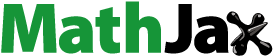
ABSTRACT
Effect of ultrasound treatment on antioxidant activity and structure of β-Lactoglobulin were investigated using antioxidant indexes and spectroscopy techniques. The 1,1-Diphenyl-2-picrylhydrazyl (DPPH) radical scavenging activities had been applied to the experimental response obtained by a three-factor-three-level Box–Behnken design. Results showed that ultrasound treatment significantly increased antioxidant activity (p < 0.05). The maximum DPPH radical scavenging activity of β-Lactoglobulin was 57.59% at the ultrasound conditions of 45°C, 20 min and amplitude of 30%. The ultrasound-treated β-Lactoglobulin exhibited higher 2,2ʹ-Azino-bis(3-ethylbenzothiazoline-6-sulfonic acid) diammonium salt radical scavenging activity and oxygen radical absorbance capacity. Circular dichroism data indicated that ultrasound treatment increased both α-helix and β-sheet contents of β-Lactoglobulin and altered Trp residues, which resulted in the secondary and tertiary structure changes. Differential scanning calorimeter and scanning electron microscopy micrographs showed that ultrasound-treated β-Lactoglobulin contained larger aggregates than untreated. In conclusion, ultrasound treatment had considerable effects on antioxidant activity and structure of β-Lactoglobulin. Ultrasound treatment may expand the applications of β-Lactoglobulin in food industries.
RESUMEN
El presente estudio investigó el efecto del tratamiento de ultrasonido en la actividad antioxidante y la estructura de la β-lactoglobulina, utilizando para ello índices antioxidantes y técnicas de espectroscopía. Las actividades de eliminación de radicales de DPPH habían sido aplicadas a la respuesta experimental obtenida por un diseño Box-Behnken de tres factores y tres niveles. Los resultados dan cuenta de que el tratamiento de ultrasonido elevó significativamente la actividad antioxidante (p < 0.05). La máxima actividad de eliminación de radicales de DPPH de la β-lactoglobulina fue 57.59%, en condiciones de ultrasonido de 45°C, 20 min y una amplitud de 30%. Se constató que la β-lactoglobulina tratada con ultrasonido mostró mayor actividad de eliminación de radicales de ABTS, así como mayor capacidad de absorción de radicales de oxígeno. Además, los datos de dicroismo circular indican que el tratamiento de ultrasonido aumentó los contenidos de hélice alfa y los de lámina beta de la β-lactoglobulina, alterando los residuales Trp, lo que resultó en cambios estructurales secundarios y terciarios. Las micrografías de calorimetría diferencial de barrido y microscopía electrónica de barrido revelaron que la β-lactoglobulina tratada mediante ultrasonido contiene agregados más grandes que aquella que no recibió este tratamiento. En conclusión, el tratamiento de ultrasonido tuvo efectos considerables en la actividad antioxidante y la estructura de la β-lactoglobulina. Por ello, el mismo puede ampliar las aplicaciones de la β-lactoglobulina en las industrias alimentarias.
Introduction
High-intensity ultrasound (HIU), a type of nonthermal technology has been attracting more attention (Aouzelleg, Bull, Price, & Kelly, Citation2004). Ultrasound (in general, > 20 kHz) has a wide application range in food processing (Chandrapala, Oliyer, Kentish, & Ashokkumar, Citation2012). A phenomenon associated with ultrasound is called acoustic cavitation, which is referred to the rapid formation and collapse of bubbles and microstreaming currents caused by a series of compressions and rarefactions (Patist & Bates, Citation2008; Zheng & Sun, Citation2006). When ultrasound passes through a liquid medium, acoustic cavitation causes a series of physical and chemical process in liquids. In the food industry, it has been used in several dairy applications, including inactivation of enzymes and bacteria, homogenization and extraction of enzymes, as well as to alter the physical properties of gels made from milk (Frydenberg, Hammershøj, Andersen, Greve, & Wiking, Citation2016; Jayasooriya, Torley, D’Arcy, & Bhandari, Citation2007; Riener, Noci, Cronin, Morgan, & Lyng, Citation2009; Sánchez, Simal, Femenia, Llull, & Rosselló, Citation2001). β-Lactoglobulin via HIU treatment could significantly improve its functional properties and structure (Stanic-Vucinic et al., Citation2012).
β-Lactoglobulin, the most abundant protein component in cheese whey, represents about 60% of the total proteins. It is a globular protein with a diameter of approximately 4 nm, containing two disulfide bridges and one free thiol. β-Lactoglobulin has an isoelectric point (pI) of 4.8 and a molar mass of 18 kDa. It can easily form dimers and some larger oligomers depending on pH, temperature, and its concentration (Anema & De Kruif, Citation2014). As the major component of whey protein, β-Lactoglobulin is generally responsible for the functional properties of many dairy products. Bovine β-Lactoglobulin is widely applied to stabilize the food-related aerated system for its pronounced surface activity. Because of its distinct secondary and tertiary structure, it can be used in either native state or in the form of thermally aggregated particles (Dombrowski, Johler, Warncke, & Kulozik, Citation2016).
β-Lactoglobulin is one of the proteins in milk which exhibits antioxidant activity. Several studies have provided evidence on the antioxidant activity of β-Lactoglobulin. Allen and Wrieden (Citation1982) reported that β-Lactoglobulin contains a free sulfhydryl group (Cys121) partially buried within the protein core which is attributed to antioxidant activity. Elias, Mcclements, and Decker (Citation2005) reported that the presence of low concentrations of continuous phase β-Lactoglobulin significantly inhibited lipid oxidation. Hernándezledesma, Dávalos, Bartolomé, and Amigo (Citation2005) investigated that the peptide fragments obtained via enzymatic hydrolysis of α-Lactalbumin and β-Lactoglobulin could enhance antioxidant properties by calculating oxygen radical absorbance capacity (ORAC) values. Liu, Chen, and Mao (Citation2007) investigated that cross-linking the free thiol groups of β-Lactoglobulin by heating or chemically modifying β-Lactoglobulin by carboxymethylation resulted in a substantial loss of antioxidant activity. Stanic-Vucinic, Prodic, and Apostolovic (Citation2013) investigated that glycation of β-Lactoglobulin via Maillard reaction induced by high-intensity ultrasound could significantly improve its antioxidative capacity. Wu, Zhang, and Ren et al. (Citation2018) investigated the antioxidant activity and structure–affinity relationship of β-Lactoglobulin and 71 phenolic acids. However, studies on the antioxidative properties of β-Lactoglobulin treated by ultrasound treatment were very limited. The objectives of the present study were to investigate the effect of ultrasound treatment on antioxidant activity and structure of β-Lactoglobulin using response surface methodology.
