ABSTRACT
Gelation behaviors of konjac glucomannan (KGM) samples processed via microwave or water bath heating were studied. The microwave-heated gels had significantly better viscoelasticity and stronger KGM molecular chain interactions than water-bath-heated gels. Scanning electron microscopy analysis of the KGM gels revealed a concentrated, uniform network microstructure for the microwave-heated gels and a dense layered structure for the water-bath-heated gels. Microwave heating promotes hydrogen bonding while preserving the molecular chain. Hence, it can be used to improve the gelation properties and micromorphology of KGM without damaging its structure.
Introduction
Konjac glucomannan (KGM), a renewable natural high-molecular-weight (200–2000 kDa) water-soluble neutral polysaccharide, is the main component of the tuber obtained from Amorphophallus konjac K. Koch.[Citation1] Konjac flour, extracted from the corm of this species, is native to Asian countries such as China, Japan, and Thailand. It has a long history of being used as a food in Asia and is used in the Far Eastern cuisine to make noodles, tofu, and various snacks.[Citation2] As a food additive, KGM acts as an emulsifier, a thickener, a meat binder, a gelation enhancer, etc., in a number of food-related applications (e.g., as a gelation enhancer in food-related applications). Example functions include gelation enhancement in low-quality surimi[Citation3,Citation4] and egg white gel[Citation5] and as a fat replacement in meat products[Citation6,Citation7] and mayonnaise.[Citation8] Several products made almost exclusively of KGM are currently available on the market, including noodles and other pasta-like items. As these KGM-based products contain very few calories, they are regarded as weight-control foods.[Citation9]
KGM is mainly composed of a main chain of β-1.4-linked d-glucosyl and d-mannosyl residues as main chain with branching β-1.4-glucosyl units.[Citation10–Citation13] The main chain of KGM is partially acetylated (~5–10% acetylation).[Citation14] It is well known that the removal of acetyl groups from the molecular chains of KGM by adding an alkaline coagulant with heating results in the formation of a thermo-irreversible gel.[Citation15,Citation16] However, the gelation mechanism is still under investigation since the early report by Maekaji[Citation17], which concluded that, upon losing their acetyl groups, KGM molecules aggregate through linkages such as hydrogen bonding and forming a network structure that ultimately leads to gel formation. Gels whose helices primarily contain hydrogen-bonded crosslinks, such as gelatin and agarose gel, melt with increasing the temperature. In contrast, when KGM gels are heated, they produce thermo-irreversible gels, and the elastic modulus increases.[Citation18] A molecular-level description of the gelation mechanism of KGM has been presented elsewhere, based on NMR relaxometry, which illustrated that alkali addition plays an important solubilizing role in addition to facilitating the deacetylation of the chain;[Citation19] however, Williams et al. did not consider the molecular forces of gelation. Further studies were conducted on the gelation mechanism of KGM made it clear that hydrophobic interactions play an important role.[Citation20,Citation21] However, the exact ratio of the contributions from hydrogen bonding and hydrophobic interactions to KGM gelation has never been determined. Numerous studies have been conducted on the junction zones in gels, showing that such zones provide many valuable clues regarding both the roles of acetyl groups and the gelation mechanism of KGM.[Citation22,Citation23]
Although the gelation of polysaccharides present in the KGM molecular chain has been investigated, the effect of different thermal processing methods on gelation has never been studied. According to related researches, microwave heating results in the alteration of the polysaccharides through the unwinding of the molecular chain; and it also affects the gel strength of the polysaccharides.[Citation24,Citation25] Microwave heating is significantly quicker than other available heating technologies; moreover, it heats food volumetrically, in contrast to the conductive heating used in traditional techniques.[Citation26–Citation28] This means that part of the applied microwave energy is converted to heat wherever the microwaves interact with food, leading to consistent heating throughout the material in a more rapid process that is less likely to cause damage, thus improving the quality of the heated product; this interaction is enhanced by the high dielectric character of certain foods.[Citation29,Citation30] In the present study, native KGM was treated with alkali in distilled water to obtain completely deacetylated KGM samples. The primary objective of this research was to gain further insight into the gelation networks formed by different thermal processing methods.
