ABSTRACT
Fresh produce is an essential part of long-term, interspatial nutrition. However, resource constraints including launch mass, system power, and spacecraft cabin volume limit the amount of produce available for crew. Increasing the available oxygen to a plant’s root system stimulates overall growth but typical terrestrial approaches (i.e. sparging) are inappropriate for gas–liquid contacting in a microgravity environment. Terrestrial studies show the use of neutrally buoyant oxygen nanobubbles increases the plant nutritional and edible mass density for a given footprint. Thereby, addressing nutritional gaps in the current food system. This paper briefly evaluates the nutritional requirements for exploration astronauts and assesses the current approach for spaceflight produce provision. Then, presents the perspective opportunity to increase harvest index by implementing nanobubble technologies. Finally, a high-level review of nanobubble integration to enhance spaceflight food production systems and testing necessary to use nanobubbles in spaceflight systems is outlined.
Highlights
Neutrally buoyant nanobubbles supply long duration gas saturation in liquids
Terrestrial hydroponic systems increase harvest density with nanobubbles
Nanobubble stability in microgravity is currently unknown
Introduction
Since the start of human spaceflight in the 1960s, the food provision system has heavily relied on pre-packaged, preserved foods. The Mercury project into the Apollo program used compressed food cubes and tubes of liquified food, appropriate for relatively short flights with limited cabin volume (Dowdy Citation2020). From later Apollo missions to today, thermostabilized, irradiated, or dehydrated food pouches allow crew to eat with spoons after minimal meal prep. Reliably shelf-stable, without refrigeration and easy to transport, these foods are suitable for use within a few years (Douglas et al. Citation2021). The current International Space Station (ISS) food system uses a hybrid approach of fresh and shelf-stable food. When resupply missions launch every three months, a quantity of fresh fruits and vegetables are included, serving the crew for a week (Douglas et al. Citation2020). This approach is sufficient for long-duration spaceflight in Low Earth Orbit (LEO). However, initial assessments of using the ISS food system for exploration missions (long duration missions beyond LEO, approximately 3–5 years) considered it volume and mass prohibitive (Douglas et al. Citation2021). Additionally, key nutrients, like vitamins B1 and C may degrade to inadequate levels over the course of the mission (Cooper et al. Citation2017). Crew health (bone density, immune system) and mission safety (task performance) are reliant on a food system tailored to the requirements of space (Douglas et al. Citation2020).
Bioregenerative food production during a mission is a promising nutritional supplementation approach, offering an increase in available food variety and easily absorbed nutrients (Massa et al. Citation2015). Since 2013, the ISS has grown salad crops (i.e. pick and eat varieties with minimal processing prior to consumption) using the Veggie and Advanced Plant Habitat Growth systems; with astronaut sampling starting in 2015 (Stromberg Citation2015; Massa et al. Citation2017a). Long-duration astronauts reported increased morale and reduced stress resulting from interactions with the plants and watching the growth cycles is an added benefit (2022 personal conversation with M. Vande Hei; Massa et al. Citation2017b). However, adequately covering the nutritional gaps in the current food system for exploration missions will require a larger system footprint or increased produce density (i.e. harvest index) (Kaschubek Citation2021). Increasing harvest index of the current footprint can be accomplished with minimal impact on system volume, mass, and power requirements (Wheeler Citation2003; de Micco et al. Citation2009; Nguyen et al. Citation2023). Terrestrial studies show that increasing available dissolved oxygen to the root system (i.e. via gas bubble sparging) stimulates growth and production for a given footprint (Du et al. Citation2018; Liu et al. Citation2019). However, sparging for gas–liquid contacting is buoyancy-driven and inappropriate for microgravity. Bubble production in microgravity can result in large, disruptive bubbles with unpredictable movement (Arias et al. Citation2009). For this, reducing the size of bubbles down to the nanoscale creates metastable bubbles reliant on Brownian motion for gas–liquid contacting and creates more even dispersion (Yaparatne et al. Citation2022). Terrestrially, nanobubbles (NB) can stay suspended in solution for weeks to months, providing increased gas availability to the surrounding liquid (Meegoda et al. Citation2018; Tan et al. Citation2020; Cerrón-Calle et al. Citation2022). Increasing available dissolved oxygen to roots in microgravity may increase the harvest index to supply sufficient nutrition to astronauts during exploration missions.
This perspective presents the opportunity for NBs to address the need for continuous gas–liquid contacting in spaceflight produce systems by reviewing nutritional considerations for exploration mission astronauts, standard for fresh produce in reduced gravity environments and limitations to spaceflight plant growth, NB for increased harvest density to decrease overall mission resource impact and increase nutritional availability. This paper concludes by outlining the opportunities and the research roadmap to test the reliability of NBs systems in reduced gravity environments.
Addressing nutritional needs of astronauts in long duration missions
The nutritional requirements for long duration spaceflight beyond LEO differ from those supporting long-duration ISS missions. Traveling beyond the protective magnetosphere leaves crew vulnerable to radiation and increases the risk of cellular damage (Kulkarni et al. Citation2022). A multimodal approach, including passive and active shielding, is necessary to minimize the risk of radiation-induced damage (Ewert et al. Citation2022). Nutritionally, increasing the amount of antioxidant vitamins and minerals (folic acid, selenium, cysteine, anthocyanins) as a part of that multimodal approach can help reduce the physiological effects of radiation (Buckey Citation2006). The most potent antioxidants (vitamin A, C, E, β-carotene, B3, and B1) are also the most susceptible to degradation in the spaceflight environment over time (Douglas et al. Citation2021; Watkins et al. Citation2022). In fresh produce, these nutrients are prevalent in leafy greens, tomatoes, peppers, pumpkin, and sunflower seeds (Hodgson Citation2023).
Since 1966, NASA has conducted various plant growth experiments in microgravity. While hydroponic systems are typically the most efficient approach, there were some challenges associated with water containment and impaired root zone aeration in these early studies. However, transportation and containment of traditional soil was launch mass prohibitive (Torres et al. Citation2020). Instead, the genesis of lightweight, permeable media for root support improved moisture accessibility and oxygen distribution across the root bed (Steinberg et al. Citation2002; Ma et al. Citation2023). The Veggie system and Advanced Plant Habitat on the ISS have successfully grown 12 varieties of leafy greens, a radish crop, and a chili pepper crop using a porous substrate (Johnson et al. Citation2021). With optimal temperature, humidity and light intensity, microgravity has not significantly impacted plant evapotranspiration, net photosynthesis, and water use efficiency (Monje et al. Citation2005; Massa et al. Citation2016). While these experiments characterized growth in a spaceflight environment, the next step is to improve the harvest index to fulfill nutritional needs for a volume-restricted spacecraft cabin.