Materials and methods
Materials and reagents
Raw bovine milk (nonfat solids ≥ 8.10%, protein 2.90%) was purchased from ChunGuang dairy co. LTD (Changchun, China). β-Lactoglobulin (≥ 90%, lyophilized powder), 1,1-Diphenyl-2-picrylhydrazyl (DPPH), 2,2ʹ-Azino-bis(3-ethylbenzothiazoline-6-sulfonic acid) diammonium salt (ABTS), 2,2-azo-bis (2-methylpropionamidine) dihydrochloride (AAPH), 2ʹ,7ʹ-dichlorofluorescin diacetate, 6-hydroxy-2,5,7,8-tetramethylchroman-2-carboxylicacid (trolox), and ethylenediamine tetraacetate were purchased from Sigma-Aldrich (St. Louis, MO, USA). Acetonitrile was purchased from Fisher Corporation (HPLC grade, USA); trifluoroacetic acid was purchased from Aladdin Corporation (HPLC grade, TFA, China). All other reagents were of analytical grade and supplied from Beijing Chemical Works (Beijing, China). A Milli-Q deionization reversed osmosis system (Millipore Corp., Bedford, MA, USA) was used to provide deionized water by filtering through syringe filter of 0.22 μm.
Preparation of β-Lactoglobulin
β-Lactoglobulin was prepared from raw bovine milk according to the methods with some modifications (Aschaffenburg & Drewry, Citation1957; Neyestani, Djalali, & Pezeshki, Citation2003). Raw bovine milk was warmed to about 40°C, and anhydrous Na2SO4 added with stirring. The temperature was down to 25°C until the salt had been dissolved. During the isolating process, the obtained filtrate was centrifuged (3000 × g at 4°C for 30 min) and the separated β-Lactoglobulin was obtained by filtrated through Whatman No. 4 filter paper. After dialysis, β-Lactoglobulin was obtained by freeze-drying at about 0.034 atm for 48 h. The purity of β-Lactoglobulin was analyzed by high performance liquid chromatography (HPLC).
β-Lactoglobulin solutions were prepared by dissolving the β-Lactoglobulin powder in deionized water to 1% (ω/v) and then stirred (2000 rpm) for 1 h at room temperature. Then, its pH was adjusted to 7.0 with NaOH solution (2 M) and stored at 4°C overnight. All solutions were filtered through syringe filter of 0.45 μm and equilibrated at room temperature before ultrasound treatment.
Ultrasound treatment
An Ultrasonic Processor (VCX 800, Vibra Cell, Sonics, USA) with a 13-mm high grade titanium alloy probe (amplitude, 114 μm) threaded to a 3 mm tapered microtip was used to treat about 15 mL β-Lactoglobulin solutions in centrifuge tubes. The samples (1%, ω/v) were treated with a frequency of 20 kHz, ultrasound intensity of 60 W/cm2, varying time from 10 to 30 min (10 s: 5 s work/rest cycles), amplitude (20%, 30%, and 40%), and temperature (40, 45, and 50°C). The temperature was controlled by an external constant temperature water bath apparatus. The intermittent ultrasound method was used for long time ultrasonic processing, to keep the temperature of samples. The probe was placed at the same distance from the base of liquid level for all samples treated (Mohod & Gogate, Citation2011).
Experimental design
Box–Behnken design (BBD) is one of the most common designs used in the Response Surface Measurment (RSM), which is a class of rotatable or nearly rotatable second-order designs based on three-level incomplete factorial designs. Compared with central composite design, BBD is often considered to be more relatively efficient. On the basis of the single factor experiments, three independent variables: ultrasonic temperature (40–50°C), ultrasonic time (0–30 min), and ultrasonic amplitude (20–40%) were applied in this study to determine the response pattern through BBD. The three variables X1, X2, and X3 were the coded variables for ultrasonic temperature, ultrasonic time, and ultrasonic amplitude, respectively, while the response value was the DPPH radical scavenging activity. The mathematics model for optimization of dependent variables was based on the following equation:
where Y is the observed response value predicted by the model; β0, βj, βjj, and βij are the regression coefficients for intercept, linearity, square, and interaction effect, respectively, Xi, Xj are independent coded variables (Neter, Wasserman, & Kutner, Citation1990). The goodness of fit model was evaluated by the coefficient R2. The whole experimental design, data analysis, and quadratic model building were accomplished using the Design Expert software (Trial Version 7.0.0, Stat-Ease Inc., Minneapolis, MN, USA).
High performance liquid chromatography
A HPLC unit (LC-20A, Shimadzu, Japan) with a reversed-phase analytical column C8 (Sepax Bio-C8, Sepax Technologies) with a silica-based packing (5 μm, 300 Å, 4.6 × 250 mm) was used for the separation. A gradient elution using solvents A (acetonitrile with 0.1% trifluoroacetic acid) and B (deionized water with 0.1% trifluoroacetic acid) was carried out according to the method of Bonfatti, Grigoletto, Cecchinato, Gallo, and Carnier (Citation2008) with some modifications. Separation was performed with linear gradient from 33% to 45% A in 35 min and return linearly to the starting condition in 1 min. The injection volume was 10 μL and the flow rate was 0.5 mL/min. The cell temperature was kept at 40°C and the detection was made at a wavelength of 214 nm.
DPPH radical scavenging activity assay
The DPPH radical scavenging activity of β-Lactoglobulin was performed by the method proposed by Chen, Tsai, Huang, and Chen (Citation2009) with slight modifications. A known value of 150 μL of β-Lactoglobulin solution at different concentrations (1–10 mg/mL) was added to 150 μL of the DPPH solution (0.2 mM). After incubation at room temperature in the dark for 30 min, the absorbance was measured at 517 nm on a spectrophotometer (UV-2550, Shimadzu, Kyoto, Japan). The control was prepared in the same manner except that deionized water was used instead of β-Lactoglobulin samples and DPPH solution. The DPPH radical scavenging activity was calculated as follows:
where Ab: blank group, the absorbance of ethyl alcohol and deionized water; AS: sample group, the absorbance of β-Lactoglobulin solution and DPPH solution; Ac: control group, the absorbance of deionized water and DPPH solution.
ABTS radical scavenging activity assay
The ABTS radical scavenging activity of β-Lactoglobulin was performed by the method of Hernándezledesma et al. (Citation2005) with minor modifications. ABTS was produced by reaction of 2.45 mM potassium persulfate and 7 mM ABTS solution at room temperature in the dark for 12 h before use. The ABTS solution was adjusted to an absorbance of 0.70 ± 0.02 at 734 nm by dilution with 5 mM phosphate buffered solution. A known value of 50 μL of β-Lactoglobulin solution at different concentrations (0.5–10 mg/mL) was added to 100 μL of the diluted ABTS solution. After incubation at room temperature in the dark for 1 h, the absorbance was measured at 734 nm. The control was prepared in the same manner except that deionized water was used instead of β-Lactoglobulin samples and ABTS solution. The ABTS radical scavenging activity was calculated as follows:
where Ab: blank group, the absorbance of deionized water; AS: sample group, the absorbance of β-Lactoglobulin solution and ABTS solution; Ac: control group, the absorbance of deionized water and ABTS solution.
