteers, CoQ10/γ-CD inclusion complexes furnished a significantly higher Cmax and AUC values than CoQ10–MCC mixtures (Terao et al., Citation2006).
Solubilization
Sikorska et al. (Citation2003) derivatized α-tocopherol (vitamin E) to a water-soluble polyoxyethanyl-α-tocopheryl sebacate product (PTS) and used it for the solubilization of CoQ10. PTS was found to complex CoQ10 non-covalently at the molar ratio of 2:1 (PTS: CoQ10) giving a water-soluble product that was stable for extended periods of time. Following oral administration of the complex, superior levels of both vitamin E and CoQ10 were detected within 1 h in the rat plasma. The formulation’s efficacy was tested against an ischemic brain damage caused by a transient (8 min) bilateral occlusion of the common carotid arteries in rats. The animals received PTS-CoQ10 by two intraperitoneal injections which were given immediately after ischemia and 3 h later and the brain damage was assessed up to 12 days post-ischemia. A significant neuro-protection was observed where 50% of the neurons were still alive in the treated group versus <5% in the non-treated group demonstrating potential for application in acute conditions such as stroke or cardiac arrest (Sikorska et al., Citation2003). A novel CoQ10 solubilizate (Solu™ Q10) was developed and compared to four marketed CoQ10 products (Schulz et al., Citation2006). The pharmacokinetic parameters were assessed after single and multiple oral supplementations of 60 mg CoQ10 over a 2-weeks period in 54 healthy volunteers. Following a single dose, the solubilizate showed significantly elevated AUC0–4h over crystalline CoQ10 while the long-term supplementation revealed significantly higher plasma levels for all the formulations tested (crystalline powder in hard gelatin capsules, as well as oily dispersions and two solubilizates of CoQ10 in soft-gel capsules). Interestingly, the highest plasma CoQ10 level was displayed by the Solu™ Q10 which also furnished increased intracellular CoQ10 levels in the human buccal mucosal cells. A dispersion of CoQ10 was developed using phospholipids and sodium glycocholate in the absence of ethanol as a cosolvent. The solubilizate exhibited submicron-sized particles with an enhanced dissolution rate and prevented the recrystallization of CoQ10. In vitro, the formulation significantly promoted blastocyst formation, proliferation and hatching and boosted energy in a bovine embryo cell culture (Stojkovic et al., Citation1999). Hydrotropic solubilization of CoQ10 was attempted using N,N-diethylnicotinamide and N,N-diethylbenzamide hydrotropes. The later hydrotrope resulted in a 1000-fold increase in the aqueous solubility due to a more hydrophobic nature and hence more solubilizing power (Kim et al., Citation2010).
Others (comparison of different formulation strategies)
Ullmann et al. (Citation2005) reported All-Q®, a starch-based tablet formulation of CoQ10, and compared its bioavailability to two other CoQ10 formulations namely: Q-Gel softsules and Q-SorB in 12 human volunteers. The highest Cmax and AUC were demonstrated in case of Q-Gel followed by All-Q® and both were bioequivalent but exhibited better bioavailability than Q-SorB. A more detailed study compared the bioavailability of different formulations of CoQ10. Colloidal-Q10 was produced using the VESIsorb technology which emulates the physiological solubilization by the bile salt-mixed micelles (Liu & Artmann, Citation2009). This product was weighed against solubilizate 1 (60 mg CoQ10 per capsule), oil-based formulation (30 mg CoQ10 per capsule) and solubilizate 2 (30 mg CoQ10 per capsule). A double-blind, comparative, controlled pharmacokinetic study with parallel design was adopted in which human subjects were randomly assigned for a single 120 mg dose in the morning on an empty stomach, and were given standard meals thereafter. Results revealed that the highest Cmax values were displayed with colloidal-Q10. The AUC0–10h values were 30.6, 6.1, 4.9 and 10.7 μg/ml h for colloidal-Q10, solubilizate 1, oil-based formulation and solubilizate 2, respectively and the differences were statistically significant. The bioavailability enhancement furnished by the colloidal-Q10 was justified by the enhancement of the rate and extent of dissolution rather than facilitation of the lymphatic absorption associated with the oily formulations.
Strategies for surmounting metabolism barriers
Oral drug bioavailability is a complex process involving solubility, permeability, gastrointestinal stability and efflux/influx transporters but equally important are the metabolizing enzymes particularly cytochrome P450 enzymes (CYPs). Little is known about the metabolism of CoQ10 by CYPs except that it undergoes structural changes when exposed to CYPs in vitro giving new hydroxylated (O-demethylated) derivatives with the same ability to bind and transport calcium across the bio-membranes in vitro but with a higher antioxidant effect (Bogeski et al., Citation2011). Piperine-mediated drug bioavailability enhancement was underlined by multiple effects; apolar complexes formation (Srinivasan, Citation2007), efflux transport (Bhardwaj et al., Citation2002) and/or enzyme inhibition (Atal et al., Citation1985; Koul et al., Citation2000; Venkatesh et al., Citation2011). The finding that piperine enhances the bioavailability of CoQ10 has been partially attributed to inhibition of CYPs metabolism (Badmaev et al., Citation2000).