Root restriction may result organically due to smaller allotted footprints, but studies have shown that root restriction in certain species can increase the volume utilization efficiency (VUE). Initial terrestrial trials indicated that with root restriction, bell pepper plants reduced overall biomass production (smaller resulting plant size) but sustained fruit production when compared to plants with larger root volumes; thereby suggesting altered resource partitioning away from plant growth to fruit production (Graham and Wheeler Citation2016). However, this is reliant on adequate nutrient, water availability, and aeration at the roots, as these studies also recorded harmful effects associated with decreased oxygen availability due to root zone flooding (Levine et al. Citation2008). Increasing the dissolved oxygen in the irrigation water may address concerns about root zone flooding, enabling accommodation changes in flow pattern. Improved oxygen availability to reduce overall plant size may expand the list of salad and ‘pick-and-eat’ crops acceptable for spaceflight. In microgravity, sustaining dissolved oxygen saturation in water must be accomplished through gravity-independent methods (Ellery Citation2021). The industry standard includes diffusion-driven, gas-permeable membranes but their transfer rates are sensitive to liquid-side contamination (Guo et al. Citation2012). Delivering gas in the form of nano-bubbles (NB) may provide the benefits of gas–liquid contacting akin to terrestrial gas sparging but be small enough to be gravity independent (Cerrón-Calle et al. Citation2022). The NB can be understood as nano-sized gas pockets in aqueous solutions or ultra-fine bubbles of nanometric diameter (usually below 300 nm). With the introduction of these NBs, hydroponics may be a viable growth method again as part of life-support systems.
Hydroponics: a terrestrial technology with spatial future
Hydroponic crop production is a well-known agronomic technique that involves growing plants using a water-based nutrient solution instead of soil (Khan et al. Citation2020). As irrigation systems are isolated, with continuous water circulation and less evaporation, both vertical and horizontal hydroponic crops consume less water. A recent study has shown that hydroponic lettuce had increased moisture, stronger roots, and higher lignin accumulation compared to soil-grown lettuce. Note that lignin plays an important role in plant growth and development by improving the rigidity of the plant cell wall, providing hydrophobicity, favoring mineral transport through the vascular bundles, and providing dietary fiber to consumers. Results also showed that there was no significant difference in morphological features between hydroponics and soil-grown lettuce (Frasetya et al. Citation2021). Hydroponics achieved higher harvest index values than soil-based systems (Manos and Xydis Citation2019). Removing the need for a root substrate or soil could further reduce system mass and allow for automated food production (van Delden et al. Citation2021). However, proper root aeration is pivotal to including hydroponic systems in spaceflight, designating the need for gas supply.
Studies revealed that sparged bubble size has an inverse effect for hydroponic cultivation, achieving better results with smaller bubbles. In a comparative study, Abu-Shahba et al. (Citation2021) concluded that lettuce grown with microbubbles achieved the best plant growth, secondary metabolites and antioxidants compared to plants grown with macrobubbles or without bubbles. In deep-flow hydroponics, systems commonly used to grow plants with a short growth period, microbubbles of air achieved 2.1 and 1.7 times larger fresh and dry weights in lettuce, respectively, than macrobubbles (Park and Kurata Citation2009). Similarly, microbubbles saturated water with dissolved oxygen more effectively than macrobubbles in deep-flow hydroponics for spinach plants (Ikeura et al. Citation2017). The exponential growth phase of plants is benefitted the most from increased oxygen availability via microbubbles, when roots are trying to support the fastest growth period. However, note that microbubbles are still larger in size than NB. With the inverse relationship of smaller bubble sizes providing higher gas supply efficiency, NBs are several orders of magnitude smaller than micro- and macrobubbles (d), positioning NBs in the forefront for achieving the best plant growth characteristics in hydroponics. This nanoscale transition will be a significant advancement for growing plants on spacecraft through increased hydroponic efficiency. Higher plant yields with faster maturity or fruiting times would help keep astronauts fed with fresh vegetables daily.
Nanobubbles and their unique nanoscale properties
Nanobubbles (NBs) are tiny gas-filled cavities immersed within liquid media, characterized by dimensions usually below 300 nm (Ulatowski and Sobieszuk Citation2018). The existence of these metastable nanointerfaces contradicts the traditional Young-Laplace theory, which determines the pressure inside the bubble, and the Epstein-Plesset theory of bubble dissolution and growth (Alheshibri et al. Citation2016). However, experimental evidence demonstrates extreme long-term stabilities of NBs in solution, where they can remain for up to months (Agarwal et al. Citation2011; Seddon et al. Citation2012). These discrepancies between theoretical prognoses and empirical observations underscore the necessity for deeper comprehension of the mechanisms governing NBs stability (Attard Citation2014; Alheshibri et al. Citation2016). Empirical studies have shown the remarkable resilience of NBs may be due in part to their surface tension and the electrostatic charges that prevent bubble coalescence (a) (Shin et al. Citation2015; Alheshibri et al. Citation2016; Michailidi et al. Citation2020; Yildirim et al. Citation2022; Cho et al. Citation2023). Note that the stability of NBs depends upon NB zeta potential values that decrease the probability of their coalescence (Han et al. Citation2022). One of the unique nanoscale properties of NBs is their agnostic character against buoyancy forces (d). Instead, the NB movement is dictated by Brownian motion (Atkinson et al. Citation2019). Additionally, NBs have high surface area and high zeta potential, which signifies the repulsive forces between bubbles (Uchida et al. Citation2011; Alheshibri et al. Citation2016), reactive oxygen species (ROS) formation (Liu et al. Citation2016a; Takahashi et al. Citation2021), and improved gas–liquid mass transfer (Cerrón-Calle et al. Citation2022; Sharma and Nirmalkar Citation2022) ().
Figure 1. Unique properties of NBs for seed germination and plant growth. a) Electrostatic repulsion between NBs with the same surface charge prevents coalescence, while attracting ions with different surface charges, increasing their bioavailability; b) The gas-water interfacial area of NBs and the associated high mass transfer coefficient allow a high gas supply to roots and beneficial bacteria in the rhizosphere; c) Reactive oxygen species (ROS, e.g. (hydroxyl (•OH), superoxide (O2•−), singlet oxygen (1O2)) can be formed using NBs to react in the bulk or by NB collapse, promoting seed germination and eliminating water-bound diseases; d) NBs have a larger surface area and interfacial free energy than larger bubbles, and are not affected by buoyancy forces, allowing NBs to remain longer in the water and interact with the plant.
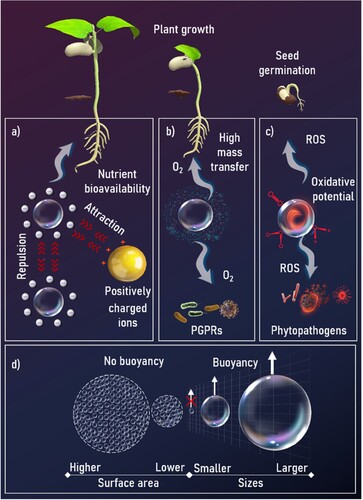
The higher interfacial surface area per volume of gas and longer lifetime in water for NBs offers a significant improvement in gas transfer efficiency compared to micro- or larger bubbles (b). This enhanced efficiency in gas–liquid contacting provides opportunities to deliver different gases, including CO2, O2, N2 and air. Increasing bubble longevity, resulting in longer saturation time and significantly increased use efficiency (i.e. from 0.01% up to 60%) will be a paradigm shift for mass and volume-constrained life support systems (Magdaleno et al. Citation2023; Zimmerman et al. Citation2011). In microgravity environments, multiphase flow can be chaotic, hard to predict movement, and difficult to sustain (Arias et al. Citation2009). NBs may be able to address these issues and expand the design space of multiphase systems to include technologies that rely on gases dissolved in solution. To date, there are no data showing how NBs behave in microgravity environments. Nonetheless, this unusual physical behavior may also present advantages in low-gravity conditions if NBs behave in spaceflight similarly to terrestrial scenarios. Experiments that provide new data in this area are indispensable, so further research on this topic will be needed.