ORAC assay
ORAC assay was carried out following the method described by Boxin, Hampschwoodill, and Prior (Citation2001) and Davalos, Gomez-Cordoves, and Bartolome (Citation2004) with slight modification. All substances were dissolved and diluted solely in sodium phosphate buffer (75 mM, pH 7.4). A known value of 20 μL of β-Lactoglobulin samples (0.1 mg/mL) was added to 120 μL of fluorescein solution (70 nM) in black 96-well microplate. After incubation at 37°C in the dark for 2 min, 60 μL of AAPH (12 mM) was added to the mixture. The fluorescence was recorded every 2 min using a microplate reader with the excitation wavelength of 485 nm and the emission wavelength of 520 nm for 3 h. The control was prepared in the same manner except that sodium phosphate buffer (75 mM, pH 7.4) was used instead of β-Lactoglobulin samples. Fluorescence measurements were normalized to the curve of the blank. From the normalized curves, the area under the fluorescence decay curve (AUC) was calculated as follows:
where f0 and fi were the fluorescence reading at time 0 and i min, respectively.
The netAUC corresponding to a sample was calculated as follows:
The antioxidant ORAC value was calculated by a linear regression analysis between netAUC and antioxidant concentration and expressed as μM TE/g sample.
Differential scanning calorimetry
The effect of ultrasound treatment on thermal properties of β-Lactoglobulin was evaluated using a differential scanning calorimeter (DSC; Q2000, TA Instrument, USA). Approximately 1 mg of β-Lactoglobulin solution (1%, ω/v) was placed in aluminium pans and then sealed. An empty pan was used as a reference. Each pan was heated up to 120°C under flow of dry nitrogen gas at 50 mL/min using a heating rate of 5°C/min. The onset temperature (To), peak temperature (Tp), ceased temperature (Tc), and enthalpy (ΔH) were then recorded for each sample.
Circular dichroism spectroscopy
The effect of ultrasound treatment on the structure of β-Lactoglobulin was measured using a circular dichroism (CD) spectropolarimeter (MOS-500, Bio-logic, France). The measurement was conducted using 2 mm quartz CD cuvette with β-Lactoglobulin samples at room temperature. The CD spectral scanning meter was recorded in the far-UV region (190–250 nm) and the near-UV region (250–320 nm) with a slit of 2 nm (Cheng et al., Citation2017), and the scan rate was 60 nm/min (Adato, Aksu, & Altug, Citation2015). Each scan was performed three times. The proportion of four different secondary structures (a-helix, β-sheet, β-turn, random coil) was calculated using the CD Pro software.
Scanning electron microscopy
Morphological changes of β-Lactoglobulin after treatment by ultrasound were performed using scanning electron microscopy (SEM; Hitachi S-3400N) with accelerating voltage of 5 kV. The samples were prepared for SEM examination according to the method reported previously (Jiang et al., Citation2014). The samples were dehydrated in ethanol, coated with an ion sputtering apparatus (Hitachi E-1010), and examined at a magnification of 4000× at a distance of 6 mm.
Statistical analysis
All measurements were performed in triplicate. The significant differences of data among samples were calculated using SPSS Version 11.5 (SPSS Inc., Chicago). Data were checked for homogeneity by Levene’s test. One-way analysis of variance (ANOVA) and then a least squared differences model were applied when the data were homogeneous. The Dunnett’s test was used when the data were heterogeneous. All the figures were drawn by origin 8.0 (OriginLab Corporation, Northampton, USA). All the data were presented as mean ± standard deviation (SD). Differences were considered as significant.
Results and discussion
The HPLC chromatography of β-Lactoglobulin
Full spectrum scanning () showed that the 214 nm was the best detection wavelength for β-Lactoglobulin (Bonfatti et al., Citation2008). The elution gradient of C8 column was set according to the method reported by Kong and Wang (Citation2012). The β-Lactoglobulin samples (≥ 90%) diluted to the concentration of 0.1–0.5 mg/mL were detected. The regression coefficients of concentration vs. peak area equation were 0.9987, indicating the equation analog effect was acceptable. The purity of β-Lactoglobulin isolated from raw cow milk was calculated to be 88% and 91% based on the linear regression equation.
Figure 1. HPLC chromatographic of β-Lactoglobulin. (A) β-Lactoglobulin (standard), (B) β-Lactoglobulin (untreated), (C) ultrasound-treated 45°C—20 min—amplitude (AP) 30%, (D) ultrasound-treated 45°C—10 min—AP 20%, (E) ultrasound-treated 45°C—30 min—AP 40%.
Figura 1. Cromatografía HPLC de β-lactoglobulina. (A) β-lactoglobulina (Estándar), (B) β-lactoglobulina (Sin tratamiento), (C) Con tratamiento de ultrasonido 45°C – 20 min – Amplitud (AP) 30%, (D) Con tratamiento de ultrasonido 45°C – 10 min – AP 20%, (E) Con tratamiento de ultrasonido 45°C – 30 min – AP 40%.
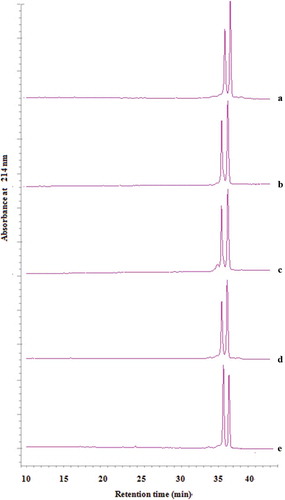
As shown in , the two large peaks eluting at 35 and 37 min represent β-Lactoglobulin A and B variants, respectively. The HPLC chromatography of ultrasound-treated β-Lactoglobulin was similar with the untreated β-Lactoglobulin. Ultrasound treatment seemed had no obviously effect on the peaks eluting of β-Lactoglobulin compared with the untreatedβ-Lactoglobulin.