Materials and methods
Materials
KGM (purity > 85%) was obtained from Sheli (Yantai, China) and used as received. All chemicals used were of analytical grade and were purchased from Bodi Chemical Co. (Tianjin, China).
Sample preparation
Preparation of sol: To prepare the KGM solution, the method developed by Li et al. (2014) was used. Na2CO3 powder (0.36 g) was first dissolved in distilled water (100 mL). Then, a 3% (w/w) KGM solution was prepared by suspending KGM (3.0 g) powder in the Na2CO3 solution using a magnetic stirrer (Shanghai Huxi Analysis Instrument Factory Co. 90-2, China) and completely swelling the resulting solution at 40°C in a water bath for 4 h to prepare totally deacetylated KGM gels.
Gel formation: Solutions were poured into identical glass containers and heated to 90°C using microwave irradiation (Questron Technologies Corp. QPro-M, Canada) or a water bath. The microwave heating method was applied using three power outputs with different heating times: 300 W/5 min; 300 W/10 min; 300 W/15 min; 400 W/5 min; 400 W/10 min; 400 W/15 min; 500 W/5 min; and 500 W/10 min. For the water bath heating method, the heating times varied from 30 to 110 min with 20 min intervals, i.e., 30 min, 50 min, 70 min, 90 min, and 110 min. Finally, nine samples (microwave: 300 W/5 min; 300 W/10 min; 300 W/15 min; 400 W/5 min; 400 W/10 min; 400 W/15 min; 500 W/5 min; 500 W/10 min; water bath, 1 h) were selected based on their textural properties. All the gel samples were cooled to room temperature (25 ± 2°C) and allowed to equilibrate for at least 2 h before scanning electron microscopy (SEM) imaging, texture analysis, rheometry, and hydrogen bonding measurements. Each analytical measurement was performed in triplicate.
Characterization methods
Texture analysis: Texture analysis of each KGM gel was carried out using a TMS-PRO texture analyzer (Food Technology Co., USA). The breaking force is defined as the force required (in g) to break the gel, and the deformation is the distance traveled by the probe (in cm) from the surface of the gel to the point of breakage. The gels were equilibrated at room temperature (25°C) before analysis. Cylindrical samples (2.5 cm in length) were prepared and placed in the texture analyzer, which was equipped with a spherical plunger (5 mm diameter, 60 mm/min depression speed) selected based on a previous work.[Citation31] The breaking force was measured and read on the force versus deformation curve as the value of the first force peak. Using the method developed by Herranz, Tovar, Solo-de-Zaldívar, & Borderias, the depression (as deformation) that occurred when the gel sample lost its strength and ruptured was recorded. Each measurement is reported as the mean value of three independent experiments (n = 3).
Rheological measurements: Dynamic viscoelastic measurements were performed with an MCR101 rheometer (Anton Paar, Austria). A parallel plate with 50 mm diameter and 1.0 mm gap was used to measure the frequency dependence of the storage (G′) and loss (G″) moduli. The measurement temperature was controlled at 25 ± 1°C using a universal temperature controller (CH1006, SHP, China). For each test, the samples were cut into uniform 1-mm-thick slices and allowed to equilibrate at the measurement temperature for 5 min before data acquisition. For oscillatory tests, a glass hood and silicon oil were used to prevent evaporation during measurements.
To determine the proper amplitude (γ) of the dynamic frequency sweep measurements, a linear viscoelastic (LVE) region sweep was first performed. Subsequently, the frequency sweep measurements were carried over the 1.0–10.0 Hz range with 1% strain (within the LVE range) at the specified temperature, i.e., 25°C. Each measurement is reported as the mean value of three independent experiments (n = 3).