Strategies for surmounting permeability barriers
CoQ10 permeability through the GI membranes needs to be addressed more thoroughly for improving its oral delivery. As expected from a lipophilic compound, the absorption is enhanced in the presence of food due to micellar solubilization by the bile salts, uptake by the chylomicrons and lymphatic absorption (Katayama & Fujita, Citation1972). The same justification applies for microemulsion and self-emulsifiable formulations where the surfactants and cosurfactants are claimed to affect absorbing membrane permeability (Gursoy & Benita, Citation2004; Hatanaka et al., Citation2008). The food increased oral absorption of CoQ10 in a suspension and an emulsion. The latter had higher pharmacokinetics parameters in the absence and presence of food presumably due to the surfactants in the emulsion (Ochiai et al., Citation2007). The cellular accumulation of CoQ10 in Caco-2 cells was shown to depend on the efficiency of CoQ10 micellization after in vitro digestion as well as on formulation type where a CoQ10/γ-CD complex powder superseded a liposomal formulation (Bhagavan et al., Citation2007). Different solvents were used for cell culture permeability studies; ethyl acetate was promising in terms of miscibility with DMEM, CoQ10 solubilization and safety to cells (up to 5% concentration) (Palamakula, Citation2004b). Palamaluka (2004b) reported high CoQ10 permeability values through Caco-2 cell monolayer and categorized it as a Class II compound in the BCS. However, this categorization is questionable based on the extremely low absorption (Fa% <1%) (Barakat et al., Citation2013) and apparent permeability coefficient (Papp) values (<1 × 10−7 cm/s) of CoQ10 (Xia et al., Citation2009). As such, CoQ10 should be sorted as a Class IV compound. Transport of CoQ10 was suggested to occur by both passive diffusion as well as carrier-mediated transport mechanisms. Using a library of substrates/inhibitors, multiple transporters for CoQ10 were identified including: the peptide transporters, organic cation/camitine transporters and organic anion transporters (Palamakula, Citation2004b). The same group of researchers used the isolated rat gut method to study the regional permeability of CoQ10; their data illustrated regional differences in permeability throughout the GIT (Palamakula et al., Citation2005). Interestingly, the maximum permeability occurred in the duodenum pointing at the peculiar expression of transporters along the GIT. The second most favorable region for absorption was the colon followed by the jejunum and stomach. The occurrence of regional variation in CoQ10 absorption or the existence of an absorption window is corroborated by the non-linear pharmacokinetics behavior of CoQ10. Such a finding should draw the formulators’ attention to the use of gastro-retentive dosage form for CoQ10. The caco-2 Papp values reported by Xia et al. (Citation2009) were 0.33 × 10−7 and 0.34 × 10−6 cm/s for a marketed liquid formulation and powder dispersion, respectively. These values contradicted the high Papp values reported by Palamaluka (2.27 × 10−3 cm/s) (Palamakula, Citation2004b). An intestinal absorption cutoff value of 1 × 10−6 cm/s has been set for highly (100%) absorbed drug while a cutoff value of 1 × 10−7 cm/s was indicative of absorption of <1% (Artursson & Karlsson, Citation1991). Accordingly, CoQ10 with its very low absorption and bioavailability should be categorized as a BCS Class IV compound. Xia et al. (Citation2006, Citation2007, Citation2009) developed a nanoliposomal CoQ10 (with and without Tween-80) and measured its Papp. Interestingly, the Papp values were 20-fold (0.65 × 10−6 cm/s) and 125-fold 80 (4.19 × 10−6 cm/s) higher for nanoliposomal formulation with and without Tween-80, respectively. The modus operandi of nanoliposomes-enhanced permeability of CoQ10 was attributed to an intermembrane transfer, adsorption, fusion and endocytosis allowing CoQ10 and phospholipids to localize into the plasma membrane and subcellular organelles, and to affect the cell association, transmembrane flux and binding to intracellular targeting sites (Xia et al., Citation2009; Lo, Citation2000). Mechanistically, the nanoliposomal CoQ10 displayed a passive diffusion and carrier-mediated transport mechanism. The nanoliposomes were able to inhibit the P-glycoprotein (P-gp) efflux of CoQ10 by virtue of its Tween-80 content which increased membrane fluidity (Xia et al., Citation2006, Citation2007). The nanoliposomes showed an accumulation of the ubiquinol form inside the cells which was justified by the redox transformation of ubiquinone to ubiquinol while transport across the caco-2 cells (Xia et al., Citation2009). The P-gp efflux of CoQ10 was confirmed by other studies showing that the cellular uptake of CoQ10 in caco-2 cells was increased in the presence of grape fruit juice as a P-gp inhibitor while the secretory (basal to apical) was reduced (Itagaki et al., Citation2008, Citation2010). The improvement in CoQ10 absorption (by 30%) by the co-administration of piperine (black pepper extract) was thought to be due to a non-specific mechanism (increase blood flow to the GIT) and/or thermo-nutrient effect of piperine (Khajuria et al., Citation1998; Badmaev et al., Citation2000). Recently reported data on the inhibitory effect of piperine on P-gp-mediated cellular efflux (Jin & Han, Citation2010) could be also an asset. These findings should be utilized for modulation of CoQ10 intestinal absorption.
Strategies for maximizing intracellular delivery
Nanocarriers for tissue target-ability and intracellular delivery
Ankola et al. (Citation2007), developed oral nanoparticulate formulations of CoQ10 using poly (lactide-co-gylcolide) (PLGA) biodegradable polymer and the quaternary ammonium salt: didodecyl dimethyl ammonium bromide (DMAB) as a surfactant/stabilizer. Using the in situ rat jejunum uptake model, the intestinal uptake of the nanoparticles was 79% compared to 75 and 45% uptake in the case of a commercial formulation and a carboxymethylcellulose suspension, respectively. Results also revealed the superiority of CoQ10 nanoparticles in normalizing the blood pressure in a renal hypertensive rat model and its higher efficacy in reducing levels of lipid peroxides in plasma (Ankola et al., Citation2007). Swarnakar et al. (Citation2011) later on reported a DMAB-stabilized CoQ10–PLGA nanoparticles formulation that accumulated in the inflamed organs in rats, demonstrated hepatoprotective and anti-inflammatory efficacy and produced more than a 4-fold increase in oral bioavailability compared to a CoQ10 suspension. Novel and multifunctional CoQ10 and quantum dot co-loaded nanoparticles were proposed for simultaneous drug delivery and imaging applications. Confocal microscopy revealed that the nanoparticles were biocompatible to and associated with PC12 cells (pheochromocytoma of the rat adrenal medulla) 1-day post-treatment (Nehilla et al., Citation2008). A synergistic antioxidant combination of ellagic acid and CoQ10 coencapsulated in nanoparticles has been tested in hyperlipidemic rats. After a 2-weeks treatment, the combination lowered cholesterol, glucose and triglycerides levels for extended periods and improved endothelial functioning (Ratnam et al., Citation2009). NLC are considered a potential bioavailability enhancement tool (Gaba et al., Citation2014). NLC of CoQ10 enhanced the aqueous dissolution and lowered the cytotoxicity as compared to a CoQ10 free suspension (Wang et al., Citation2012). Glyceryl distearate and glyceryl behenate (as a solid lipid) and glyceryl triacetate (as a liquid lipid) furnished NLC formulations with enhanced efficacy (in terms of DPPH scavenging, anti-lipid peroxidation, reduced scopolamine-induced amnesia) and higher rate and extent of bioavailability than a solution form (Nanjwade et al., Citation2013). Heart tissue targeting was attempted using lecithin and TPGS-based nanoemulsions of CoQ10 (Zhou et al., Citation2013a). Following an intravenous administration, 2.8-folds higher myocardium levels of CoQ10 were achieved in as fast as 5-min onset and was further maintained for 90 min by the TPGS- than lecithin-containing nanoemulsions presumable to due to the P-gp inhibitory effect of TPGS (Zaki et al., Citation2013, Zaki 2014). Extending the circulation time of lipid nanocarriers in the blood has been explored as a different manoeuvre to increase bioavailability of CoQ10 liposomes by grafting the inert hydrophilic polymers (PEG) into the lipid components. Sterically stabilized glyceryl dioleate-PEG12 and glyceryl dipalmitate-PEG23 were developed (Koynova & Tihova, Citation2010). In aqueous media, the hydrated diacylglycerol-PEG lipid conjugates spontaneously formed liquid crystals of uni- or oligolamellar liposomes. Dry mixing, rather than use of organic solvents, was used for the encapsulation of up to 30% by weight of CoQ10. The 50-nm sized self-emulsifying lipid vesicles had the privilege of expedited intracellular release of CoQ10. Nanocapsules prepared by high pressure homogenization using lecithin improved the relative oral bioavailability of CoQ10 in mice (176.6% over tablets) (Zhou et al., Citation2013b). Verma et al. (Citation2007) reported a liposomal CoQ10 with enhanced intracellular delivery and significantly lowered fraction of irreversibly damaged myocardium administered by intracoronary infusion in an occlusion-induced ischemic zone in rabbits.