Effect of temperature, time, and amplitude on the DPPH radical scavenging activity
The untreated β-Lactoglobulin had shown a weak DPPH radical scavenging activity (). showed that the DPPH radical scavenging activity increased significantly when the ultrasonic temperature changed from 40 to 50°C, the maximum DPPH radical scavenging activity was 38.77 ± 0.98% at 50°C, but decreased remarkably above 50°C. It may be due to the protein tended to be denatured when the ultrasonic temperature is too high. showed that the DPPH radical scavenging activity increased and then decreased as ultrasonic time increased. At 20 min, the maximum DPPH radical scavenging activity was 48.32 ± 0.06%. It may be due to changes in the space conformation of β-Lactoglobulin induced by ultrasound treatment.
Figure 2. Effect of three factors on the DPPH radical scavenging activity of β-Lactoglobulin. The concentration of untreated and ultrasound-treated β-Lactoglobulin was 1% (ω/v). (a) Ultrasound-treated 20 min, AP 30%, varying temperature from 40 to 55°C, (b) ultrasound-treated 45°C, AP 30%, varying time from 10 to 30 min, (c) ultrasound-treated 45°C, 20 min, varying amplitude from 20% to 40%.
Figura 2. Efecto de los tres factores en la actividad de eliminación de radicales de DPPH de la β-lactoglobulina. La concentración de β-lactoglobulina sin tratamiento y con tratamiento de ultrasonido fue 1% (ω/v). (a) Con tratamiento de ultrasonido 20 min, AP 30%, variando la temperatura de 40 a 55°C, (b) Con tratamiento de ultrasonido 45°C, AP 30%, variando la duración de 10 a 30 min, (c) Con tratamiento de ultrasonido 45°C, 20 min, variando la amplitud de 20 a 40%.
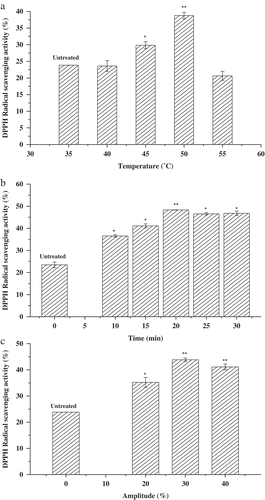
With the extension of ultrasonic time, some of the active sites of protein were destroyed gradually, as well as the effect of transient thermal decreased the antioxidation capacity of β-Lactoglobulin. showed that the DPPH radical scavenging activity first increased and then decreased with the ultrasonic amplitude increasing. The maximum DPPH radical scavenging activity was 43.82 ± 0.67% at amplitude of 30%. With the increase of amplitude, the increasing acoustic energy could break up the agglomerates of β-Lactoglobulin, which contributed to the scavenging abilities of β-Lactoglobulin against free radicals. Based on the above data, the factors of ultrasonic temperature (40–50°C), ultrasonic time (10–30 min), and ultrasonic amplitude (20–40%) were selected for further BBD.
Model fitting and response surface analysis
The experimental design and the response of DPPH radical scavenging activity obtained for each experiment along with the predicted values were listed in . The adjusted R2 and predicted R2 were calculated to check the adequacy of the model. The significance of the model was calculated with analysis of F ratio and P value.
Table 1. Box-Behnken design and its responses.
Tabla 1. Diseño Box-Behnken y sus respuestas.
The mathematical model, representing the effect of three factors on the response values of DPPH radical scavenging activity, can be described by the following quadratic Equation (6). Y is the DPPH radical scavenging activity, X1, X2, and X3 are the coded variables for temperature, time, and amplitude, respectively. The adequacy of a fitted-response surface model was statistically evaluated based on the coefficient of determination (R2) and the ANOVA results as shown in . The F-values (839.7237 for the three factors) were greater than 0.001. The P-value of the response model was significant (p < 0.0001) which mean that only 0.01% of the total variation cannot be explained by the model because of noise. The lack of fit F-values (p = 3. 3687 > 0.05) showed no statistical significant, and the models can be successfully used for the prediction, because the lack of fit is insignificant. The R2 value was 0.9993, which was in good agreement with the Pred R2 as 0.9909, indicating that the models could be successfully used to predict the DPPH radical scavenging activity in the experimental range. The adjusted R2 value was 0.9981, meaning that only 0.19% of the total variation in the model is not explained by the input variables (Daneshvand, Ara, & Raofie, Citation2012). The P-value of X2, X3, X1X3, X2X3 were 0.0005, 0.0003, 0.0126, 0.0006, which were significant in model terms.
Table 2. Variance analysis of DPPH radical scavenging activity.
Tabla 2. Análisis de varianza de la actividad de eliminación de radicales de DPPH.
(a–c) represents the combined effects of independent variables on the DPPH radical scavenging activity. showed the interaction between ultrasonic time and ultrasonic amplitude affected the DPPH radical scavenging activity significantly (p < 0.05). Up to 45°C, the DPPH radical scavenging activity of β-Lactoglobulin increased slightly in the early stage, within the range of amplitude applied in the experiment, the DPPH radical scavenging activity of β-Lactoglobulin increased from 45.91% to 57.59%, and then decreased to 46.07% with ultrasonic time prolonged. showed the interaction between ultrasonic temperature and ultrasonic amplitude affected the DPPH radical scavenging activity significantly (p < 0.05). At 20 min, within the range of temperature applied in the experiment, the DPPH radical scavenging activity of β-Lactoglobulin first increased from 45.55% to 57.32%, and then decreased to 46.27% with ultrasonic amplitude increasing. Ultrasonic amplitude seemed to play more role in these changes. The ultrasonic temperature was not significantly affected the DPPH radical scavenging activity of β-Lactoglobulin, but the interaction of ultrasonic time or ultrasonic amplitude was significantly affected, indicating the optimal ultrasound treatment could increase the antioxidant capacity by changing the structure of protein.
Figure 3. Response surface plots showing the effect of three factors and their interactions on the DPPH radical scavenging activity of β-Lactoglobulin. (a) Ultrasonic temperature and ultrasonic time, (b) ultrasonic amplitude and ultrasonic time, (c) ultrasonic amplitude and ultrasonic temperature.
Figura 3. Diagramas de superficies de respuesta que constatan los efectos de los tres factores y sus interacciones en la actividad de eliminación de radicales de DPPH de la β-lactoglobulina. (a) Temperatura ultrasónica y tiempo ultrasónico, (b) Amplitud ultrasónica y tiempo ultrasónico, (c) Amplitud ultrasónica y temperatura ultrasónica.
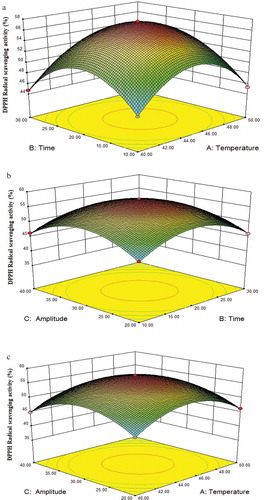
The maximum experimental value of the DPPH radical scavenging activity was 57.59% under the conditions of experiment 15 (45°C, 20 min, AP 30%). The homologous predict value of the DPPH radical scavenging activity was 57.48%. It was consisted with the observed experimental responses according to the models. The experimental data were in the confidence interval with no abnormal data. The results indicated that the ultrasound-treated β-Lactoglobulin under optimized conditions showing significantly (p < 0.05) higher radical scavenging activity (p < 0.05). Similar results obtained by Stanic-Vucinic et al. (Citation2013).