SEM imaging: The microstructures of the KGM gels were analyzed by SEM.[Citation32,Citation33] KGM gels with 2–3 mm thickness were fixed with a 3% glutaraldehyde solution for 4 h. The samples were then rinsed with distilled water for 1 h before dehydrating them in a gradient ethanol series of 50%, 70%, 80%, 90%, and 100% (v/v). After drying, the samples were mounted on a bronze stub and sputter-coated with gold. The specimens were observed using SEM (JEOLJSM-5800 LV, Tokyo, Japan) at an acceleration voltage of 15 kV.
Hydrogen bonding measurement: The hydrogen bonding measurements were performed with an ultraviolet-visible (UV-vis) spectrophotometer (UV-2550, SHIMADZU, Japan). KGM gel (1 g) was cut into identical small cubes and added to 60 mL aqueous solutions of guanidine hydrochloride, which is a hydrogen-bond-cracking agent. The mass fractions of guanidine hydrochloride used were 1.0%, 3.0%, 5.0%, 7.0%, and 9.0%. Then, the gels were hydrolyzed in a water bath at a constant temperature (37°C) for 15 h followed by filtration to obtain the hydrolysates. The turbidity of the hydrolysates, reflecting the degree of dissociation, was measured using the UV-vis spectrophotometer and with the same concentration of guanidine hydrochloride as in the blank. The maximum absorption peak was measured by obtaining full UV-vis scans, and the turbidity of the hydrolysates was measured at the maximum absorption peak. Each measurement is reported as the mean value of three independent experiments (n = 3).
Fourier transform infrared (FT-IR) spectroscopy: The various KGM gel samples produced by different heating methods were analyzed over the 400–4000 cm−1 range using FT-IR spectroscopy (Thermo, Nicolet iN10, USA). The samples were ground, mixed with KBr, and scanned against an air background. The data were analyzed using the Omnic 3.2 software package.
Statistical analysis
All experimental data were obtained as mean ± standard deviation (SD). Differences between the means of the data were compared by the least significant difference (LSD) (p < 0.05), which was calculated using the Statistical Analysis System (SAS Institute, Inc., Cary, NC).
Results and discussion
Effect of different heating methods on the textural properties of the gels
As shown in , in general, the KGM gels processed by microwave heating (MKGM) exhibit higher breaking force () and deformation () values than those produced by water bath heating. Both the breaking force and deformation of the KGM gel processed by water bath heating (WBKGM) first increased and then decreased with prolonged heating. There are significant differences in the breaking force and deformation between the MKGM and WBKGM samples. Clearly, the breaking force of the MKGM processed at 300 W continuously increases on extension of the heating time, with maximum deformation at 10 min. However, the breaking force and deformation of the MKGM processed at 500 W increased first and then decreased with prolonged heating. This means that heating at a higher power output for a long time may damage the products. Moreover, the surfaces of the MKGM gels are partially wrinkled owing to the loss of moisture, especially at high power outputs, which indicates that although higher power outputs shorten the heating time, they might be detrimental to product quality.
Figure 1. Breaking force (a) and deformation (b) of the KGM gels treated with different heating methods.
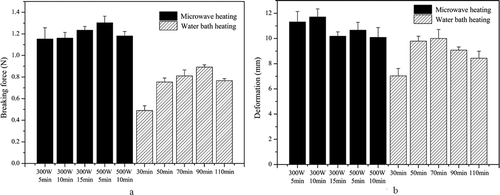
Overall, MKGM gels heated for 5 min at 500 W have significantly better textural properties and the maximum extent of gel strength. We speculate that KGM molecules are stretched by the generated water vapor, offering more sites for intermolecular cross-linking and resulting in the restructured network. The energy transfer under microwave radiation (MKGM samples) is associated with a long mean free path for the radiant energy carriers, in contrast with the short mean free path for heat conduction (WBKGM).[Citation34–Citation36] The energy carriers in microwave heating are photons; however, in heat conduction, the energy carriers are normally electrons moving in a lattice-vibration environment. Owing to the thermal effects of the microwave radiation energy on the polysaccharide molecules, further polymerization of the polysaccharides occurs, resulting in more concentrated intermolecular cross-linking, which might increase the breaking force and reduce the deformation.