Benefits of nanobubbles for terrestrial food production
Research has demonstrated that different gases in form of NBs may stimulate seed germination and plant growth in a variety of soil-based crop experiments, including lettuce, carrot, fava bean, spinach and tomato. For instance, Ahmed et al. (Citation2018a) achieved 25% more sprouting in lettuce seeds exposed to N2. Similarly, the use of air NB-infused water for growing Brassica campestris and lettuce plants demonstrated a significant growth increase (e.g. 9% of leaf length and 34% of aerial fresh weight in B. campestris) compared to normal water (Ebina et al. Citation2013; Kobayashi and Yamaji Citation2021). Reports indicate that there has been an increase in stem length, the number of leaves, and overall root growth when plants were exposed to NBs of O2 CO2, and N2 gases () (Zhou et al. Citation2019; Iijima et al. Citation2020; Pal and Anantharaman Citation2022; Babu and Amamcharla Citation2022). In particular, an improvement in fruit and soil quality was observed, with crop yields up to 24% and 40% in cucumbers and tomatoes due to effective NB-assisted oxygenation, respectively (Liu et al. Citation2019; Zhou et al. Citation2019).
Figure 2. Illustration of the multiple potential benefits of using nanobubbles in bioregenerative food production.
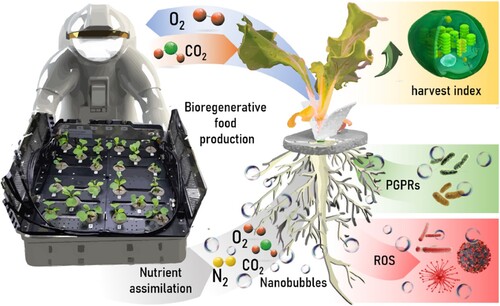
Other authors have reported sprouting rates of seeds immersed in NB gas-mixed water similar to those of hydrogen peroxide, noting that a moderate level of exogenous ROS produced by NB may influence seed physiology, induce plant growth, and result in faster germination rates (Liu et al. Citation2016; Ahmed et al. Citation2018b; Liu et al. Citation2017, Citation2016b, Citation2014, Citation2013; Oshita et al. Citation2020; Siregar et al. Citation2020). The level of ROS generated by NBs serves a critical function by loosening the cell wall and acts as a fundamental signaling molecule during plant growth (Müller et al. Citation2009; Kai et al. Citation2016; Oshita et al. Citation2023).
Beyond direct physiological interactions, NBs can also activate multiple pathways that promote plant utilization of soil nutrients () (Wang et al. Citation2021). It is hypothesized that electrostatic attraction of positively charged nutrients on negatively charged NBs may facilitate faster and strategized nutrient transport towards the plant roots, enhancing nutrient bioavailability (Park and Kurata Citation2009; Nirmalkar et al. Citation2018). Note that attention should be driven to effects of ionic strength on nutrient transport and NB long-term stability (Zhou et al. Citation2021). Wang et al. (Citation2021) suggested this NB nutrient transport induced the expression of genes responsible for root elongation and height of rice plants. Furthermore, stimulation of growth hormone synthesis and upregulation of plant nutrient absorption genes were observed in the NB-treated samples (Wang et al. Citation2021). This improvement in nutrient uptake reduced fertilizer use by 25%. The growth promotion was attributed to the NB-induced increase in partial pressure of dissolved oxygen and negative NB surface charge attracting positively charged ions dissolved in the nutrient solution (a) (Park and Kurata Citation2009).
Terrestrial measurements suggest that NBs are negatively charged and maintain their zeta potential over time (Nirmalkar et al. Citation2018). This property plays an important role in particle removal processes through increased particle aggregation (Atkinson et al. Citation2019; Meegoda et al. Citation2019), and perhaps also influences nutrient bioavailability processes in nutrient-rich waters for plant irrigation. The exploit of these metastable nanointerfaces of gas in liquid that behave as perfect homogeneous dissolutions is at its infancy. Nevertheless, NB technology has started demonstrating outstanding positive impacts on conventional agricultural systems and hydroponics.
Nanobubble impacts on microbial communities
Controlled NB delivery in hydroponic systems may play a key role in modulating microbiological communities by balancing beneficial and harmful microbes (). One challenge of protecting the microbiome of extensive hydroponic crops is the prevention of waterborne diseases. Some of the most common phytopathogens, including bacteria, viruses and fungi, can quickly spread throughout the system, as irrigation systems are typically connected to each container (Carducci et al. Citation2015; Lee and Lee Citation2015; Fortuna et al. Citation2023). This threatens plant viability and limits hydroponic yields, even in indoor systems (Owen-Going et al. Citation2003). For this reason, the main strategy in hydroponics is to keep the system as aseptic as possible. NB technology can become a paradigm shift for crop protection. Oxygen NBs can be a sustained source of ROS with high bactericidal activity, as a superoxide radical or hydroxyl radical (Liu et al. Citation2016; Takahashi and Chiba Citation2007). Bactericidal activity of ROS can prevent biofouling and organic fouling on pipes and other surfaces (Zhu et al. Citation2016). For instance, it has been demonstrated that NBs are able to remove biofilms from plastic and stainless-steel coupons, achieving a reduction of 1 to 3 log colony forming units (CFU)/cm2 within minutes (Shiroodi et al. Citation2021). In addition, the NB treatment influenced the surface tension of the surfaces, which decreased bacterial adhesion (Jiang et al. Citation2021) . Likewise, experiments with membranes showed that NBs delayed the flux attenuation and promoted antifouling ability of 165.9% (Fan et al. Citation2022). Ozone NBs could delay spoilage and prevent foodborne illness caused by persistent microorganisms when used during produce washing, handling, preparation, and storage of fresh vegetables (Zhang et al. Citation2022). Increased efficiency in gas delivery and long-term stability of NB provides sustained inactivation of pathogens and peak performance with less gas than conventional macrobubbling (Kyzas et al. Citation2019; Thi Phan et al. Citation2020; Seridou and Kalogerakis Citation2021; Magdaleno et al. Citation2023). Therefore, supplying NBs as an oxidizer/disinfectant option for hydroponic crops provides a more efficient and healthier alternative to other phytosanitary products (c). This is especially attractive for surface missions, where resupply of growth chamber consumables may be launch mass prohibitive, and gases generated in-situ may allow the generation of NBs anywhere.
Plant growth and harvest indexes are also closely related to microbial metabolic activity associated with and near plant roots. It has been observed that irrigation of sugarcane with air NBs-enriched water influenced the microbial community diversity and promoted bacterial metabolic functions related to nitrification and nitrogen fixation, resulting in improved soil fruitfulness and plant growth performance (Zhou et al. Citation2020). Similarly, this was observed with oxygen NBs, and their ability to increase microbial metabolic activity to provide a greater available nitrogen and phosphorous in the soil for tomato plants (Wu et al. Citation2019).