Strategies for maximizing mitochondrial targeting
Mitochondrially targeted CoQ10
The mitochondrion is the target for antioxidants including CoQ10 since it is the major site of ROS generation within cells (Murphy & Smith, Citation2000). Limited mitochondrial bioavailability of CoQ10 resulted in a clinical inefficacy. Hence, mitochondrially targeted CoQ10 formulations were sought-after. Membrane-penetrating triphenyl alkyl phosphonium cations (TPPBr) were reported earlier by Skulachev et al. (Citation2005) for their ability to measure the mitochondrial potential (Samartsev et al., Citation2000; Severina et al., Citation2007). Recently, Murphy (Citation2008) and Murphy & Smith (Citation2007) pioneered the mitochondrial-targeting of antioxidants using these lipophilic cations and others (Adlam et al., Citation2005; Brown et al., Citation2007; Smith et al., Citation1999, Citation2011). Specifically, they were used to target CoQ to mitochondria by chemical conjugation of TPPBr with CoQ10 forming the so called mitochondrially targeted CoQ10 (MitoQ10) as shown in . The MitoQ10 conjugates move easily through membrane phospholipid bilayers by a non-specific uptake mechanism (Murphy & Smith, Citation2007; Murphy, Citation2008; Porteous et al., Citation2010). By virtue of their cationic nature, the TPPBr sturdily interacts with the highly negative mitochondrial membrane (−150 to 170 mV) which triggers a several hundred-fold accumulation of MitoQ10 within the mitochondria (Murphy & Smith, Citation2007).
Several cationic ubiquinones MitoQn with different aliphatic chain lengths (n = 3, 5 and 10) were developed with tailored hydrophobicities aiming to modify the partitioning between the lipid mitochondrial membrane and the aqueous phase (Lin & Engbersen, Citation2009). Fortunately, all derivatives exhibited antioxidant effect but the optimal antioxidant efficacy was exhibited by MitoQ10. Li et al. (Citation2008) studied the absorptive and secretory transport and metabolism of MitoQn species in caco-2 cell monolayers. The intracellular accumulation was proportional to the lipophilicity. On the contrary, the apical to basolateral permeability (Papp A → B) was inversely related to lipophilicity being high for MitoQ3 and low for MitoQ5 and MitoQ10. A higher secretory than absorptive transport was shown with efflux ratios (Papp B → A/Papp A → B) 2.3, 24.9 and 4.0 for n = 3, 5 and 10, respectively. Cyclosporine A increased Papp A → B and decreased Papp B → A. Bovine serum albumin, added in the basolateral side to provide sink condition, enhanced the Papp A → B by 9-fold of MitoQ10 (log P 3.44) but did not improve that of MitoQ5 (log P 1.14). While transport a synchronized turnover from ubiquinone to reduced ubiquinol species was detected.
Mitochondrially targeted nanosystems
Mitochondria-targeted nanosystems for CoQ10 were developed using multifunctional star polymers based on ABC miktoarm [A = polyethylene glycol (PEG), B = polycaprolactone (PCL) and C = TPPBr self-assembled into nanosized micelles (Sharma et al., Citation2012). As depicted in , the micelles exhibited a small critical association concentration, maintained colloidal stability for 3 months and afforded a high CoQ10 loading capacity (60%). Intracellular trafficking of a mitochondria-specific vital dye and a fluorescently labeled polymer revealed mitochondrial delivery of the nanosystems. The nanosystems proved its efficacy in oxidative stress and inflammation at two different experimental paradigms.
The enhanced therapeutic efficacy of the cationic CoQ10 nanoparticles stabilized by DMAB has been attributed to an enhanced mitochondrial delivery as a result of the interaction with the highly negative mitochondrial membrane (−150 to 170 mV). This was evidenced by a co-localization of the nanoparticles with the mitochondria and lysosomes (Swarnakar et al., Citation2011).
Future perspectives
Nutrients gain a lot by being formulated and administered in the form of nutraceutical products; the wonder nutrient CoQ10 is not an exception. Endless endeavors have been put into formulating it in a medicinal form that can improve its oral bioavailability. Progress to better understanding of its physicochemical, pharmaceutical, biopharmaceutical and biological properties will unravel areas never explored before. For example, the low melting point (48 °C) and the grease ball nature (underprivileged solubility attributable to high lipophilicity), should refrain attempts of amorphization that better suits a brick dust compound (inadequate solubility due to a stable crystal lattice). For a dissolution evaluation, bio-relevant dissolution media such as the fasted- and fed-state simulated intestinal fluids (FaSSIF and FeSSIF, respectively) should be considered for CoQ10 to avoid underrated physiological solubility and absorption. In the same context, the issue of concomitant food intake is crucial when evaluating and comparing the bioavailability of different CoQ10 formulations.
In terms of future development of targeted dosage forms for CoQ10, the existence of absorption window should draw attention to a specific formulation that provides extended residence in this region of the GIT, e.g. a gastro-retentive dosage form. In the context of permeability, more research should be done to explore active and passive transport of CoQ10, by studying permeability on cell lines not functionally expressing transporters e.g. 2/4/A1 cell line [rat small intestinal cells conditionally immortalized with a temperature sensitive mutant of Simian virus 40 (SV40T)] to delineate the effect of transporters on CoQ10 permeability. Alternatively, “pure” measures of permeability like liposome partitioning, retention on immobilized artificial membranes (IAM), the parallel artificial membrane permeability assay (PAMPA) or binding to liposomes measured by surface plasmon resonance (SPR) biosensors would help in evaluating the passive permeability of CoQ10 apart from active transport. The effect of metabolizing enzymes like CYPs on CoQ10 bioavailability need to be probed using some predictive-metabolism tools as rapid filters for testing CoQ10 for CYPs liability. The complex absorption of CoQ10 from gut by both active and passive transport as well as the presence of multiple transporters might trigger scientists to design a prodrug for targeting a specific transporter on the cell surface. The possibility of having a drug–drug interaction at the transporter level would be crucial for geriatrics receiving CoQ10 supplementation concomitantly with other drugs. In this case, mutually altered pharmacokinetics cannot be ruled out. With deeper knowledge of transporters for CoQ10, especially at epithelial and endothelial barriers such as the liver, kidney, intestine and blood–brain barrier, it is foreseeable that human pathologies of these organs might be better treated using CoQ10 supplements. Last but not the least, prodrug approach for designing cationic ubiquinone antioxidants (MitoQn) should be optimally tailored in terms lipophilicity, transporter interaction, protein binding and affinity to metabolizing enzymes for successful mitochondrial targeting.
Declaration of interest
The author reports no conflicts of interest. The author alone is responsible for the content and writing of this article.