In spite of such large strain deformation, mechanical tests provide information that correlates more with the sensory perception and handing properties of food products.[Citation37] Small deformation oscillatory measurements have been considered as necessary and useful tools to study the network structure of foods, especially that of food gels that present complex viscoelastic behavior.[Citation38] Thus, we considered it necessary to conduct a thorough rheological study to identify the properties of these gel networks.
Effects of different heating methods on the dynamic rheology measurements of KGM samples
A frequency sweep test was carried out to study the viscoelastic properties and interactions between KGM molecular chains. During the elastic deformation of a viscoelastic material, G′ denotes the stored energy and G″ denotes the lost energy. Both G′ and G″ can be used to investigate the effects of the heating methods on the KGM gels. The performance of the gels processed by microwave and water bath heating was compared. In the first step, the linear viscoelastic range (LVE) for all the polysaccharide gels was investigated. Within this range, the viscoelastic moduli are independent of the stress. As the applied stress is increased, the bonds holding the network together begin to rupture. shows the frequency dependence of G′ for 3.0 wt% aqueous solutions of KGM obtained using different heating methods and heating times. For all samples which characterize the amplitude gamma at 1%, G′ shows almost the same trend. The value of G′ increases less with increasing angular frequency. For every sample, G′ (elastic component) is greater than G″ (viscous component), indicating the presence of a network structure, and both parameters exhibited predominantly solid-like behavior over the experimental frequency range. Notably, the viscoelastic moduli, especially G′, of the WBKGM gels have extremely low values over the entire angular frequency range, indicating the weaker structure of the WBKGM gels. In contrast, the MKGM gels heated at 300 W for 10 min and 400 W for 10 min exhibited relatively higher G′, which means that these gels have better viscoelasticity and stronger interactions between the KGM molecular chains.
Figure 2. Mechanical spectra of storage modulus (G’), loss modulus (G’’), and loss tangent (tanδ) for 3% KGM gels at room temperature (25°C).
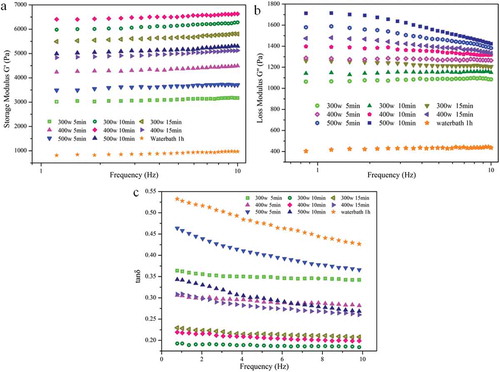
Subsequently, the products obtained using different microwave heating conditions, including different power outputs and heating times, were compared. As can be seen from , the samples heated at 300 W for 10 min and 400 W for 10 min showed higher G′ and relatively lower G′′, indicating that an optimum condition for producing more elastic gels existed. This shows that both the output power and heating time are important factors in improving the elastic properties of MKGM products. In addition, G″ shows a slightly different trend from that of G′: the change in the former is much more regular than that of the latter. The value of G″ increases with prolonged heating time at the same output and is the highest the power output is 500 W.
These results are more clearly revealed by the tan δ values (Fig. 2c), which is defined as the ratio of G″ and G′, which reflect the viscoelastic behavior of the gels.[Citation39] The sample heated at 300 W for 10 min has a low tan δ, indicating good elastic properties. In comparison, the tan δ value of the sample heated at 500 W for 5 min is higher, indicating a less dense cross-linked gel network that becomes vulnerable to breakdown during shearing at low frequencies. Moreover, we suspected that heating by microwave is a faster process, which could reduce the connectivity level in the gel network, probably resulting in a less time-stable polymeric network.