The ABTS radical scavenging activity of β-Lactoglobulin
The ABTS radical scavenging activity assay was carried out to further investigate the effect of ultrasound treatment on antioxidant capacity of β-Lactoglobulin. Based on the BBD design, we selected three β-Lactoglobulin samples of experimental 10 (ultrasound-treated 45°C, 30 min, AP 40%), 12 (ultrasound-treated 45°C, 10 min, AP 20%), and 13 (ultrasound-treated 45°C, 20 min, AP 30%) to compare the antioxidant capacity of β-Lactoglobulin. The ABTS radical scavenging activity of untreated and ultrasound-treated β-Lactoglobulin was shown in , native β-Lactoglobulin showed ABTS radical scavenging activity of 19.52 ± 0.13%, 20.40 ± 0.13%, 21.40 ± 0.24%, and 30.60 ± 0.13% at concentrations from 1 to 10 mg/mL, respectively. The results indicated that the antioxidant activity of β-Lactoglobulin was improved which was associated with the concentration of β-Lactoglobulin pretreatment. Ultrasound treatment improved the ABTS radical scavenging activity of β-Lactoglobulin with the highest value of 61.87 ± 0.06% at 10 mg/mL (sample C). The ABTS radical scavenging activity increased with the increasing of ultrasonic time and ultrasonic amplitude (p < 0.05), while the ultrasonic time was above 20 min or ultrasonic amplitude was 40%, the ABTS radical scavenging activity decreased gradually. The results showed that the antioxidant activity of β-Lactoglobulin depended not only on the concentration, but also on the ultrasonic conditions. The antioxidant activity of β-Lactoglobulin may be contributed by the sulfhydryl (-SH) that exists on the surface of β-Lactoglobulin (Li, Ma, & Ngadi, Citation2013). Ultrasound treatment could increase the specific surface area and break up the agglomerates of β-Lactoglobulin (Yan, Wang, Ma, & Wang, Citation2016). When the ultrasonic time or ultrasonic amplitude was too high, the active parts of protein and the molecular conformation of protein would be destroyed, so the ABTS radical scavenging activity of β-Lactoglobulin would be decreased. The results from the ABTS radical scavenging activity were in good agreement with those results obtained from the DPPH radical scavenging activity.
ORAC of β-Lactoglobulin
The ORAC assay was used to further estimate the effect of ultrasound treatment on the antioxidant capacity of β-Lactoglobulin. The ORAC assay was estimated through the donation of hydrogen atom and breaking the radical chains based on the inhibition of peroxyl radical-induced oxidations (Prior, Wu, & Schaich, Citation2005). The ORAC results were shown in . The trolox standard curve was plotted between 0 and 20 μM, and its NetAUC standard equation was y = 7.3 x −6.7 (R2 = 0.996). The decay curves of inhibition on fluorescence by ultrasound-treated β-Lactoglobulin were higher than untreated β-Lactoglobulin. The ORAC value of standard β-Lactoglobulin and untreated β-Lactoglobulin were 3.71 ± 0.43 and 3.59 ± 0.13 TE/μM, respectively. With the increase of ultrasonic time and ultrasonic amplitude, the fluorescence quenching time was prolonged, and its antioxidation was improved. The results showed that ultrasound-treated β-Lactoglobulin exhibited amplitude-dependent increase in the inhibition of fluorescein decay. When the β-Lactoglobulin ultrasound treated at amplitude of 40%, the ORAC value was 17.28 ± 0.18 TE/μM, which was increased five times than untreated β-Lactoglobulin (3.59 ± 0.13 TE/μM). The fluorescence intensity was maintained at a certain time, and the fluorescence quenching time was significantly prolonged (p < 0.05). Overall, ultrasound treatment increased the ORAC value of β-Lactoglobulin and its effect was more pronounced at high amplitude. β-Lactoglobulin contained a free sulfhydryl group and two disulfide bonds in its hydrophobic core, which contributed to the scavenging abilities of β-Lactoglobulin against free radicals (Sakai, Sakurai, Sakai, Hoshino, & Goto, Citation2000). The antioxidant activity of β-Lactoglobulin increased with increasing ultrasonic amplitude due to the inter- and intra-molecular hydrogen bonds partly destroyed.
Figure 5. The decay curve and oxygen radical absorbance capacity of inhibition on fluorescence by ultrasound-treated β-Lactoglobulin. (a) Decay curve of β-Lactoglobulin, (b) oxygen radical absorbance capacity of β-Lactoglobulin.
Figura 5. Curva de decaimiento y capacidad de absorción de radicales de oxígeno de inhibición en fluorescencia por la β-lactoglobulina con tratamiento de ultrasonido. (a) Curva de decaimiento de la β-lactoglobulina, (b) Capacidad de absorción de radicales de oxígeno (CARO) de la β-lactoglobulina.
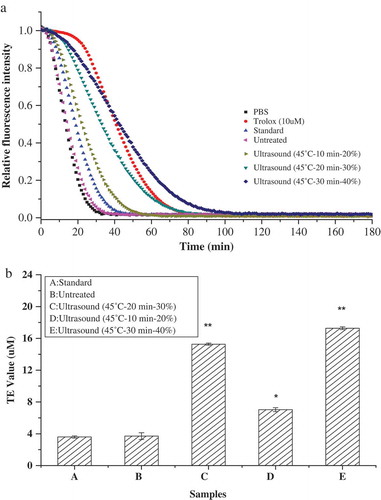
Thermal properties analysis by DSC
DSC has been used to study the thermal stability of proteins by measuring the heat amount associated with the molecule’s thermal denaturation (Konieczny et al., Citation2016). Enthalpy (ΔH) and transition temperatures (To, Tp, Tc) involved in the denaturation process of β-Lactoglobulin were analyzed by DSC. As shown in , the results showed that the transition temperature (To, Tp, Tc) of untreated β-Lactoglobulin was 71.08 ± 2.78°C, 92.56 ± 4.04°C, and 109.62 ± 3.23°C, respectively. It was similarly reported by ElMaksoud Abd et al. (Citation2018). Ultrasound treatment destroyed the stability of the tertiary structure of β-Lactoglobulin since the thermal denaturation started around 95–103°C, compared to untreated β-Lactoglobulin at 92°C. The enthalpy (∆H) increased significantly (p < 0.05) after ultrasound treatment for 20 or 30 min at the amplitude of 20% or 40%. There was significant difference between the untreated and ultrasound-treated samples (p < 0.05). When the β-Lactoglobulin solutions were heated from 20 to 120°C, the enthalpy increased with the increasing ultrasonic amplitude from 20% to 40% and prolonging ultrasonic time from 10 to 30 min, and it suggested the potential denaturation and aggregation due to the layer of hydration lost (Shen, Fang, Gao, & Guo, Citation2017).