References
- Adlam VJ, Harrison JC, Porteous CM, et al. (2005). Targeting an antioxidant to mitochondria decreases cardiac ischemia-reperfusion injury. FASEB J 19:1088–95
- Al-Hasso S. (2001). Coenzyme Q10: a review. Hospital Pharmacy 36:51–5
- Ankola DD, Viswanad B, Bhardwaj V, et al. (2007). Development of potent oral nanoparticulate formulation of coenzyme Q10 for treatment of hypertension: can the simple nutritional supplements be used as first line therapeutic agents for prophylaxis/therapy? Eur J Pharm Biopharm 67:361–9
- Araya H, Nagao S, Tomita M, Hayashi M. (2005a). The novel formulation design of self-emulsifying drug delivery systems (SEDDS) type O/W microemulsion I: enhancing effects on oral bioavailability of poorly water soluble compounds in rats and beagle dogs. Drug Metab Pharmacokinet 20:244–56
- Araya H, Tomita M, Hayashi M. (2005b). The novel formulation design of self-emulsifying drug delivery systems (SEDDS) type O/W microemulsion II: stable gastrointestinal absorption of a poorly water soluble new compound, ER-1258 in bile-fistula rats. Drug Metab Pharmacokinet 20:257–67
- Artursson P, Karlsson J. (1991). Correlation between oral drug absorption in humans and apparent drug permeability coefficients in human intestinal epithelial (Caco-2) cells. Biochem Biophys Res Commun 175:880–5
- Atal CK, Dubey RK, Singh J. (1985). Biochemical basis of enhanced drug bioavailability by piperine: evidence that piperine is a potent inhibitor of drug metabolism. J Pharmacol Exp Ther 232:258–62
- Avis HJ, Hargreaves IP, Ruiter JP, et al. (2011). Rosuvastatin lowers coenzyme Q10 levels, but not mitochondrial adenosine triphosphate synthesis, in children with familial hypercholesterolemia. J Pediatr 158:458–62
- Badmaev V, Majeed M, Prakash L. (2000). Piperine derived from black pepper increases the plasma levels of coenzyme Q10 following oral supplementation. J Nutr Biochem 11:109–13
- Baker SK, Tarnopolsky MA. (2003). Targeting cellular energy production in neurological disorders. Expert Opin Investig Drugs 12:1655–79
- Balakrishnan P, Lee BJ, Oh DH, et al. (2009). Enhanced oral bioavailability of coenzyme Q10 by self-emulsifying drug delivery systems. Int J Pharm 374:66–72
- Bank G, Kagan D, Madhavi D. (2011). Coenzyme Q10: clinical update and bioavailability. J Evid Based Complementary Altern Med 16:129–37
- Barakat A, Shegokar R, Dittgen M, Müller RH. (2013). Coenzyme Q10 oral bioavailability: effect of formulation type. J Pharm Invest 43:431–45
- Bhagavan HN, Chopra RK. (2006). Coenzyme Q10: absorption, tissue uptake, metabolism and pharmacokinetics. Free Radic Res 40:445–53
- Bhagavan HN, Chopra RK. (2007). Plasma coenzyme Q10 response to oral ingestion of coenzyme Q10 formulations. Mitochondrion 7:S78–88
- Bhagavan HN, Chopra RK, Craft NE, et al. (2007). Assessment of coenzyme Q10 absorption using an in vitro digestion-Caco-2 cell model. Int J Pharm 333:112–17
- Bhandari KH, Newa M, Kim JA, et al. (2007). Preparation, characterization and evaluation of coenzyme Q10 binary solid dispersions for enhanced solubility and dissolution. Biol Pharm Bull 30:1171–6
- Bhardwaj RK, Glaeser H, Becquemont L, et al. (2002). Piperine, a major constituent of black pepper, inhibits human P-glycoprotein and CYP3A4. J Pharmacol Exp Ther 302:645–50
- Bogeski I, Gulaboski R, Kappl R, et al. (2011). Calcium binding and transport by coenzyme Q. J Am Chem Soc 133:9293–303
- Brown SE, Ross MF, Sanjuan-Pla A, et al. (2007). Targeting lipoic acid to mitochondria: synthesis and characterization of a triphenylphosphonium-conjugated alpha-lipoyl derivative. Free Radic Biol Med 42:1766–80
- Caliph SM, Charman WN, Porter CJH. (2000). Effect of short-, medium-, and longchain fatty acid-based vehicles on the absolute oral bioavailability and intestinal lymphatic transport of halofantrine and assessment of mass balance in lymphcannulated and non-cannulated rats. J Pharm Sci 89:1073–84
- Carli F, Chiellini EE, Bellich B, et al. (2005). Ubidecarenone nanoemulsified composite systems. Int J Pharm 291:113–18
- Cheng B, Yuan QP, Sun XX, Li WJ. (2010). Enhanced production of coenzyme Q10 by overexpressing HMG-CoA reductase and induction with arachidonic acid in Schizosaccharomyces pombe. Appl Biochem Biotechnol 160:523–31
- Cooper JM, Schapira AH. (2003). Friedreich’s Ataxia: disease mechanisms, antioxidant and coenzyme Q10 therapy. Biofactors 18:163–71
- Cordero MD, Alcocer-Gómez E, De Miguel M, et al. (2013). Can coenzyme Q10 improve clinical and molecular parameters in fibromyalgia? Antioxid Redox Signal 19:1356–61
- Costantinides PP. (1985). Lipid microemulsion for improving drug dissolution and oral absorption: physical and biopharmaceutical aspects. Pharm Res 12:161–72
- Crane FL. (2001). Biochemical functions of coenzyme Q10. J Am Coll Nutr 20:591–8
- Dimauro S, Hirano M, Schon EA. (2006). Approaches to the treatment of mitochondrial diseases. Muscle Nerve 34:265–83
- Dokania S, Joshi AK. (2014). Self-microemulsifying drug delivery system (SMEDDS) – challenges and road ahead. Drug Deliv [Epub ahead of Print]
- Dos Santos GC, Antunes LMG, Dos Santos AC, Bianchi MDLP. (2009). Coenzyme Q10 and its effects in the treatment of neurodegenerative diseases. Brazilian J Pharm Sci 45:607–18
- Eaimtrakarn S, Rama Prasad YV, Ohno T, et al. (2002). Absorption enhancing effect of labrasol on the intestinal absorption of insulin in rats. J Drug Target 10:255–60
- Ebadi M, Govitrapong P, Sharma S, et al. (2001). Ubiquinone (coenzyme q10) and mitochondria in oxidative stress of parkinson’s disease. Biol Signals Recept 10:224–53
- El-Abhar HS. (2010). Coenzyme Q10: a novel gastroprotective effect via modulation of vascular permeability, prostaglandin E, nitric oxide and redox status in indomethacin-induced gastric ulcer model. Eur J Pharmacol 649:314–19
- Eriksson JG, Forsen TJ, Mortensen SA, Rohde M. (1999). The effect of coenzyme Q10 administration on metabolic control in patients with type 2 diabetes mellitus. Biofactors 9:315–18
- Eussen S, Klungel O, Garssen J, et al. (2010). Support of drug therapy using functional foods and dietary supplements: focus on statin therapy. Br J Nutr 103:1260–77
- Frankovic M. (2006). Introduction to CoQ10. Agro Food Industry Hi-Tech 17:12–13