Certain microorganisms are beneficial and can even enhance plant growth (). Therefore, targeting and controlling NBs action zones could result in an increased harvest index. In the rhizosphere, microbial communities are fundamental for nutrient recycling, tolerance to biotic and abiotic stress, and plant-growth promotion (Philippot et al. Citation2013; Fierer Citation2017; Dhawi Citation2023). Thus, plant growth-promoting rhizobacteria (PGPRs) have been widely used in soil agriculture to increase germination rate, root, leaf and stem development, photosynthesis yield, chlorophyll ratio, nitrogen fixation and protein production, hydraulic activity, drought tolerance, delay leaf aging and provide resistance to some diseases (Azizoglu et al. Citation2021). NBs may have the ability to boost microbial metabolism and shift microbial communities in solution; hence their application for PGPR development and maintenance in soilless environments warrants investigation.
Hydroponic systems with direct root-to-water contact rely on gas transfer through the water media to supply the root system with the required gas species. However, gas transfer process is inefficient and occurs at a slower timescale than gas utilization in the root system. In this frame, hydroponics are themed to be gas-starved systems, whose performance might be enhanced with effective gas delivery. There is clear evidence that the high mass transfer of NBs could increase dissolved oxygen in nutrient-rich waters, promoting hydroponic production (Li and Cave Citation2019; Kobayashi and Yamaji Citation2021). These results are in line with previous studies where microbubbles were used to maintain a high concentration of dissolved oxygen in roots during the growth of lettuce and spinach (Park and Kurata Citation2009; Ikeura et al. Citation2017). Increasing the harvest index of these antioxidant-rich crops could enhance radiation protection for exploration astronauts. However, characterizing the producibility, stability, and absorption of these NBs in a microgravity environment will help identify use cases for these nano-sized gas pockets.
Nanobubble experiments for plant cultivation in terrestrial and spaceflight environments
The utilization of NBs in food production and agricultural practices on Earth has promising results. However, the incorporation of NBs in spaceflight systems necessitates the assessment of NBs’ role and behavior under microgravity conditions. Given the novelty of NB technology, there is a lack of research regarding how NB behavior is influenced by microgravity. Pioneered by NASA’s Life and Physical Science Division, along with the Advanced Exploration Systems Division, recent endeavors have investigated plant growth in terrestrial experiments for future spaceflights (‘Nanobubbles grow ”space crops,”’ Citation2020). However, there are still many unknowns regarding the interrelation between NB gas nature (e.g. O2, CO2, N2, etc.) and the crop species, the ability for nitrifying bacteria fed by NBs and urine to sustain different types of photoautotrophs (higher plants, microalgae), and the necessary NB density to sustain multiple harvests of salad crops. The hydroponic cultures are more sensitive to gas delivery via NB when compared to soil, given the direct exposure of roots to NBs-enriched water. Understanding the gas-root interaction mechanism in soilless systems appears as a key fundamental question that will define NBs concentration doses required to enhance crop yield. Thus, developing the knowledge on how NBs influence plant physiology in terms of photosynthetic performance, nutrient assimilation and root activity in hydroponics can be identified as an essential stepstone for NBs technology implementation in aerospace and terrestrial agriculture systems. Future research endeavors focusing on the use of NB technologies for enhanced agriculture systems hold the promise to shape food-production methods for supporting and sustaining human space exploration.
Conclusion
Exploration missions beyond LEO will require fresh produce as nutritional supplementation to ensure necessary nutritional profiles are fulfilled across a multi-year mission, increase menu diversity, and to reduce launched consumables. Initial plant growth studies conducted on the ISS proved that plant growth is possible in a microgravity environment, but higher harvest indexes or VUE is necessary to sustain crew while fitting with the volumetric constraints of a spaceflight vehicle. Terrestrially, oxygen micro- and macrobubbles sparged into hydroponic systems stimulate growth and productivity of salad crops. However, this buoyancy-driven method for gas–liquid contacting is inappropriate for microgravity environments. Reducing the bubbles down to the nano scale removes the gravity dependence for movement, increases the stability of the bubble, and the overall surface area to volume ratio. Ground-based NB experiments resulted in faster growth rates and a higher harvest index, when compared to the larger bubble-sparged systems. This implies that NBs in a hydroponics system may stimulate plant growth to meet the needs of a plant growth chamber in the microgravity environment. However, the incorporation of NBs into terrestrial agricultural systems is novel, with no NB experiments in microgravity to date. Therefore, characterizing NB production, stability, and integration in microgravity systems needs to be a priority for any sector interested in increasing the overall efficiency of plant growth systems for exploration missions.
Disclosure statement
No potential conflict of interest was reported by the author(s).
Additional information
Funding
Notes on contributors
Jesús Morón-López
Dr. Jesús Morón-Lopez is a postdoctoral researcher at Arizona State University in the School of Sustainable Engineering and the Built Environment. He holds a Ph.D. in Hydrology and Water Resources Management from the University of Alcalá, a M.Sc. in Molecular Genetic and Biotechnology and a B.Sc. degree in Biology from the University of Seville. He has extensive international experience in multidisciplinary teams related to water quality. He is involved in several research projects focused on the use of nanobubbles for water treatment, including harmful algae mitigation, aquaculture or hydroponic crop improvement. He is author of 17 peer-reviewed articles, one book and two international patents.
Renato Montenegro-Ayo
Renato Martin Montenegro Ayo is a Ph.D. student specializing in Civil, Environmental, and Sustainable Engineering at Arizona State University (ASU). He earned his B.Sc. degree in Industrial Engineering from the University of Lima, Peru. Beyond his academic pursuits, Renato stands as a notable figure in the Peruvian business sector. He is the CEO of Analyzen Peru SAC, a leading technological firm dedicated to the design, construction, and implementation of advanced autonomous systems for biological and electrochemical wastewater treatment. Additionally, he serves as the CTO of LimaEM SAC, a company proficient in wastewater treatment utilizing both biological and electrochemical reactors. With a vast international background, Renato boasts expertise in the automation, design, control, and enhancement of advanced water treatment solutions for industrial applications. Currently, at ASU, his research delves into amplifying lettuce growth in germination and hydroponic environments using the innovative nanobubble technology. Renato’s future work aims to enhance astronauts’ living conditions in space enabling food production.
Andrea Maya
Andrea Maya is an undergraduate student majoring in Environmental Engineering at Arizona State University (ASU). She holds an A.S. in Science with an emphasis in Engineering from Glendale Community College (GCC). Andrea aspires to continue her education, earning a master’s degree and a Ph.D. in Engineering. With years of research experience focused on plant nano-interactions, Andrea is passionate about advancing sustainable solutions that increase crop yield with minimal water footprint. Her current research centers on improving plant and seed germination using nanobubbles. Andrea’s keen interest lies in managing and optimizing life support systems within spacecraft and space habitats, contributing to the future of space exploration and sustainability.
Sudheera Yaparatne
Dr. Sudheera Yaparatne is a postdoctoral researcher in the Department of Civil and Environmental Engineering at the New Jersey Institute of Technology. He obtained his Ph.D. in chemistry from the University of Maine at Orono. His research focuses on advancing innovative and sustainable methods for water and wastewater treatment. His research specialization includes nanobubble applications in environmental contexts, the development of nano photocatalysts, analytical chemistry, and material characterization.