Microwave heating indeed improves the rheological behavior of KGM gels, especially in terms of their elastic properties. We conjectured that volumetric microwave heating could stretch the KGM chain by water vapor diffusion resulting in a more obvious expansion of the network structure, in contrast to the conductive heating of a water bath. Therefore, the microwave-heated gels show stored G′ and G″ values that are based on a more homogeneous and elastic network structure. However, the processing conditions should be controlled because overheating may cause irreversible damage to the product.
SEM imaging
The SEM images of the cross-sections of the KGM gels treated by different heating methods are shown in . The photographs show that the microstructure of the MKGM gels is significantly different from that of the WBKGM gels. Clear network structure formation, which allows the KGM gels to maintain certain elastic characteristics, only occurs in the MKGM gels, whereas the WBKGM gels have only a layered microstructures. We suspect that, compared with MKGM gels, WBKGM may not easily form a clear network structure owing to the lower degree of molecular chain stretching. The MKGM gels showed a concentrated and uniform network structure with many sizable holes and strong frames. There are no significant differences in the network structure among the KGM gels prepared under different microwave conditions. This could be explained by the fact that KGM formed gels more easily under microwave heating, and the elastic characteristics of the gels could also be improved under appropriate conditions (e.g., suitable heating time and power output). These results are in agreement with the textural properties. From the images, it can be speculated that there are two main causes for this phenomenon. First, the temperature rise during microwave heating is significantly faster than that in water bath heating. Second, microwave energy, in the form of electromagnetic waves, is absorbed by the KGM samples and converted to effective heat. In other words, compared with the conductive heating using a water bath, microwave heating leads to volumetric heating in the KGM samples, inducing network formation in the gels. We speculate that microwave energy under the appropriate conditions leads to the vibration of the polysaccharide and water molecules, resulting in more intermolecular cross-linking between them, thus forming the network structure shown in the images. However, it might be difficult to achieve this effect using conductive heating. This difference in the heating mechanism suggests that microwave heating is a more rapid and effective heat processing method for KGM than water bath heating.
Hydrogen bonding measurements
In the UV-vis scan, the maximum absorption peak of the KGM gel is observed at 256 nm. shows the turbidity measurements of the gel hydrolysates, which were treated with different concentrations of guanidine hydrochloride solution. Disrupting the hydrogen bonds will increase the solute quantity in the solution, resulting in higher turbidity.[Citation40] The trends observed in the turbidity of MKGM processed at 300 W and 500 W were higher than those of WBKGM. In other words, the gels subjected to microwave heating were more turbid, indicating that more hydrogen bonds had been destroyed. Thus, we suspect that the molecular interactions in the MKGM gels are much more extensive, in accordance with the former analyses.
Fourier transform infrared (FT-IR) spectroscopy measurements
shows the FT-IR spectra obtained for the powders of the KGM gel samples produced by different heating methods. The peaks at 2919 and 1400 cm−1 are assigned to -CH2-stretching vibrations. The intense peak at 1643 cm−1 is attributed to the in-plane deformation of water.[Citation41,Citation42] In addition, the absorption at approximately 3400 cm−1 corresponds to the polysaccharide hydroxyl stretching vibrations, whereas that at approximately 1734 cm−1 is due to the carbonyl stretching vibration of the polysaccharide acetyl groups.
This characteristic native KGM absorption bands at approximately 1734 cm−1, shown in , is indicative of the acetyl groups on the molecular chains. However, this peak disappears after processing because all the samples are deacetylated, as reported previously.[Citation43] The samples produced by microwave heating and water bath heating show the same stretching vibrations, indicating that the backbones and functional groups of the KGM molecular chains are unchanged by microwave heating.