- Fujimoto S, Kurihara N, Hirata K, Takeda T. (1993). Effects of coenzyme Q10 administration on pulmonary function and exercise performance in patients with chronic lung diseases. Clin Investig 71:S162–6
- Gaba B, Fazil M, Ali A, et al. (2014). Nanostructured lipid (NLCs) carriers as a bioavailability enhancement tool for oral administration. Drug Deliv [Epub ahead of Print]
- Gao X, Nishimura K, Hirayama F, et al. (2006). Enhanced dissolution and oral bioavailability of coenzyme Q10 in dogs by inclusion complexation with γ-cyclodextrin. Asian J Pharm Sci 1:95–102
- Gawish AS, Zaki NM, Ola MG, Sadel HS. (2011). Clinical and microbiological assessment of Coenzyme Q-10 gel in treatment of chronic periodontitis. Egypt Dent J 57:1–9
- Gürkan AS, Bozdaǧ-Dündar O. (2005). Coenzyme Q10. Ankara Universitesi Eczacilik Fakultesi Dergisi 34:129–54
- Gursoy RN, Benita S. (2004). Self-emulsifying drug delivery systems (SEDDS) for improved oral delivery of lipophilic drugs. Biomed Pharmacother 58:173–82
- Hamilton SJ, Chew GT, Watts GF. (2009). Coenzyme Q10 improves endothelial dysfunction in statin-treated type 2 diabetic patients. Diabetes Care 32:810–12
- Hargreaves IP. (2003). Ubiquinone: cholesterol’s reclusive cousin. Ann Clin Biochem 40:207–18
- Hargreaves IP, Duncan AJ, Heales SJR, Land JM. (2005). The effect of HMG-CoA reductase inhibitors on coenzyme Q10: possible biochemical/clinical implications. Drug Safety 28:659–76
- Hatanaka J, Kimura Y, Lai-Fu Z, Onoue S, Yamada S. (2008). Physicochemical and pharmacokinetic characterization of water-soluble coenzyme Q(10) formulations. Int J Pharm 363:112–17
- Hathcock JN, Shao A. (2006). Risk assessment for coenzyme Q10 (ubiquinone). Regul Toxicol Pharmacol 45:282–8
- Hyson HC, Kieburtz K, Shoulson I, et al. (2010). Safety and tolerability of high-dosage coenzyme Q10 in Huntington’s disease and healthy subjects. Mov Disord 25:1924–8
- Ikematsu H, Nakamura K, Harashima S, et al. (2006). Safety assessment of coenzyme Q10 (Kaneka Q10) in healthy subjects: a double-blind, randomized, placebo-controlled trial. Regul Toxicol Pharmacol 44:212–18
- Itagaki S, Ochiai A, Kobayashi M, et al. (2008). Interaction of coenzyme Q10 with the intestinal drug transporter P-glycoprotein. J Agric Food Chem 56:6923–7
- Itagaki S, Ochiai A, Kobayashi M, et al. (2010). Grapefruit juice enhance the uptake of coenzyme Q10 in the human intestinal cell-line Caco-2. Food Chem 120:552–5
- Janicki B, Buzała M. (2012). Role of coenzyme Q10 in humans and animals. Rola Koenzymu Q10 w Organizmie Ludzi i Zwierz̧t 68:214–17
- Jin MJ, Han HK. (2010). Effect of piperine, a major component of black pepper, on the intestinal absorption of fexofenadine and its implication on food-drug interaction. J Food Sci 75:H93–6
- Kaikkonen J, Tuomainen TP, Nyyssonen K, Salonen JT. (2002). Coenzyme Q10: absorption, antioxidative properties, determinants, and plasma levels. Free Radic Res 36:389–97
- Katayama K, Fujita T. (1972). Studies on the lymphatic absorption of 1′,2′-(3H)-coenzyme Q10 in rats. Chem Pharm Bull 250:2585–92
- Kawabata Y, Wada K, Nakatani M, et al. (2011). Formulation design for poorly water-soluble drugs based on biopharmaceutics classification system: basic approaches and practical applications. Int J Pharm 420:1–10
- Kawasaki T. (1992). Antioxidant function of coenzyme Q. J Nutr Sci Vitaminol 38:552–5
- Khajuria A, Zutshi U, Bedi KL. (1998). Permeability characteristics of piperine on oral absorption–an active alkaloid from peppers and a bioavailability enhancer. Indian J Exp Biol 36:46–50
- Kim JY, Kim S, Papp M, et al. (2010). Hydrotropic solubilization of poorly water-soluble drugs. J Pharm Sci 99:3953–65
- Kommuru TR, Ashraf M, Khan MA, Reddy IK. (1999). Stability and bioequivalence studies of two marketed formulations of coenzyme Q10 in beagle dogs. Chem Pharm Bull 47:1024–8
- Kommuru TR, Gurley B, Khan MA, Reddy IK. (2001). Self-emulsifying drug delivery systems (SEDDS) of coenzyme Q10: formulation development and bioavailability assessment. Int J Pharm 212:233–46
- Koul S, Koul JL, Taneja SC, et al. (2000). Structure-activity relationship of piperine and its synthetic analogues for their inhibitory potentials of rat hepatic microsomal constitutive and inducible cytochrome P450 activities. Bioorg Med Chem 8:251–68
- Koynova R, Tihova M. (2010). Nanosized self-emulsifying lipid vesicles of diacylglycerol-PEG lipid conjugates: biophysical characterization and inclusion of lipophilic dietary supplements. Biochim Biophys Acta 1798:646–53
- Kwon SS, Nam YS, Lee JS, et al. (2002). Preparation and characterization of coenzyme Q10-loaded PMMA nanoparticles by a new emulsification process based on microfluidization. Colloids Surf A Physicochem Eng Asp 210:95–104
- Land JM, Heales SJR, Duncan AJ, Hargreaves IP. (2007). Some observations upon biochemical causes of ataxia and a new disease entity ubiquinone, CoQ10 deficiency. Neurochem Res 32:837–43
- Langsjoen PH, Langsjoen AM. (2003). The clinical use of HMG CoA-reductase inhibitors and the associated depletion of coenzyme Q10. A review of animal and human publications. Biofactors 18:101–11
- Li Y, Fawcett JP, Zhang H, Tucker IG. (2008). Transport and metabolism of some cationic ubiquinone antioxidants (MitoQn) in Caco-2 cell monolayers. Eur J Drug Metab Pharmacokinet 33:199–204
- Lin C, Engbersen JF. (2009). The role of the disulfide group in disulfide-based polymeric gene carriers. Expert Opin Drug Deliv 6:421–39
- Lindmark T, Nikkila T, Artusson P. (1995). Mechanism of absorption enhancement by medium chain fatty acids in intestinal epithelial Caco-2 monolayers. J Pharmacol Exp Ther 275:958–64
- Littarru GP, Tiano L. (2005). Clinical aspects of coenzyme Q10: an update. Curr Opin Clin Nutr Metab Care 8:641–6
- Littarru GP, Tiano L. (2007). Bioenergetic and antioxidant properties of coenzyme Q10: recent developments. Mol Biotechnol 37:31–7
- Littarru GP, Tiano L. (2010). Clinical aspects of coenzyme Q10: an update. Nutrition 26:250–4
- Littlefield N, Beckstrand RL, Luthy KE. (2013). Statins’ effect on plasma levels of Coenzyme Q10 and improvement in myopathy with supplementation. J Am Assoc Nurse Pract 26:85–90
- Liu ZX, Artmann C. (2009). Relative bioavailability comparison of different coenzyme Q10 formulations with a novel delivery system. Altern Ther Health Med 15:42–6