Table 3. Enthalpy and transition temperatures of untreated and ultrasound-treated β-Lactoglobulin solution (1%, ω/v).
Tabla 3. Entalpía y temperaturas de transición de una solución (1%, ω/v) de β-lactoglobulina sin tratamiento y con tratamiento de ultrasonido.
Table 4. Secondary structure of β-Lactoglobulin untreated and ultrasound treated (0.05 mg/mL).
Tabla 4. Estructura secundaria de β-lactoglobulina sin tratamiento y con tratamiento de ultrasonido (0.05 mg/mL).
Measurement of CD spectroscopy
The secondary and tertiary structure of untreated and ultrasound-treated β-Lactoglobulin solutions were analyzed using CD spectroscopy (). Far UV-CD measurement was carried out to characterize the secondary structure changes of untreated and ultrasound-treated β-Lactoglobulin (). The secondary structural contents of untreated and ultrasound-treated β-Lactoglobulin were shown in . The estimated secondary structure of standard β-Lactoglobulin contained 16.9% α-helix, 38.0% β-sheet, 17.4% β-turn, and 27.7% random coil; untreated β-Lactoglobulin solution contained 21.8% α-helix, 33.3% β-sheet, 16.7% β-turn, and 28.2% random coil. Compared with untreated β-Lactoglobulin, ultrasound-treated β-Lactoglobulin showed an increase in the α-helix and β-sheet proportion, but a decrease in the random coil proportion (Aouzelleg et al., Citation2004). The CD spectra of β-Lactoglobulin indicated that the protein exhibited a broad negative peak at about 218 nm at different ultrasound treatment conditions, which is characteristic of a protein with well-defined antiparallel β-sheet. The result was consistent with the previous study (Agyare, & Damodaran, Citation2010; Cheng et al., Citation2017; Stanic-Vucinic et al., Citation2013). With the increase of amplitude, the contents of β-sheet increased gradually, when the amplitude reached 40%, the proportions of β-structure reached the maximum. It may be due to the acoustic energy which increased with ultrasonic amplitude. Vivian and Callis (Citation2001) reported that ultrasound-treated whey protein (20 kHz, 450W) showed an increase in the α-helix component but a decrease in the β-sheet component. Stathopulos et al. (Citation2004) confirmed ultrasound treatment changed protein secondary structure significantly and induce protein aggregates to exhibit a high β-structure content but low α-structure content after ultrasonication. Hu et al. (Citation2013) indicated that higher sonication power combined with longer time could increase the random coil and β-turn components of soy protein isolate (SPI).
Figure 6. CD spectroscopy of untreated and ultrasound-treated β-Lactoglobulin. (a) The far UV-CD spectra of untreated and ultrasound-treated β-Lactoglobulin (0.05%, ω/v), (b) the near UV-CD spectra of untreated and ultrasound-treated β-Lactoglobulin (1%, ω/v).
Figura 6. Espectroscopía CD de β-lactoglobulina sin tratamiento y con tratamiento de ultrasonido. (a) Espectro UV-CD lejano de β-lactoglobulina sin tratamiento y con tratamiento de ultrasonido (0.05%, ω/v), (b) Espectro UV-CD cercano de β-lactoglobulina sin tratamiento y con tratamiento de ultrasonido (1%, ω/v).
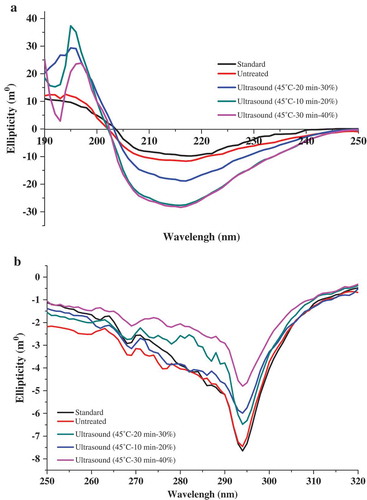
Near UV-CD measurement was carried out to investigate the tertiary structure changes of untreated and ultrasound-treated β-Lactoglobulin (). The spectra of β-Lactoglobulin indicated that the protein exhibited a broad negative peak at about 290 nm at different ultrasound treatment conditions, which is attributed to the Trp residues. With the increase of amplitude, the maximum absorption peak around 290 nm displayed a red shift and a decrease in ellipticities, suggesting the alterations of Trp residues and the tertiary structure of β-Lactoglobulin had been significantly loosened by ultrasound treatment.
The data of CD spectroscopy analysis indicated that ultrasound treatment increased both α-helix and β-sheet contents of β-Lactoglobulin and altered Trp residues, which resulted in the secondary and tertiary structure changes. It was possibly induced by the interactions of local sequence stretches of amino acids and hydrogen bonds. The changes in structure were associated with the antioxidant capacity.
Microstructure analysis by SEM
Microstructures of β-Lactoglobulin untreated and ultrasound treated were shown in . The SEM images were obtained at 4000× magnification. As the SEM images showed, the ultrasound-treated β-Lactoglobulin exhibited more disordered structures and irregular fragments compared with untreated. The sample C (Ultrasound-treated 45°C, 20 min, AP 30%) was smaller than other samples after ultrasound treatment, which led to protein aggregation and network formation. The sample D and E were more uniform than other samples, suggesting that exposure to medium ultrasonic power could generate large uniform structure; it might be caused by the unfolding of proteins and increasement of surface hydrophobic groups of the β-Lactoglobulin molecules. Overall, the changes in microstructure of β-Lactoglobulin might have been generated because of the cavitational forces exerted by the probe during ultrasonic treatment and because of micro streaming and turbulent forces (Jambrak et al., Citation2012).
Figure 7. Microstructure of β-Lactoglobulin untreated and ultrasound treated determined by scanning electron microscopy. (a) β-Lactoglobulin (standard), (b) β-Lactoglobulin (untreated), (c) ultrasound-treated 45°C—20 min—AP 30%, (d) ultrasound-treated 45°C—10 min—AP 20%, (e) ultrasound-treated 45°C—30 min—AP 40%.