Conclusion
In the present study, we found that microwave heating plays an important role in the gelation behavior of KGM. The microstructures of the MKGM and WBKGM gels are significantly different: the MKGM gels have a uniform network structure, and the WBKGM gels have a dense layered structure, which explains the superior textural properties resulting from microwave heating. In addition, the MKGM gels have higher storage and loss moduli, indicating that the viscoelastic properties and molecular interactions are improved by the network structure. Hydrogen bonding is stronger in the MKGM gels, although the backbone and groups of the KGM molecular chain are not changed by microwave heating. The better properties and microstructure of the MKGM gels should improve the quality of food products, especially those produced industrially. In addition, the control of the microwave heating conditions is crucial for achieving the desired effects. Importantly, microwave heating can cause the loss of moisture and, hence, should be carried out under controlled conditions.
Funding
This study was supported by the National Natural Science Fund of China (No. 31371791) and by the National Natural Science Funds of China (No. 31571865).
Additional information
Funding
References
- Nishinari, K.; Williams, P.A.; Phillips, G.O. Review of the Physico-chemical Characteristics and Properties of Konjac Mannan. Food Hydrocolloids 1992, 6 (2), 199–222.
- Chua, M.; Baldwin, T.C.; Hocking, T.J.; Chan, K. Traditional Uses and Potential Health Benefits of Amorphophallus konjac K. Koch ex N.E.Br. Journal of Ethnopharmacology 2010, 128 (2), 268–278.
- Iglesias-Otero, M.A.; Borderías, J.; Tovar, C.A. Use of Konjac Glucomannan as Additive to Reinforce the Gels from Low-quality Squid Surimi. Journal of Food Engineering 2010, 101 (3), 281–288.
- Liu, J.; Wang, X.; Ding, Y. Optimization of Adding Konjac Glucomannan to Improve Gel Properties of Low-quality Surimi. Carbohydrate Polymers 2013, 92 (1), 484–489.
- Liu, J.; Zhu, K.; Ye, T.; Wan, S.; Wang, Y.; Wang, D.; Li, B.; Wang, C. Influence of Konjac Glucomannan on Gelling Properties and Water State in Egg White Protein Gel. Food Research International 2013, 51 (2), 437–443.
- Jiménez-Colmenero, F.; Triki, M.; Herrero, A.M.; Rodríguez-Salas, L.; Ruiz-Capillas, C.. Healthy Oil Combination Stabilized in a Konjac Matrix as Pork Fat Replacement in Low-fat, PUFA-enriched, Dry Fermented Sausages. LWT - Food Science and Technology 2013, 51 (1), 158–163.
- Ruiz-Capillas, C.; Triki, M.; Herrero, A.M.; Rodriguez-Salas, L.; Jimenez-Colmenero, F. Konjac Gel as Pork Backfat Replacer in Dry Fermented Sausages: Processing and Quality Characteristics. Meat Science 2012, 92 (2), 144–150.
- Li, J.; Wang, Y.; Jin, W.; Zhou, B.; Li, B. Application of Micronized Konjac Gel for Fat Analogue in Mayonnaise. Food Hydrocolloids 2014, 35, 375–382.
- Marcano, J.; Hernando, I.; & Fiszman, S. In Vitro Measurements of Intragastric Rheological Properties and their Relationships with the Potential Satiating Capacity of Cheese Pies with Konjac Glucomannan. Food Hydrocolloids 2015, 51, 16–22.
- Kato, K.; Matsuda, K. Studies on the Chemical Structure of Konjac Mannan Part I. Isolation and Characterization of Oligosaccharides from the Partial Acid Hydrolyzate of the Mannan. Agricultural and Biological Chemistry 1969, 33 (10), 1446–1453.
- Katsuraya, K.; Okuyama, K.; Hatanaka, K.; Oshima, R.; Sato, T.; Matsuzaki, K. Constitution of Konjac Glucomannan: Chemical Analysis and 13C NMR Spectroscopy. Carbohydrate Polymers 2003, 53 (2), 183–189.
- Lafarge, C.; Journaux, L.; Bonnotte, A.; Lherminier, J.; Lee, J.A.; Baile, P.L.; Cayot, N. Trapping of Carvacrol by Konjac Glucomannan-potato Starch Gels: Stability from Macroscopic to Microscopic Scale, Using Image Processing. Food Hydrocolloids 2017, 66, 216–226.