- Lo YL. (2000). Phospholipids as multidrug resistance modulators of the transport of epirubicin in human intestinal epithelial Caco-2 cell layers and everted gut sacs of rats. Biochem Pharmacol 60:1381–90
- Lutka A, Pawlaczyk J. (1995). Inclusion complexation of coenzyme Q10 with cyclodextrins. Acta Pol Pharm 52:379–86
- Lutka A, Pawlaczyk J. (1996a). Investigation of inclusion complexes of coenzyme Q10 with c-cyclodextrin and methyl-b-cyclodextrin (MS = 0.97). Part I. Comparison of complexation methods in the solution state. Acta Pol Pharm 53:193–6
- Lutka A, Pawlaczyk J. (1996b). Investigation of inclusion complexes of coenzyme Q10 with cyclodextrin and methyl-b-cyclodextrin (MS = 0.97). Part II. The influence of complexation temperature (heating method) on coenzyme Q10 solubility. Acta Pol Pharm 53:197–201
- Mahlin D, Bergstrom CA. (2013). Early drug development predictions of glass-forming ability and physical stability of drugs. Eur J Pharm Sci 49:323–32
- Makabi-Panzu B, Sprott GD, Patel GB. (1998). Coenzyme Q10 in vesicles composed of archaeal ether lipids or conventional lipids enhances the immuno-adjuvanticity to encapsulated protein. Vaccine 16:1504–10
- Marcoff L, Thompson PD. (2007). The role of coenzyme Q10 in statin-associated myopathy. A systematic review. J Am Coll Cardiol 49:2231–7
- Matejikova J, Kucharska J, Pancza D, Ravingerova T. (2008). The effect of antioxidant treatment and NOS inhibition on the incidence of ischemia-induced arrhythmias in the diabetic rat heart. Physiol Res 57:S55–60
- Matsuda Y, Masahara R. (1983). Photostability of solid-state ubidecarenone at ordinary and elevated temperatures under exaggerated UV irradiation. J Pharm Sci 72:1198–203
- Mauludin R. (2009). Nanosuspensions of poorly soluble drugs for oral administration [PhD Thesis]. Berlin: Freie Universität Berlin
- Meng X, Zu Y, Zhao X, et al. (2012). Characterization and pharmacokinetics of coenzyme Q10 nanoparticles prepared by a rapid expansion of supercritical solution process. Pharmazie 67:161–7
- Milagros Rocha M, Victor VM. (2007). Targeting antioxidants to mitochondria and cardiovascular diseases: the effects of mitoquinone. Med Sci Monit 13: RA132–45
- Miyake Y, Shouzu A, Nishikawa M, et al. (1999). Effect of treatment with 3-hydroxy-3-methylglutaryl coenzyme A reductase inhibitors on serum coenzyme Q10 in diabetic patients. Arzneimittelforschung 49:324–9
- Miyamoto S, Kawai A, Higuchi S, et al. (2009). Structural studies of coenzyme Q10 inclusion complex with c-cyclodextrin using chemical analyses and molecular modeling. Chem Bio Informatics J 9:1–11
- Mizuno K, Tanaka M, Nozaki S, et al. (2008). Antifatigue effects of coenzyme Q10 during physical fatigue. Nutrition 24:293–9
- Mortensen SA, Vadhanavikit S, Muratsu K, Folkers K. (1990). Coenzyme Q10: clinical benefits with biochemical correlates suggesting a scientific breakthrough in the management of chronic heart failure. Int J Tissue React 12:155–62
- Murphy MP. (2008). Targeting lipophilic cations to mitochondria. Biochim Biophys Acta 1777:1028–31
- Murphy MP, Smith RA. (2007). Targeting antioxidants to mitochondria by conjugation to lipophilic cations. Annu Rev Pharmacol Toxicol 47:629–56
- Murphy MP, Smith RAJ. (2000). Drug delivery to mitochondria: the key to mitochondrial medicine. Adv Drug Deliv Rev 41:235–50
- Naini AB, Quinzii CM, Navas P, et al. (2006). Genetics of primary CoQ10 deficiency. Curr Genomics 7:343–9
- Nanjwade BK, Kadam VT, Manvi FV. (2013). Formulation and characterization of nanostructured lipid carrier of ubiquinone (coenzyme Q10). J Biomed Nanotechnol 9:450–60
- Nazzal S, Guven N, Reddy IK, Khan MA. (2002a). Preparation and characterization of coenzyme Q10-Eudragit solid dispersion. Drug Dev Ind Pharm 28:49–57
- Nazzal S, Nutan M, Palamakula A, et al. (2002b). Optimization of a self-nanoemulsified tablet dosage form of ubiquinone using response surface methodology: effect of formulation ingredients. Int J Pharm 240:103–14
- Nazzal S, Smalyukh II, Lavrentovich OD, Khan MA. (2002c). Preparation and in vitro characterization of a eutectic based semisolid self-nanoemulsified drug delivery system (SNEDDS) of ubiquinone: mechanism and progress of emulsion formation. Int J Pharm 235:247–65
- Nazzal S, Zaghloul AA, Khan MA. (2002d). Effect of extra-granular microcrystalline cellulose on compaction, surface roughness and in-vitro dissolution of a self-nanoemulsified solid dosage form of ubiquinone. Pharm Technol 26:86–98
- Nehilla BJ, Allen PG, Desai TA. (2008). Surfactant-free, drug-quantum-dot coloaded poly(lactide-co-glycolide) nanoparticles: towards multifunctional nanoparticles. ACS Nano 2:538–44
- Nepal PR, Han HK, Choi HK. (2010). Enhancement of solubility and dissolution of coenzyme Q10 using solid dispersion formulation. Int J Pharm 383:147–53
- New RRC, Kirby CJ. (1999). Solubilisation aids. US Patent No 5968549
- Nishimura K, Higashi T, Yoshimatsu A, et al. (2008). Pseudorotaxane-like supramolecular complex of coenzyme Q10 with gamma-cyclodextrin formed by solubility method. Chem Pharm Bull 56:701–6
- Nucci C, Tartaglione R, Cerulli A, et al. (2007). Retinal damage caused by high intraocular pressure-induced transient ischemia is prevented by coenzyme Q10 in rat. Int Rev Neurobiol 82:397–406
- Ochiai A, Itagaki S, Kurokawa T, et al. (2007). Improvement in intestinal coenzyme q10 absorption by food intake. Yakugaku Zasshi 127:1251–4
- Onoue S, Uchida A, Kuriyama K, et al. (2012). Novel solid self-emulsifying drug delivery system of coenzyme Q(1)(0) with improved photochemical and pharmacokinetic behaviors. Eur J Pharm Sci 46:492–9
- Ooya T, Yui N. (1999). Polyrotaxanes: synthesis, structure, and potential in drug delivery. Crit Rev Ther Drug Carrier Syst 16:289–330
- Palamakula A. (2004a). Biopharmaceutical classification and development of limonene-based self-nanoemulsified capsule dosage form of coenzyme Q10. Texas Tech University Sciences Center, PhD Dissertation, 80–83
- Palamakula A. (2004b). Biopharmaceutical classification and development of limonene-based self-nanoemulsified capsule dosage form of coenzyme Q10. Texas Tech University Sciences Center, PhD Dissertation, 55–79
- Palamakula A, Khan MA. (2004). Evaluation of cytotoxicity of oils used in coenzyme Q10 Self-emulsifying Drug Delivery Systems (SEDDS). Int J Pharm 273:63–73