Mariana Hernandez-Molina
Mariana Hernández Molina is a Civil, Environmental and Sustainable Engineering Ph.D. student at Arizona State University (ASU). She holds a M.S.E. in Civil, Environmental and Sustainable Engineering from (ASU) and B.Sc. degree in Environmental Science with a concentration in Chemistry from the University of Texas at El Paso (UTEP). She has multidisciplinary research experience related to nano-biointeractions in plants and membrane processes. Her research interests are on water-energy-food nexus. She is currently involved in research projects focused on the use of nanobubbles for membrane scaling and fouling mitigation in water treatment (water-energy nexus), and nanobubble applications for enhancement of seed germination and plant growth for food production in extreme environments (water-food nexus).
John Graf
Dr. John Graf serves as Technology Development Lead for Life Support at NASA’s Johnson Space Center. In his role as technology development lead, he leads efforts to identify emerging technologies, and direct their potential to atmospheric control and water reuse for human spaceflight. He earned his Ph.D. at Michigan Technological University and is a registered Professional Engineer in the state of Wisconsin.
Onur Apul
Dr. Onur Apul is an Assistant Professor of Environmental Engineering and a Libra Professor in Civil and Environmental Engineering at the University of Maine. He serves as a steering committee member and the science lead of UMaine PFAS + Research Initiative. He earned his Ph.D. degree from Environmental Engineering and Earth Sciences at Clemson University in 2014. His research focuses on unraveling complex intermolecular interactions between synthetic organic compounds and carbon-based adsorbents to improve safe and sustainable practices in water treatment. Most recently he studies nanobubble-organic compound interactions to understand the physicochemical characteristics of nanobubbles. He has published over 75 peer-reviewed articles (h = 27) and received nationally-competitive research awards.
Sergi Garcia-Segura
Dr. Sergi Garcia-Segura is an Assistant Professor at Arizona State University in the School of Sustainable Engineering and the Built Environment. He holds a Ph.D. in Chemistry from the University of Barcelona (UB), an M.Sc. in Electrochemistry from the University of Alicante, and two B.Sc. degrees in both Chemistry and Materials Science Engineering from UB and the Polytechnic University of Catalonia, respectively. He has an international and multidisciplinary research background, having worked across four continents in groups studying physical and analytical chemistry, materials science, and environmental engineering. His research explores the use of interfaces for environmental remediation including electrocatalysis and nanobubble technologies. He has published over 140 peer-reviewed articles (h = 56) and received multiple international research awards.
Emily E. Matula
Dr. Emily E. Matula is an extravehicular activity instructor and flight controller at NASA's Johnson Space Center. She completed her Ph.D. at the University of Colorado Boulder in the Smead Aerospace Engineering Sciences department with a Bioastronautics focus funded through a NASA Space Technology Research Fellowship. Her dissertation titled, ‘Characterization of photobioregenerative technology for simultaneous thermal control and air revitalization of spacecraft and surface habitats’ focused on the potential of a multifunctional, bioregenerative environmental control and life support system.
References
- Abu-Shahba MS, Mansour MM, Mohamed HI, Sofy MR. 2021. Comparative cultivation and biochemical analysis of iceberg lettuce grown in sand soil and hydroponics with or without microbubbles and macrobubbles. J Soil Sci Plant Nutr. 21(1):389–403. doi:10.1007/s42729-020-00368-x.
- Agarwal A, Ng WJ, Liu Y. 2011. Principle and applications of microbubble and nanobubble technology for water treatment. Chemosphere. 84(9):1175–1180. doi:10.1016/j.chemosphere.2011.05.054.
- Ahmed AKA, Shi X, Hua L, Manzueta L, Qing W, Marhaba T, Zhang W. 2018a. Influences of air, oxygen, nitrogen, and carbon dioxide nanobubbles on seed germination and plant growth. J Agric Food Chem. 66:5117–5124. doi:10.1021/acs.jafc.8b00333.
- Ahmed AKA, Sun C, Hua L, Zhang Z, Zhang Y, Zhang W, Marhaba T. 2018b. Generation of nanobubbles by ceramic membrane filters: the dependence of bubble size and zeta potential on surface coating, pore size and injected gas pressure. Chemosphere. 203:327–335. doi:10.1016/j.chemosphere.2018.03.157.
- Alheshibri M, Qian J, Jehannin M, Craig VSJ. 2016. A history of nanobubbles. Langmuir. 32(43):11086–11100. doi:10.1021/acs.langmuir.6b02489.
- Arias S, Ruiz X, Casademunt J, Ramírez-Piscina L, González-Cinca R. 2009. Experimental study of a microchannel bubble injector for microgravity applications. Microgravity Sci Technol. 21:107–111. doi:10.1007/s12217-008-9060.
- Atkinson AJ, Apul OG, Schneider O, Garcia-Segura S, Westerhoff P. 2019. Nanobubble Technologies Offer Opportunities to Improve Water Treatment. Acc Chem Res. 52(5):1196–1205. doi:10.1021/acs.accounts.8b00606.
- Attard P. 2014. The stability of nanobubbles. Eur Phys J Spec Top. 223:893–914. doi:10.1140/epjst/e2013-01817-0.
- Azizoglu U, Yilmaz N, Simsek O, Ibal JC, Tagele SB, Shin J-H. 2021. The fate of plant growth-promoting rhizobacteria in soilless agriculture: future perspectives. Biotech. 11(3):382. doi:10.1007/s13205-021-02941-2.
- Babu KS, Amamcharla JK. 2022. Generation methods, stability, detection techniques, and applications of bulk nanobubbles in agro-food industries: a review and future perspective. Crit Rev Food Sci Nutr. 0:1–20. doi:10.1080/10408398.2022.2067119.
- Buckey JC. 2006. Space physiology. New York: Oxford Press. Retrieved from https://books.google.com/books?id=RYnxmAEACAAJ.
- Carducci A, Caponi E, Ciurli A, Verani M. 2015. Possible internalization of an enterovirus in hydroponically grown lettuce. Int J Environ Res Public Health. 12(7):8214. doi:10.3390/ijerph120708214.
- Cerrón-Calle GA, Luna Magdaleno A, Graf JC, Apul OG, Garcia-Segura S. 2022. Elucidating CO2 nanobubble interfacial reactivity and impacts on water chemistry. J Colloid Interface Sci. 607:720–728. doi:10.1016/j.jcis.2021.09.033.
- Cho C-H, Shin H-J, Singh B, Kim K, Park M-H. 2023. Assessment of sub-200-nm nanobubbles with ultra-high stability in water. Appl Water Sci. 13:149. doi:10.1007/s13201-023-01950-1.
- Cooper M, Perchonok M, Douglas GL. 2017 December. Initial assessment of the nutritional quality of the space food system over three years of ambient storage. Npj Microgravity. 3. doi:10.1038/s41526-017-0022-z.
- de Micco V, Aronne G, Colla G, Fortezza R, de Pascale S. 2009 December. Agro-biology for bioregenerative life support systems in long-term space missions: general constraints and the Italian efforts. J Plant Interact. 4:241–252. doi:10.1080/17429140903161348.
- Dhawi F. 2023. The role of plant growth-promoting microorganisms (PGPMs) and their feasibility in hydroponics and vertical farming. Metabolites. 13(2):247. doi:10.3390/metabo13020247.