- Bewley, J.D.; Reid, J.S.G. Biochemistry of Storage Carbohydrates in Green Plants; Academic Press: New York, 1985, pp. 289–304.
- Khanna, S.; Tester, R.F. Influence of Purified Konjac Glucomannan on the Gelatinisation and Retrogradation Properties of Maize and Potato Starches. Food Hydrocolloids 2006, 20 (5), 567–576.
- Chen, J.; Li, J.; & Li, B. Identification of Molecular Driving Forces involved in the Gelation of Konjac Glucomannan: Effect of Degree of Deacetylation on Hydrophobic Association. Carbohydrate Polymers 2011, 86 (2), 865–871.
- Cheng, L.H.; Abd Karim, A.; Norziah, M.H.; Seow, C.C. Modification of the Microstructural and Physical Properties of Konjac Glucomannan-based Films by Alkali and Sodium Carboxymethylcellulose. Food Research International 2002, 35 (9), 829–836.
- Maekaji, K. The Mechanism of Gelation of Konjacmannan. Agricultural and Biological Chemistry 1974, 38, 315–321.
- Tye, R.J. Konjac Flour: Properties and Applications. Food Technology 1991, 45 (3), 82–92.
- Williams, M.A.; Foster, T.J.; Martin, D.R.; Norton, I.T.; Yoshimura, M.; Nishinari, K. A Molecular Description of the Gelation Mechanism of Konjac Mannan. Biomacromolecules 2000, 1 (3), 440–450.
- Herranz, B.; Borderias, A.J.; Solo-de-Zaldívar, B.; Solas, M.T.; Tovar, C.A. Thermostability Analyses of Glucomannan Gels. Concentration Influence. Food Hydrocolloids 2012a, 29 (1), 85–92.
- Herranz, B.; Tovar, C.A.; Solo-de-Zaldívar, B.; Borderias, A.J. Effect of Alkalis on Konjac Glucomannan Gels for use as Potential Gelling Agents in Restructured Seafood Products. Food Hydrocolloids 2012b, 27 (1), 145–153.
- Herranz, B.; Borderias, A.J.; Solas, M.T.; Tovar, C.A. Influence of Measurement Temperature on the Rheological and Microstructural Properties of Glucomannan Gels with different Thermal Histories. Food Research International 2012c, 48 (2), 885–892.
- Solo-de-Zaldívar, B.; Tovar, C.A.; Borderías, A.J.; Herranz, B. Effect of Deacetylation on the Glucomannan Gelation Process for Making Restructured Seafood Products. Food Hydrocolloids 2014, 35, 59–68.
- Ahmad, M.; Gani, A.; Shah, A.; Gani, A.; Masoodi, F.A. Germination and Microwave Processing of Barley (Hordeum vulgare L) changes the Structural and Physicochemical Properties of β-D-glucan & Enhances its Antioxidant Potentialmudasir. Carbohydrate Polymers 2016, 153, 696–702.
- Zhang, W.; Luan, D.; Tang, J.; Sablani, S.S.; Rasco, B.; Lin, H.; Liu, F. Dielectric Properties and other Physical Properties of low-acyl gellan gel as Relevant to Microwave Assisted Pasteurization Process. Journal of Food Engineering 2015, 149, 195–203.
- Kong, F.; Tang, J.; Rasco, B.; Crapo, C. Kinetics of Salmon Quality changes during Thermal Processing. Journal of Food Engineering 2007, 83 (4), 510–520.
- Tang, J.; Liu, F.; Pathak, S.; Eves, G. Apparatus and Method for Heating Objectives with Microwaves. U.S. Patent 7 2006, 119, 313.
- Sahin, S.; Sumnu, G. Effects of Microwave Cooking on Fish Quality. International Journal of Food Properties 2001, 4 (3), 501–512.
- Sosa-Morales, M.E.; Valerio-Junco, L.; López-Malo, A.; García, H.S. Dielectric properties of foods: Reported Data in the 21st Century and their Potential Applications. LWT - Food Science and Technology 2010, 43 (8), 1169–1179.