Figura 7. Microestructura de la β-lactoglobulina sin tratamiento y con tratamiento de ultrasonido determinada por el microscopio electrónico de barrido. (a) β-lactoglobulina (estándar), (b) β-lactoglobulina (Sin tratamiento), (c) Con tratamiento de ultrasonido 45°C – 20 min – AP 30%, (d) Tratamiento de ultrasonido 45°C – 10 min – AP 20%, (e) Tratamiento de ultrasonido 45°C – 30 min – AP 40%.
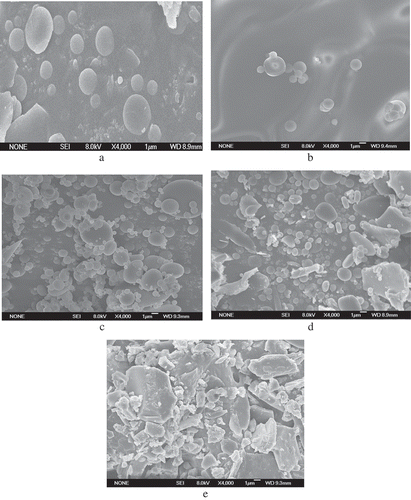
Conclusions
The results indicated that ultrasound treatment had considerable impact on the antioxidant activity and structure of β-Lactoglobulin. The maximal DPPH radical scavenging activity of β-Lactoglobulin was 57.59% at the conditions of 45°C, 20 min and amplitude of 30%. The results of ABTS radical scavenging activity and ORAC were both increased after ultrasound treatment. Ultrasound treatment can change the structure of β-Lactoglobulin by altering β-structures, α-helical structures, and Trp residues from CD data. SEM and DSC data revealed that ultrasonicated β-Lactoglobulin contained larger aggregates than untreated samples. Ultrasound treatment can be used to improve antioxidant activities of β-Lactoglobulin through varying the ultrasound treatment conditions. Amplitude seemed to play more role in this change, the antioxidant activities and structure of β-Lactoglobulin changed obviously with the increase of ultrasonic amplitude. Under the optimized ultrasonic conditions, the antioxidant activities could be increased significantly. These studies provide a theoretical basis for the improvement of antioxidant activity of β-Lactoglobulin. Further studies should be conducted to investigate the effect of ultrasound treatment on the antioxidant mechanism of β-Lactoglobulin using antioxidant index by cellular methods, as well as other potential aspects of functional characteristics. β-Lactoglobulin modified by ultrasound treatment could be explored as a natural antioxidant agent or a promising natural antibacterial agent applied in functional food industries and medical proposes.
Acknowledgments
The study was financially supported by the Ministry of Science and Technology of China (Project# 2013BAD18B07).
Disclosure statement
No potential conflict of interest was reported by the authors.
Additional information
Funding
References
- Adato, R., Aksu, S., & Altug, H. (2015). Engineering mid-infrared nanoantennas for surface enhanced infrared absorption spectroscopy. Materials Today, 18, 436–446.
- Agyare, K. K., & Damodaran, S. (2010). pH-stability and thermal properties of microbial transglutaminase-treated whey protein isolate. Journal of Agricultural & Food Chemistry, 58, 1946–1953.
- Allen, J. C., & Wrieden, W. L. (1982). Influence of milk-proteins on lipid oxidation in aqueous emulsion. 1. Casein, whey-protein and alpha-lactalbumin. The Journal of Dairy Research, 49(2), 239–248.
- Anema, S. G., & De Kruif, C. G. (2014). Complex coacervates of lactotransferrin and beta-lactoglobulin. Journal of Colloid and Interface Science, 430, 214–220.
- Aouzelleg, A., Bull, L. A., Price, N. C., & Kelly, S. M. (2004). Molecular studies of pressure/temperature-induced structural changes in bovine beta-lactoglobulin. Journal of the Science of Food and Agriculture, 84, 398–404.
- Aschaffenburg, R., & Drewry, J. (1957). Improved method for the prepration of crystalline β-lactoglobulin and α-lactalbumin from cow’s milk. Biochemical Journal, 65, 273–277.
- Bonfatti, V., Grigoletto, L., Cecchinato, A., Gallo, L., & Carnier, P. (2008). Validation of a new reversed-phase high-performance liquid chromatography method for separation and quantification of bovine milk protein genetic variants. Journal of Chromatography A, 1195, 101–106.
- Boxin, O., Hampschwoodill, M., & Prior, R. L. (2001). Development and validation of an improved oxygen radical absorbance capacity assay using fluorescein as the fluorescent probe. Journal of Agricultural & Food Chemistry, 49, 4619–4626.
- Chandrapala, J., Oliyer, C., Kentish, S., & Ashokkumar, M. (2012). Ultrasonics in food processing. Ultrasonics Sonochemistry, 19, 975–983.
- Chen, S. K., Tsai, M. L., Huang, J. R., & Chen, R. H. (2009). In vitro antioxidant activities of low-molecular-weight polysaccharides with various functional groups. Journal of Agricultural & Food Chemistry, 57, 2699–2704.
- Cheng, J., Liu, J. H., Prasanna, G., & Jing, P. (2017). Spectrofluorimetric and molecular docking studies on the interaction of cyanidin-3-O-glucoside with whey protein, β-lactoglobulin. International Journal of Biological Macromolecules, 105, 965–972.
- Daneshvand, B., Ara, K. M., & Raofie, F. (2012). Comparison of supercritical fluid extraction and ultrasound-assisted extraction of fatty acids from quince (Cydonia oblonga Miller) seed using response surface methodology and central composite design. Journal of Chromatography A, 1252, 1–7.
- Davalos, A., Gomez-Cordoves, C., & Bartolome, B. (2004). Extending applicability of the oxygen radical absorbance capacity (orac−fluorescein) assay. Journal of Agricultural & Food Chemistry, 52, 48–54.
- Dombrowski, J., Johler, F., Warncke, M., & Kulozik, U. (2016). Correlation between bulk characteristics of aggregated β-lactoglobulin and its surface and foaming properties. Food Hydrocolloids, 61, 318–328.
- Elias, R. J., Mcclements, D. J., & Decker, E. A. (2005). Antioxidant activity of cysteine, tryptophan, and methionine residues in continuous phase beta-lactoglobulin in oil-in-water emulsions. Journal of Agricultural & Food Chemistry, 53, 10248–10253.
- ElMaksoud Abd, A. A., ElGhany Abd, I. H., ElBeltagi, H. S., Anankanbil, S., Banerjee, C., Petersen, S. V., ... Guo, Z. (2018). Adding functionality to milk-based protein: Preparation, and physico-chemical characterization of β-lactoglobulin-phenolic conjugates. Food Chemistry, 241, 281–289.