- Douglas GL, Wheeler RM, Fritsche RF. 2021 August. Sustaining astronauts: resource limitations, technology needs, and parallels between spaceflight food systems and those on earth. Sustainability (Switzerland). 13:9424. doi:10.3390/su13169424.
- Douglas GL, Zwart SR, Smith SM. 2020 September. Space food for thought: challenges and considerations for food and nutrition on exploration missions. J Nutr. 150:2242–2244. doi:10.1093/jn/nxaa188.
- Dowdy R. 2020 August. Space Station 20th: food on ISS. Retrieved from https://www.nasa.gov/feature/space-station-20th-food-on-iss.
- Du YD, Niu WQ, Gu XB, Zhang Q, Cui BJ, Zhao Y. 2018 November. Crop yield and water use efficiency under aerated irrigation: a meta-analysis. Agric Water Manag. 210:158–164. doi:10.1016/j.agwat.2018.07.038.
- Ebina K, Shi K, Hirao M, Hashimoto J, Kawato Y, Kaneshiro S, Morimoto T, Koizumi K, Yoshikawa H. 2013. Oxygen and air nanobubble water solution promote the growth of plants, fishes, and mice. PLoS ONE. 8:e65339. doi:10.1371/journal.pone.0065339.
- Ellery A. 2021. Supplementing closed ecological life support systems with in-situ resources on the moon. Life. 11(8). doi:10.3390/life11080770.
- Ewert MK, Chen TT, Powell CD. 2022. Life support baseline values and assumptions document. Retrieved from http://www.sti.nasa.gov.
- Fan K, Huang Z, Lin H, Shen L, Gao C, Zhou G, Hu J, Yang H, Xu F. 2022. Effects of micro-/nanobubble on membrane antifouling performance and the mechanism insights. J Cleaner Prod. 376:1–9. doi:10.1016/j.jclepro.2022.134331.
- Fierer N. 2017. Embracing the unknown: disentangling the complexities of the soil microbiome. Nat Rev Microbiol. 15. doi:10.1038/nrmicro.2017.87.
- Fortuna KJ, Holtappels D, Venneman J, Baeyen S, Vallino M, Verwilt P, Rediers H, De Coninck B, Maes M, Van Vaerenbergh J, et al. 2023. Back to the roots: agrobacterium-specific phages show potential to disinfect nutrient solution from hydroponic greenhouses. Appl Environ Microbiol. 89(4). doi:10.1128/AEM.00215-23/SUPPL_FILE/AEM.00215-23-S0001.PDF.
- Frasetya B, Harisman K, Ramdaniah NAH. 2021. The effect of hydroponics systems on the growth of lettuce. IOP Conf Ser: Mater Sci Eng. 1098(4):042115. doi:10.1088/1757-899X/1098/4/042115.
- Graham T, Wheeler R. 2016 June. Root restriction: a tool for improving volume utilization efficiency in bioregenerative life-support systems. Life Sci Space Res (Amst). 9:62–68. doi:10.1016/j.lssr.2016.04.001.
- Grooms L. 2020 August. Nanobubbles grow “space crops”.AgUpdate. Retrieved from https://agupdate.com/agriview/news/business/nanobubbles-grow-space-crops/article_faee850a-b348-5903-a024-295449e684db.html.
- Guo W, Ngo H-H, Li J. 2012. A mini-review on membrane fouling. Biores Technol. 122:27–34. doi:10.1016/j.biortech.2012.04.089.
- Han Z, Kurokawa H, Matsui H, He C, Wang K, Wei Y, Dodbiba G, Otsuki A, Fujita T. 2022. Stability and free radical production for CO2 and H2 in air nanobubbles in ethanol aqueous solution. Nanomaterials. 12:237. doi:10.3390/nano12020237.
- Hodgson L. 2023, January 5. 15 healthiest vegetables: nutrition and health benefits. Medical News Today. https://www.medicalnewstoday.com/articles/323319.
- Iijima M, Yamashita K, Hirooka Y, Ueda Y, Yamane K, Kamimura C. 2020. Ultrafine bubbles effectively enhance soybean seedling growth under nutrient deficit stress. Plant Prod Sci. 23(3):366–373. doi:10.1080/1343943X.2020.1725391.
- Ikeura H, Tsukada K, Tamaki M. 2017. Effect of microbubbles in deep flow hydroponic culture on spinach growth. J Plant Nutr. 40(16):2358–2364. doi:10.1080/01904167.2017.1346663.
- Jiang X, Wang W, Yu G, Deng S. 2021. Contribution of Nanobubbles for PFAS Adsorption on graphene and OH- and NH2-functionalized graphene: comparing simulations with experimental results. Environ Sci Technol. 55:13254–13263. doi:10.1021/acs.est.1c03022.
- Johnson CM, Boles HO, Spencer LSE, Poulet L, Romeyn M, Bunchek JM, … Wheeler RM. 2021 November. Supplemental food production with plants: a review of NASA research. Front Astron Space Sci. 8. doi:10.3389/fspas.2021.734343.
- Kai K, Kasa S, Sakamoto M, Aoki N, Watabe G, Yuasa T, Iwaya-Inoue M, Ishibashi Y. 2016. Role of reactive oxygen species produced by NADPH oxidase in gibberellin biosynthesis during barley seed germination. Plant Signal Behav. 11:e1180492. doi:10.1080/15592324.2016.1180492.
- Kaschubek D. 2021 August. Optimized crop growth area composition for long duration spaceflight. Life Sci Space Res (Amst). 30:55–65. doi:10.1016/j.lssr.2021.05.005.
- Khan S, Purohit A, Vadsaria N. 2020. Hydroponics: current and future state of the art in farming. J Plant Nutr. 44(10):1515–1538. doi:10.1080/01904167.2020.1860217.
- Kobayashi N, Yamaji K. 2021. Leaf lettuce (Lactuca sativa L. ‘L-121’) growth in hydroponics with different nutrient solutions used to generate ultrafine bubbles. J Plant Nutr. 45(6):816–827. doi:10.1080/01904167.2021.2006227.
- Kulkarni S, Gandhi D, Mehta PJ. 2022 January. Nutraceuticals for reducing radiation effects during space travel. Handbook of Space Pharmaceuticals. doi:10.1007/978-3-030-05526-4_54.
- Kyzas GZ, Bomis G, Kosheleva RI, Efthimiadou EK, Favvas EP, Kostoglou M, Mitropoulos AC. 2019. Nanobubbles effect on heavy metal ions adsorption by activated carbon. Chem Eng J. 356:91–97. doi:10.1016/j.cej.2018.09.019.
- Lee S, Lee J. 2015. Beneficial bacteria and fungi in hydroponic systems: types and characteristics of hydroponic food production methods. Sci Hortic. 195:206–215. doi:10.1016/j.scienta.2015.09.011.
- Levine LH, Kasahara H, Kopka J, Erban A, Fehrl I, Kaplan F, Zhao W, Littell RC, Guy C, Wheeler R, et al. 2008. Physiologic and metabolic responses of wheat seedlings to elevated and super-elevated carbon dioxide. Adv Space Res. 42(12):1917–1928. doi:10.1016/j.asr.2008.07.014.