- Palamakula A, Nutan MT, Khan MA. (2004). Response surface methodology for optimization and characterization of limonene-based coenzyme Q10 self-nanoemulsified capsule dosage form. AAPS PharmSciTech 5:e66
- Palamakula A, Soliman M, Khan MM. (2005). Regional permeability of coenzyme Q10 in isolated rat gastrointestinal tracts. Pharmazie 60:212–14
- Palazzoni G, Pucello D, Littarru GP, et al. (1997). Coenzyme Q10 and colorectal neoplasms in aged patients. Rays 22:73–6
- Pepe S, Marasco SF, Haas SJ, et al. (2007). Coenzyme Q10 in cardiovascular disease. Mitochondrion 7:S154–67
- Persson EM, Gustafsson AS, Carlsson AS, et al. (2005). The effects of food on the dissolution of poorly soluble drugs in human and in model small intestinal fluids. Pharm Res 22:2141–51
- Porteous CM, Logan A, Evans C, et al. (2010). Rapid uptake of lipophilic triphenylphosphonium cations by mitochondria in vivo following intravenous injection: implications for mitochondria-specific therapies and probes. Biochim Biophys Acta 1800:1009–17
- Porter CJH, Charman WN. (1997). Uptake of drugs into the intestinal lymphatics after oral administration. Adv Drug Deliv Rev 25:71–89
- Prosek M, Butinar J, Lukanc B, et al. (2008). Bioavailability of water-soluble CoQ10 in beagle dogs. J Pharm Biomed Anal 47:918–22
- Prosek M, Smidovnik A, Fir M, et al. (2005). Water-soluble coenzyme Q10 in inclusion complex with beta-cyclodextrin, process of preparing, and use thereof. PCT Patent Application No. PCT/SI2005/000013 (WO 2005/111224)
- Quinzii CM, Dimauro S, Hirano M. (2007). Human coenzyme Q10 deficiency. Neurochem Res 32:723–7
- Quinzii CM, Hirano M. (2010). Coenzyme Q and mitochondrial disease. Dev Disabil Res Rev 16:183–8
- Ratnam DV, Chandraiah G, Meena AK, et al. (2009). The co-encapsulated antioxidant nanoparticles of ellagic acid and coenzyme Q10 ameliorates hyperlipidemia in high fat diet fed rats. J Nanosci Nanotechnol 9:6741–6
- Rosenberg J, Breitenbach J. (2004). Stable dosage forms containing Ubiquinones. US Patent Publication No. 2004/0014817A1
- Russo R, Cavaliere F, Rombola L, et al. (2008). Rational basis for the development of coenzyme Q10 as a neurotherapeutic agent for retinal protection. Prog Brain Res 173:575–82
- Samartsev VN, Simonyan RA, Markova OV, et al. (2000). Comparative study on uncoupling effects of laurate and lauryl sulfate on rat liver and skeletal muscle mitochondria. Biochim Biophys Acta 1459:179–90
- Sato Y, Mutoh H, Suzuki M, et al. (2013). Emulsification using highly hydrophilic surfactants improves the absorption of orally administered coenzyme q10. Biol Pharm Bull 36:2012–17
- Schulz C, Obermuller-Jevic UC, Hasselwander O, et al. (2006). Comparison of the relative bioavailability of different coenzyme Q10 formulations with a novel solubilizate (Solu Q10). Int J Food Sci Nutr 57:546–55
- Severina II, Vyssokikh MY, Pustovidko AV, et al. (2007). Effects of lipophilic dications on planar bilayer phospholipid membrane and mitochondria. Biochim Biophys Acta 1767:1164–8
- Sharma N, Baldi A. (2014). Exploring versatile applications of cyclodextrins: an overview. Drug Deliv 22:1–19
- Sharma S, Sahni JK, Ali J, Baboota S. (2014). Effect of high-pressure homogenization on formulation of TPGS loaded nanoemulsion of rutin – pharmacodynamic and antioxidant studies. Drug Deliv [Epub ahead of Print]
- Sharma A, Soliman GM, Al-Hajaj N, et al. (2012). Design and evaluation of multifunctional nanocarriers for selective delivery of coenzyme Q10 to mitochondria. Biomacromolecules 13:239–52
- Shults CW, Flint Beal M, Song D, Fontaine D. (2004). Pilot trial of high dosages of coenzyme Q10 in patients with Parkinson’s disease. Exp Neurol 188:491–4
- Shults CW, Oakes D, Kieburtz K, et al. (2002). Effects of coenzyme Q10 in early Parkinson disease: evidence of slowing of the functional decline. Arch Neurol 59:1541–50
- Sikorska M, Borowy-Borowski H, Zurakowski B, Walker PR. (2003). Derivatised alpha-tocopherol as a CoQ10 carrier in a novel water-soluble formulation. Biofactors 18:173–83
- Singh U, Devaraj S, Jialal I. (2007). Coenzyme Q10 supplementation and heart failure. Nutr Rev 65:286–93
- Singh RB, Niaz MA, Kumar A, et al. (2005). Effect on absorption and oxidative stress of different oral Coenzyme Q10 dosages and intake strategy in healthy men. Biofactors 25:219–24
- Singh G, Pai RS. (2014). Trans-resveratrol self-nano-emulsifying drug delivery system (SNEDDS) with enhanced bioavailability potential: optimization, pharmacokinetics and in situ single pass intestinal perfusion (SPIP) studies. Drug Deliv [Epub ahead of Print]
- Skulachev VP. (2005). How to clean the dirtiest place in the cell: cationic antioxidants as intramitochondrial ROS scavengers. IUBMB Life 57:305–10
- Smith RA, Hartley RC, Murphy MP. (2011). Mitochondria-targeted small molecule therapeutics and probes. Antioxid Redox Signal 15:3021–38
- Smith RA, Porteous CM, Coulter CV, Murphy MP. (1999). Selective targeting of an antioxidant to mitochondria. Eur J Biochem 263:709–16
- Soja AM, Mortensen SA. (1997). Coenzyme Q10 treatment of congestive heart failure: Results of meta-analyses of controlled clinical trials. Mol Aspects Med 159:7302–8
- Spindler M, Flint Beal M, Henchcliffe C. (2009). Coenzyme Q10 effects in neurodegenerative disease. Neuropsychiatr Dis Treat 5:597–610
- Srinivasan K. (2007). Black pepper and its pungent principle-piperine: a review of diverse physiological effects. Crit Rev Food Sci Nutr 47:735–48
- Stocker R, Keaney JF Jr. (2004). Role of oxidative modifications in atherosclerosis. Physiol Rev 84:1381–478
- Stojkovic M, Westesen K, Zakhartchenko V, et al. (1999). Coenzyme Q(10) in submicron-sized dispersion improves development, hatching, cell proliferation, and adenosine triphosphate content of in vitro-produced bovine embryos. Biol Reprod 61:541–7
- Storch A. (2007). Coenzyme Q 10 in Parkinson’s disease. Symptomatic or neuroprotective effects? Koenzym Q 10 beim morbus Parkinson. Symptomatische Oder Neuroprotektive Effekte 78:1378–82
- Sugino K, Dohi K, Yamada K, Kawasaki T. (1989). Changes in the levels of endogenous antioxidants in the liver of mice with experimental endotoxemia and the protective effects of the antioxidants. Surgery 105:200–6