- Frydenberg, R. P., Hammershøj, M., Andersen, U., Greve, M. T., & Wiking, L. (2016). Protein denaturation of whey protein isolates (wpis) induced by high intensity ultrasound during heat gelation. Food Chemistry, 192, 415–423.
- Hernándezledesma, B., Dávalos, A., Bartolomé, B., & Amigo, L. (2005). Preparation of antioxidant enzymatic hydrolysates from alpha-lactalbumin and beta-lactoglobulin. identification of active peptides by hplc-ms/ms. Journal of Agricultural & Food Chemistry, 53, 588–593.
- Hu, H., Wu, J. H., Li-Chan, E. C. Y., Zhu, L., Zhang, F., Xu, X. Y., … Pan, S. Y. (2013). Effects of ultrasound on structural and physical properties of soy protein isolate (SPI) dispersions. Food Hydrocolloids, 30, 647–655.
- Jambrak, A. R., Lerda, D., Mirčeta, R., Šimunek, M., Lelas, V., Chemat, F., ... Batur, V.. (2012). Experimental design and optimization of ultrasound treatment: Functional and physical properties of sonicated ice cream model mixtures. Journal of Food Processing & Technology, 71, 145–148.
- Jayasooriya, S. D., Torley, P. J., D’Arcy, B. R., & Bhandari, B. R. (2007). Effect of high power ultrasound and ageing on the physical properties of bovine Semitendinosus and Longissimus muscles. Meat Science, 75, 628–639.
- Jiang, L., Wang, J., Li, Y., Wang, Z., Liang, J., Wang, R., ... Zhang, M. (2014). Effects of ultrasound on the structure and physical properties of black bean protein isolates. Food Research International, 62, 595–601.
- Kong, Q., & Wang, M. (2012). Positive solutions of nonlinear fractional boundary value problems with dirichlet boundary conditions. Electronic Journal of Qualitative. Theory of Differential Equations, 63, 1–13.
- Konieczny, P., Tomaszewska-Gras, J., Andrzejewski, W., Mikołajczak, B., Urban’ska, M., Mazurkiewicz, J., & Stangierski, J. (2016). DSC and electrophoretic studies on protein denaturation of Anodonta woodiana (Lea, 1834). Journal of Thermal Analysis and Calorimetry, 126, 69–75.
- Li, M., Ma, Y., & Ngadi, M. O. (2013). Binding of curcumin to beta-lactoglobulin and its effect on antioxidant characteristics of curcumin. Food Chemistry, 141, 1504–1511.
- Liu, H. C., Chen, W. L., & Mao, S. J. (2007). Antioxidant nature of bovine milk beta-lactoglobulin. Journal of Dairy Science, 90, 547–555.
- Mohod, A. V., & Gogate, P. R. (2011). Ultrasonic degradation of polymers: Effect of operating parameters and intensification using additives for carboxymethyl cellulose (CMC) and polyvinyl alcohol (PVA). Ultrasonics Sonochemistry, 18, 727–734.
- Neter, J., Wasserman, W., & Kutner, M. H. (1990). Applied linear statistical models: Regression, analysis of variance, and experimental designs (3rd ed.). Burr Ridge, Ill: R.D. Irwin.
- Neyestani, T. R., Djalali, M., & Pezeshki, M. (2003). Isolation of alpha-lactalbumin, beta-lactoglobulin, and bovine serum albumin from cow’s milk using gel filtration and anion-exchange chromatography including evaluation of their antigenicity. Protein Expression and Purification, 29, 202–208.
- Patist, A., & Bates, D. (2008). Ultrasonic innovations in the food industry: From the laboratory to commercial production. Innovative Food Science & Emerging Technologies, 9, 147–154.
- Prior, R. L., Wu, X., & Schaich, K. (2005). Standardized methods for the determination of antioxidant capacity and phenolics in foods and dietary supplements. Journal of Agricultural and Food Chemistry, 53, 4290–4302.
- Riener, J., Noci, F., Cronin, D. A., Morgan, D. J., & Lyng, J. G. (2009). The effect of thermosonication of milk on selected physicochemical and microstructural properties of yoghurt gels during fermentation. Food Chemistry, 114, 905–911.
- Sakai, K., Sakurai, K., Sakai, M., Hoshino, M., & Goto, Y. (2000). Conformation and stability of thiol-modified bovine beta-lactoglobulin. Protein Science: A Publication of the Protein Society, 9, 1719–1729.
- Sánchez, E. S., Simal, S., Femenia, A., Llull, P., & Rosselló, C. (2001). Proteolysis of Mahon cheese as affected by acoustic-assisted brining. European Food Research and Technology, 212, 147–152.
- Shen, X., Fang, T. Q., Gao, F., & Guo, M. R. (2017). Effects of ultrasound treatment on physicochemical and emulsifying properties of whey proteins pre-and post-thermal aggregation. Food Hydrocolloids, 63, 668–676.
- Stanic-Vucinic, D., Stojadinovic, M., Atanaskovic-Markovic, M., Ognjenovic, J., Grönlund, H., van Hage, M., Lantto, R., ... Velickovic, T. C. (2012). Structural changes and allergenic properties of β-lactoglobulin upon exposure to high-intensity ultrasound. Molecular Nutrition & Food Research, 56, 1894–1905.
- Stanic-Vucinic, D., Prodic, I., & Apostolovic, D. (2013). Structure and antioxidant activity of beta-lactoglobulin-glycoconjugates;obtained by high-intensity-ultrasound-induced maillard reaction in;aqueous model systems under neutral conditions. Food Chemistry, 138, 590–599.
- Stathopulos, P. B., Scholz, G. A., Hwang, Y. M., Rumfeldt, J. A. O., Lepock, J. R., & Meiering, E. M. (2004). Sonication of proteins causes formation of aggregates that resemble amyloid. Protein Science, 13, 3017–3027.
- Vivian, J. T., & Callis, P. R. (2001). Mechanisms of tryptophan fluorescence shifts in proteins. Biophysical Journal, 80, 2093–2109.
- Wu, S., Zhang, Y., Ren, F., Qin, Y., Liu, J., Liu, J., ... Zhang, H. (2018). Structure-affinity relationship of the interaction between phenolic acids and their derivatives and Î2-lactoglobulin and effect on antioxidant activity. Food Chemistry, 245, 613–619.
- Yan, J. K., Wang, Y. Y., Ma, H. L., & Wang, Z. B. (2016). Ultrasonic effects on the degradation kinetics, preliminary characterization and antioxidant activities of polysaccharides from Phellinus linteus mycelia. ULtrasonics Sonochemistry, 29, 251–257.
- Zheng, L., & Sun, D. W. (2006). Innovative applications of power ultrasound during food freezing processes - a review. Trends in Food Science & Technology, 17, 16–23.