- Li Y, Cave R. 2019. Nanobubbles in hydroponics. Proc AMIA Annu Fall Symp. 36:39. doi:10.3390/proceedings2019036039.
- Liu S, Kawagoe Y, Makino Y, Oshita S. 2013. Effects of nanobubbles on the physicochemical properties of water: the basis for peculiar properties of water containing nanobubbles. Chem Eng Sci. 93:250–256. doi:10.1016/j.ces.2013.02.004.
- Liu S, Oshita S, Kawabata S, Makino Y, Yoshimoto T. 2016. Identification of ROS produced by nanobubbles and their positive and negative effects on vegetable seed germination. Langmuir. 32(43):11295–11302. doi:10.1021/acs.langmuir.6b01621.
- Liu S, Oshita S, Kawabata S, Thuyet DQ. 2017. Nanobubble water’s promotion effect of barley (Hordeum vulgare L.) sprouts supported by RNA-Seq analysis. Langmuir. 33:12478–12486. doi:10.1021/acs.langmuir.7b02290.
- Liu S, Oshita S, Makino Y. 2014. Stimulating effect of nanobubbles on the reactive oxygen species generation inside barley seeds as studied by the microscope spectrophotometer, In: Proceedings]. Int. Conf. of Agric. Eng., Zurich. 06–10.
- Liu S, Oshita S, Makino Y, Wang Q, Kawagoe Y, Uchida T. 2016b. Oxidative capacity of nanobubbles and its effect on seed germination. ACS Sustain Chem Eng. 4:1347–1353. doi:10.1021/acssuschemeng.5b01368.
- Liu Y, Zhou Y, Wang T, Pan J, Zhou B, Muhammad T, Zhou C, Li Y. 2019. Micro-nano bubble water oxygation: synergistically improving irrigation water use efficiency, crop yield and quality. J Cleaner Prod. 222:835–843. doi:10.1016/j.jclepro.2019.02.208.
- Ma L, Chai C, Wu W, Qi P, Liu X, Hao J. 2023. Hydrogels as the plant culture substrates: a review. Carbohydr Polym. 305. doi:10.1016/j.carbpol.2023.120544.
- Magdaleno AL, Cerrón-calle GA, Santos AJ, Lanza MRV, Apul OG, Garcia-segura S. 2023. Unlocking the potential of nanobubbles: achieving exceptional Gas efficiency in electrogeneration of hydrogen peroxide. Small. 2304547:1–10. doi:10.1002/smll.202304547.
- Manos DP, Xydis G. 2019. Hydroponics: are we moving towards that direction only because of the environment? a discussion on forecasting and a systems review. Environ Sci Pollut Res. 26(13):12662–12672. doi:10.1007/s11356-019-04933-5.
- Massa G, Dufour N, Carver J, Hummerick M, Wheeler R, Morrow R, Smith T. 2017a. VEG-01: veggie hardware validation testing on the international space station. Open Agriculture. 2(1):33–41. doi:10.1515/opag-2017-0003.
- Massa GD, Romeyn M, Fritsche R. 2017b. Future food production system development pulling from space biology crop growth testing in veggie. Seattle, WA: American Society for Gravitational and Space Research. Oct 25-21.
- Massa GD, Wheeler RM, Morrow RC, Levine HG. 2016. Growth chambers on the international space station for large plants. Acta Hortic. 1134:215–221. doi:10.17660/ActaHortic.2016.1134.29.
- Massa GD, Wheeler RM, Stutte GW, Richards JT, Spencer LE, Hummerick ME, … Technology AF. 2015. Selection of leafy green vegetable varieties for a pick-and- eat diet supplement on ISS.
- Meegoda, J.N., Aluthgun Hewage, S., Batagoda, J.H., 2018. Stability of nanobubbles. Environ Eng Sci 35, 1216–1227. doi:10.1089/ees.2018.0203.
- Meegoda JN, Hewage SA, Batagoda JH. 2019. Application of the diffused double layer theory to nanobubbles. Langmuir. 35:10. doi:10.1021/acs.langmuir.9b01443.
- Michailidi ED, Bomis G, Varoutoglou A, Kyzas GZ, Mitrikas G, Mitropoulos AC, Efthimiadou EK, Favvas EP. 2020. Bulk nanobubbles: production and investigation of their formation/stability mechanism. J Colloid Interface Sci. 564:371–380. doi:10.1016/j.jcis.2019.12.093.
- Monje O, Stutte G, Chapman D. 2005. Microgravity does not alter plant stand gas exchange of wheat at moderate light levels and saturating CO2 concentration. Planta. 222(2):336–345. doi:10.1007/s00425-005-1529-1.
- Müller K, Linkies A, Vreeburg RAM, Fry SC, Krieger-Liszkay A, Leubner-Metzger G. 2009. In vivo cell wall loosening by hydroxyl radicals during cress seed germination and elongation growth. Plant Physiol. 150:1855–1865. doi:10.1104/pp.109.139204.
- Nguyen M, Knowling M, Tran NN, Burgess A, Fisk I, Watt M, Escribà-Gelonch M, This H, Culton J, Hessel V. 2023. Space farming: horticulture systems on spacecraft and outlook to planetary space exploration. Plant Physiol Biochem. 194:708–721. doi:10.1016/j.plaphy.2022.12.017.
- Nirmalkar N, Pacek AW, Barigou M. 2018. On the existence and stability of bulk nanobubbles. Langmuir. 34:10964–10973. doi:10.1021/acs.langmuir.8b01163.
- Oshita, S., Boerzhijin, S., Kameya, H., Yoshimura, M., Sotome, I., 2023. Promotion effects of ultrafine bubbles/nanobubbles on seed germination. Nanomaterials 13. doi:10.3390/nano13101677.
- Oshita S, Kamijo Y, Pham TQA, Yoshimura M, Sotome I, Kameya H, Fujita T, Liu S. 2020. Number concentration of ultrafine bubble being effective in promoting barley seed germination. 混相流(Miscible flow). 34:194–204.
- Owen-Going N, Sutton JC, Grodzinski B. 2003. Relationships of Pythium isolates and sweet pepper plants in single-plant hydroponic units. Can J Plant Pathol. 25(2):155–167. doi:10.1080/07060660309507064.
- Pal P, Anantharaman H. 2022. CO2 nanobubbles utility for enhanced plant growth and productivity: recent advances in agriculture. J CO2 Util. 61:102008. doi:10.1016/j.jcou.2022.102008.
- Park J-S, Kurata K. 2009. Application of microbubbles to hydroponics solution promotes lettuce growth. HortTechnology. 19:212–215. doi:10.21273/HORTTECH.19.1.212.
- Philippot L, Raaijmakers JM, Lemanceau P, Van Der Putten WH. 2013. Going back to the roots: the microbial ecology of the rhizosphere. Nat Rev Microbiol. 11(11):789–799. doi:10.1038/nrmicro3109.
- Seddon JRT, Lohse D, Ducker WA, Craig VSJ. 2012. A deliberation on nanobubbles at surfaces and in bulk. ChemPhysChem. 13(8):2179–2187. doi:10.1002/cphc.201100900.
- Seridou P, Kalogerakis N. 2021. Disinfection applications of ozone micro- and nanobubbles. Environmental Science: Nano. 8(12):3493–3510. doi:10.1039/D1EN00700A.