- Suzuki T, Nozawa T, Sobajima M, et al. (2008). Atorvastatin-induced changes in plasma coenzyme q10 and brain natriuretic peptide in patients with coronary artery disease. Int Heart J 49:423–33
- Swarnakar NK, Jain AK, Singh RP, et al. (2011). Oral bioavailability, therapeutic efficacy and reactive oxygen species scavenging properties of coenzyme Q10-loaded polymeric nanoparticles. Biomaterials 32:6860–74
- Swenson ES, Curatolo WJ. (1992). Means to enhance penetration. Adv Drug Del Rev 8:39–42
- Tang B, Cheng G, Gu JC, Xu CH. (2008). Development of solid self-emulsifying drug delivery systems: preparation techniques and dosage forms. Drug Discov Today 13:606–12
- Tang PH, Miles MV, Degrauw A, et al. (2001). HPLC analysis of reduced and oxidized coenzyme Q(10) in human plasma. Clin Chem 47:256–65
- Tarr BD, Yalkowsky SH. (1989). Enhanced intestinal absorption of cyclosporine in rats through the reduction of emulsion of droplets size. Pharm Res 6:40–3
- Terao K, Nakata D, Fukumi H, et al. (2006). Enhancement of oral bioavailability of coenzyme Q10 by complexation with c-cyclodextrin in healthy adults. Nutr Res 26:503–8
- Thanatuksorn P, Kawai K, Hayakawa M, et al. (2009). Improvement of the oral bioavailability of coenzyme Q10 by emulsification with fats and emulsifiers used in the food industry. LWT Food Sci Technol 42:385–90
- Trevisson E, Dimauro S, Navas P, Salviati L. (2011). Coenzyme Q deficiency in muscle. Curr Opin Neurol 24:449–56
- Uekaji Y, Nakata D, Shiga H, et al. (2010). Formation of CoQ10 reduced form by mixing CoQ10 oxidized form a-CD complex and vitamin C in powder. J Incl Phenom Macrocycl Chem 70:447–51
- Ullmann U, Metzner J, Schulz C, et al. (2005). A new Coenzyme Q10 tablet-grade formulation (all-Q) is bioequivalent to Q-Gel and both have better bioavailability properties than Q-SorB. J Med Food 8:397–9
- Venkatesh S, Durga KD, Padmavathi Y, et al. (2011). Influence of piperine on ibuprofen induced antinociception and its pharmacokinetics. Arzneimittelforschung 61:506–9
- Verma DD, Hartner WC, Thakkar V, et al. (2007). Protective effect of coenzyme Q10-loaded liposomes on the myocardium in rabbits with an acute experimental myocardial infarction. Pharm Res 24:2131–7
- Villalba JM, Parrado C, Santos-Gonzalez M, Alcain FJ. (2010). Therapeutic use of coenzyme Q10 and coenzyme Q10-related compounds and formulations. Exp Opin Invest Drugs 19:535–54
- Wadsworth TL, Bishop JA, Pappu AS, et al. (2008). Evaluation of coenzyme Q as an antioxidant strategy for Alzheimer’s disease. J Alzheimers Dis 14:225–34
- Wajda R, Zirkel J, Schaffer T. (2007). Increase of bioavailability of coenzyme Q(10) and vitamin E. J Med Food 10:731–4
- Wang J, Wang H, Zhou X, et al. (2012). Physicochemical characterization, photo-stability and cytotoxicity of coenzyme Q10-loading nanostructured lipid carrier. J Nanosci Nanotechnol 12:2136–48
- Wassvik CM, Holmen AG, Draheim R, et al. (2008). Molecular characteristics for solid-state limited solubility. J Med Chem 51:3035–9
- Wenz G, Han BH, Muller A. (2006). Cyclodextrin rotaxanes and polyrotaxanes. Chem Rev 106:782–817
- Wilkinson EG, Arnold RM, Folkers K, et al. (1975). Bioenergetics in clinical medicine. II. Adjunctive treatment with coenzyme Q in periodontal therapy. Res Commun Chem Pathol Pharmacol 12:111–23
- Wynn RL. (2010). The effects of CoQ10 supplements on patients taking statin drugs. Gen Dent 58:168–70
- Xia S, Xu S, Zhang X. (2006). Optimization in the preparation of coenzyme Q10 nanoliposomes. J Agric Food Chem 54:6358–66
- Xia S, Xu S, Zhang X, et al. (2009). Nanoliposomes mediate coenzyme Q10 transport and accumulation across human intestinal Caco-2 cell monolayer. J Agric Food Chem 57:7989–96
- Xia S, Xu S, Zhang X, Zhong F. (2007). Effect of coenzyme Q(10) incorporation on the characteristics of nanoliposomes. J Phys Chem B 111:2200–7
- Yamamoto Y, Yamashita S, Fujisawa A, et al. (1998). Oxidative stress in patients with hepatitis, cirrhosis, and hepatoma evaluated by plasma antioxidants. Biochem Biophys Res Commun 247:166–70
- Yamashita S, Yamamoto Y. (1997). Simultaneous detection of ubiquinol and ubiquinone in human plasma as a marker of oxidative stress. Anal Biochem 250:66–73
- Yang C, Li H, Wang X, Li J. (2009). Cationic supramolecules consisting of oligoethylenimine-grafted alpha-cyclodextrins threaded on poly(ethylene oxide) for gene delivery. J Biomed Mater Res A 89:13–23
- Yang C, Wang X, Li H, et al. (2007). Synthesis and characterization of polyrotaxanes consisting of cationic alpha-cyclodextrins threaded on poly[(ethylene oxide)-ran-(propylene oxide)] as gene carriers. Biomacromolecules 8:3365–74
- Zaki NM. (2012). Site-specific delivery of the nutraceutical CoQ10 for periodontal therapy. Int J Pharm Pharm Sci 4:717–23
- Zaki NM. (2014a). Progress and Problems in Nutraceuticals Delivery (Expert Review). J Bioequiv Availab 6:075–077
- Zaki NM. (2014b). Augmented cytotoxicity of hydroxycamptothecin-loaded nanoparticles in lung and colon cancer cells by chemosensitizing pharmaceutical excipients. Drug Deliv 21:265–75
- Zaki NM, Albarraq A, Hafez MM. (2013). Cytotoxicity of pharmaceutically optimized nanometric systems of a chemotherapeutic drug on breast and liver tumor cells. Int J Pharm Pharm Sci 5:161–8
- Zaki NM, Artursson P, Bergström CAS. (2010). A modified physiological BCS for prediction of intestinal absorption in drug discovery. Mol Pharm 7:1478–87
- Zhou H, Zhang J, Jin Q, et al. (2013a). Targeting of coenzyme Q10 via d-alpha-tocopheryl polyethylene glycol 1000 succinate-based nanoemulsion to the heart. Mater Lett 109:20–2
- Zhou H, Zhang J, Long Y, et al. (2013b). Improvement of the oral bioavailability of coenzyme Q10 with lecithin nanocapsules. J Nanosci Nanotechnol 13:706–10
- Zlatohlavek L, Vrablik M, Grauova B, et al. (2012). The effect of coenzyme Q10 in statin myopathy. Neuro Endocrinol Lett 33:98–101
- Žmitek J, Pravst I, Žmitek K, Barišić N. (2011). Coenzyme Q10: Usage and pharmaceutical preparations. Koenzim Q10: Farmaceutski Oblici i Njihova Primjena 67:87–100
- Zmitek J, Smidovnik A, Fir M, et al. (2008). Relative bioavailability of two forms of a novel water-soluble coenzyme Q10. Ann Nutr Metab 52:281–7
- Žmitek J, Žmitek K. (2009). Coenzyme Q10 as a dietary supplement and drug. Koencim Q10 Kot Prehransko Dopolnilo in Zdravilo 60:150–7