- Wang, Y.; Tang, J.; Rasco, B.; Wang, S.; Alshami, A.A.; Kong, F. Using Whey Protein Gel as a Model Food to Study Dielectric Heating Properties of Salmon (Oncorhynchus gorbuscha) fillets. LWT - Food Science and Technology 2009, 42 (6), 1174–1178.
- Zhang, T.; Xue, Y.; Li, Z.; Wang, Y.; Xue, C. Effects of Deacetylation of Konjac Glucomannan on Alaska Pollock surimi gels subjected to high-temperature (120°C) treatment. Food Hydrocolloids 2015, 43, 125–131.
- Liu, R.; Zhao, S.M.; Xiong, S.B.; Xie, B.J.; Qin, L.H. Role of Secondary Structures in the Gelation of Porcine Myosin at different pH Values. Meat Science 2008, 80 (3), 632–639.
- Bi, W.; Zhao, W.; Li, D.; Li, X.; Yao, C.; Zhu, Y.; Zhang, Y. Effect of Resistant Starch and Inulin on the Properties of Imitation Mozzarella Cheese. International Journal of Food Properties 2016. 19 (1), 159–171.
- Bhattacharya, M.; Basak, T. A Review on the Susceptor Assisted Microwave Processing of Materials. Energy 2016, 97, 306–338.
- Divekar, M.T.; Karunakaran, C.; Lahlali, R.; Kumar, S.; Chelladurai, V.; Liu, X.; Borondics, F.; Shanmugasundaram, S.; Jayas, D.S. Effect of Microwave Treatment on the Cooking and Macronutrient Qualities of Pulses. International Journal of Food Properties 2016, 20 (2), 409–422.
- Arocas, A.; Sanz, T.; Hernando, M.I.; Fiszman, S.M. Comparing Microwave- and Water Bath-thawed Starch-based Sauces: Infrared Thermography, Rheology and Microstructure. Food Hydrocolloids 2011, 25, 1554–1562.
- Bollaín, C.; Angioloni, A.; Collar, C. Bread Staling Assessment of Enzyme-supplemented Pan Breads by Dynamic and Static Deformation Measurements. European Food Research & Technology 2005, 220 (1), 83–89.
- Romero, A.; Cordobés, F.; Puppo, M.C.; Villanueva, Á.; Pedroche, J.; Guerrero, A. Linear Viscoelasticity and Microstructure of Heat-induced Crayfish Protein Isolate Gels. Food Hydrocolloids 2009, 23 (3), 964–972.
- Long, Z.; Zhao, M.; Sun-Waterhouse, D.; Lin, Q.; Zhao, Q. Effects of Sterilization Conditions and Milk Protein Composition on the Rheological and Whipping Properties of Whipping cream. Food Hydrocolloids 2016, 52, 11–18.
- Gong, J.; Peng, C.; Xing, Z.; Pang, J. Studies on the Interaction Force of Konjac Glucomannan and Soybean Isolate Protein Combination during Gel Formation. Journal of Chinese Institute of Food Science and Technology 2006, 6(5), 64–68.
- Chua, M.; Chan, K.; Hocking, T.J.; Williams, P.A., Perry, C.J.; Baldwin, T.C. Methodologies for the Extraction and Analysis of Konjac Glucomannan from Corms of Amorphophallus konjac K. Koch. Carbohydrate Polymers 2012, 87 (3), 2202–2210.
- Yu, H.; Huang, Y.; Ying, H.; Xiao, C. Preparation and Characterization of a Quaternary Ammonium Derivative of Konjac Glucomannan. Carbohydrate Polymers 2007, 69 (1), 29–40.
- Zhang, H.; Yoshimura, M.; Nishinari, K.; Williams, M.A.K.; Foster, T.J.; Norton, I.T. Gelation Behavior of Konjac Glucomannan with different Molecular Weights. Biopolymers 2001, 59, 38–50.