- Sharma H, Nirmalkar N. 2022. Enhanced gas-liquid mass transfer coefficient by bulk nanobubbles in water. Materials Today: proceedings, International Chemical Engineering Conference 2021 (100 Glorious Years of Chemical Engineering & Technology). 57:1838–1841. doi:10.1016/j.matpr.2022.01.029.
- Shin D, Park JB, Kim YJ, Kim SJ, Kang JH, Lee B, Cho SP, Hong BH, Novoselov KS. 2015. Growth dynamics and gas transport mechanism of nanobubbles in graphene liquid cells. Nat Commun. 6(1):1–6. doi:10.1038/ncomms7068.
- Shiroodi S, Schwarz MH, Nitin N, et al. 2021. Efficacy of nanobubbles alone or in combination with neutral electrolyzed water in removing escherichia coli O157:H7, vibrio parahaemolyticus, and listeria innocua biofilms. Food Bioprocess Technol. 14:287–297. doi:10.1007/s11947-020-02572-0.
- Siregar IZ, Muharam KF, Purawanto YA, Sudrajat DJ. 2020. Seed germination characteristics in different storage time of Gmelina arborea treated with ultrafine bubbles priming. Biodiversitas J Bio Divers. 21.
- Steinberg S, Ming DW, Henninger DL. 2002. Plant production systems for microgravity: critical issues in water, air, and solute transport through unsaturated porous media. In NASA Technical Report Server (No. 20020039552). Retrieved October 20, 2023, from https://ntrs.nasa.gov/api/citations/20020039552/downloads/20020039552.pdf.
- Stromberg J. 2015 August. NASA astronauts just ate food grown in space for the first time. Vox. Retrieved from https://www.vox.com/2015/8/10/9126949/nasa-food-space-station
- Takahashi M, Chiba K, Li P. 2007. Free-radical generation from collapsing microbubbles in the absence of a dynamic stimulus. J Phys Chem B. 111:1343–1347. doi:10.1021/jp0669254.
- Takahashi M, Shirai Y, Sugawa S. 2021. Free-radical generation from bulk nanobubbles in aqueous electrolyte solutions: ESR spin-trap observation of microbubble-treated water. Langmuir. 37:5005–5011. doi:10.1021/acs.langmuir.1c00469.
- Tan BH, An H, Ohl C-D. 2020. How bulk nanobubbles might survive. Phys Rev Lett. 124:134503. doi:10.1103/PhysRevLett.124.134503.
- Thi Phan, K.K., Truong, T., Wang, Y., Bhandari, B., 2020. Nanobubbles: fundamental characteristics and applications in food processing. Trends Food Sci Technol 95, 118–130. doi:10.1016/j.tifs.2019.11.019.
- Torres LJ, Jenson R, Weislogel M. 2020. Capillary Hydroponic Plant Watering System for Spacecraft. 2020 International Conference on Environmental Systems. 1–9.
- Uchida T, Oshita S, Ohmori M, Tsuno T, Soejima K, Shinozaki S, Take Y, Mitsuda K. 2011. Transmission electron microscopic observations of nanobubbles and their capture of impurities in wastewater. Nanoscale Res Lett. 6:295. doi:10.1186/1556-276X-6-295.
- Ulatowski K, Sobieszuk P. 2018. Influence of liquid flowrate on size of nanobubbles generated by porous-membrane modules. Chem Process Eng. 335–345.
- van Delden SH, SharathKumar M, Butturini M, Graamans LJA, Heuvelink E, Kacira M, Kaiser E, Klamer RS, Klerkx L, Kootstra G, et al. 2021. Current status and future challenges in implementing and upscaling vertical farming systems. Nature Food. 2(12):944–956. doi:10.1038/s43016-021-00402-w.
- Wang Y, Wang S, Sun J, Dai H, Zhang B, Xiang W, Hu Z, Li P, Yang J, Zhang W. 2021. Nanobubbles promote nutrient utilization and plant growth in rice by upregulating nutrient uptake genes and stimulating growth hormone production. Sci Total Environ. 800:149627. doi:10.1016/j.scitotenv.2021.149627.
- Watkins P, Hughes J, Gamage TV, Knoerzer K, Ferlazzo ML, Banati RB. 2022 February. Long term food stability for extended space missions: a review. Life Sci Space Res (Amst). 32:79–95. doi:10.1016/j.lssr.2021.12.003.
- Wheeler RM. 2003 January. Carbon balance in bioregenerative life support systems: some effects of system closure, waste management, and crop harvest index. Adv Space Res. 31:169–175. doi:10.1016/S0273-1177(02)00742-1.
- Wu Y, Lyu T, Yue B, Tonoli E, Verderio EAM, Ma Y, Pan G. 2019. Enhancement of tomato plant growth and productivity in organic farming by agri-nanotechnology using nanobubble oxygation. J Agric Food Chem. 67(39):10823–10831. doi:10.1021/acs.jafc.9b04117.
- Yaparatne S, Doherty ZE, Magdaleno AL, Matula EE, MacRae JD, Garcia-Segura S, Apul OG. 2022 May. Effect of air nanobubbles on oxygen transfer, oxygen uptake, and diversity of aerobic microbial consortium in activated sludge reactors. Bioresour Technol. 351:1–10. doi:10.1016/J.BIORTECH.2022.127090.
- Yildirim T, Yaparatne S, Graf J, Garcia-Segura S, Apul O. 2022. Electrostatic forces and higher order curvature terms of Young–Laplace equation on nanobubble stability in water. npj Clean Water. 5:1–3. doi:10.1038/s41545-022-00163-4.
- Zhang Z-H, Wang S, Cheng L, Ma H, Gao X, Brennan CS, Yan J-K. 2022. Micro-nano-bubble technology and its applications in food industry: a critical review. Food Rev Int. 1–23. doi:10.1080/87559129.2021.2023172.
- Zhou Y, Bastida F, Zhou B, Sun Y, Gu T, Li S, Li Y. 2020. Soil fertility and crop production are fostered by micro-nano bubble irrigation with associated changes in soil bacterial community. Soil Biol Biochem. 141:107663. doi:10.1016/j.soilbio.2019.107663.
- Zhou Y, Han Z, He C, Feng Q, Wang K, Wang Y, Luo N, Dodbiba G, Wei Y, Otsuki A, et al. 2021. Long-Term stability of different kinds of Gas nanobubbles in deionized and salt water. Materials (Basel). 14(7):1808. doi:10.3390/ma14071808.
- Zhou Y, Zhou B, Xu F, Muhammad T, Li Y. 2019. Appropriate dissolved oxygen concentration and application stage of micro-nano bubble water oxygation in greenhouse crop plantation. Agric Water Manag. 223:105713. doi:10.1016/j.agwat.2019.105713.
- Zhu J, An H, Alheshibri M, Liu L, Terpstra PMJ, Liu G, Craig VSJ. 2016. Cleaning with bulk nanobubbles. Langmuir. 32:11203–11211. doi:10.1021/acs.langmuir.6b01004.
- Zimmerman, W.B., Tesař, V., Bandulasena, H.C.H., 2011. Towards energy efficient nanobubble generation with fluidic oscillation. Curr Opin Colloid Interface Sci 16, 350–356. doi:10.1016/j.cocis.2011.01.010.