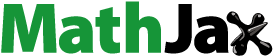
ABSTRACT
In this study, second-derivative laser-induced fluorescence spectroscopy (SD-LIF) and its derivative intrinsic-ratiometric laser-induced fluorescence spectroscopy (IR-LIF) were developed for the qualitative and quantitative analysis of camellia oil adulterated with sunflower oil or rapeseed oil, respectively. In the qualitative analysis, three pure oils and two blended oils were successfully discriminated based on SD-LIF and linear discriminant analysis. The influence of fluorescence concentration quenching on the adulterant concentration analysis was discussed. IR-LIF was established by using 646 nm chlorophyll b fluorescence as the insensitive reference and proved to be an effective approach to reduce the possible quenching influence on the full spectrum. Then, the combination of IR-LIF and partial least squares regression was employed to determine the adulterant concentration, and the results were satisfactory. The plots of the observed versus predicted adulteration concentration values showed high linearity. Root-mean-square errors of prediction lower than 3% were achieved.
RESUMEN
En el presente estudio se desarrollaron la espectroscopia de fluorescencia de segunda derivada inducida por láser (SD-LIF) y su derivada, la espectroscopia de fluorescencia intrínseca-ratiométrica inducida por láser (IR-LIF), para el análisis cualitativo y cuantitativo de aceite de camelia (CO) adulterado con aceite de girasol (SO) y con aceite de canola (RO), respectivamente. En el análisis cualitativo, se discriminaron con éxito tres aceites puros y dos aceites mezclados a partir de la SD-LIF y el análisis discriminante lineal (LDA). En este sentido, se analizó la influencia que tiene la desactivación de concentración de fluorescencia en el análisis de concentración del adulterante. Mediante el uso de 646 nm de fluorescencia de clorofila b como referencia insensible se estableció la IR-LIF, comprobándose que representa una opción efectiva para reducir la posible influencia de la desactivación en todo el espectro. Luego se utilizó la combinación de la IR-LIF y la regresión de mínimos cuadrados parciales (PLSR) para determinar la concentración del adulterante, obteniéndose resultados satisfactorios. Los diagramas de los valores de la concentración de adulteración observados versus los valores pronosticados muestran una alta linealidad. Se logró obtener raíces cuadradas de errores cuadráticos medios de predicción (RMSEP) inferiores a 3%.
Introduction
Camellia oil (CO) is one of the most widely consumed oils in Asia and is well known as ‘Eastern olive oil’ because its major fatty acids resemble those of olive oil (Bumrungpert, Pavadhgul, & Kalpravidh, Citation2016). CO is also rich in antioxidants such as vitamins and polyphenols, which makes it a highly nutritious oil (Su, Shih, & Lin, Citation2014). All these factors cause CO to be popular and seduce sellers to adulterate CO with other low-grade vegetable oils for profit. The common adulterants in CO are sunflower oil (SO) and rapeseed oil (RO) owing to their low cost. The qualitative and quantitative analyses of adulterants in CO deserve increasing attention not only because of the general interest of consumers in detecting adulterants but also because of health problems associated with these adulterants.
Many advanced methods have been developed and employed for the analysis of adulterants in vegetable oil. Sun et al. employed a gas chromatography and mass spectrometry method based on fatty acid profiles in the discrimination of flaxseed oil adulterated with five types of vegetable oils (Sun et al., Citation2015). Liquid chromatography with tandem mass spectrometry has also been used for the adulteration detection of CO, and the monitored indicators were isoflavone, trans-resveratrol, and sinapic acid (Dou et al., Citation2018). High-performance liquid chromatography and nuclear magnetic resonance have also proved to be successful methods for identifying adulterants in vegetable oil based on their corresponding indicators (Garcia-Gonzalez, Mannina, D’Imperio, Segre, & Aparicio, Citation2004; Salghi, Armbruster, & Schwack, Citation2014). However, these methods either need sample pretreatment or consume substantial reagent, which limits their application in rapid detection techniques.
Fluorescence spectroscopy combined with chemometrics analysis is a sensitive, rapid and non-intrusive method for the analysis of adulterants in vegetable oil (Dankowska & Malecka, Citation2009). Generally, fluorescence signals in vegetable oil range from 300 to 800 nm and mainly originate from polyphenols, vitamins, and chlorophylls (Christodouleas, Fotakis, Papadopoulos, Dimotikali, & Calokerinos, Citation2012). These fluorophores often have high concentration levels and usually dominate the signal profile. However, signals from other low-concentration fluorophores exhibit a relatively lower fluorescence contribution and influence the signal profile to a lesser degree. Moreover, the fluorescent signals from different fluorophores in vegetable oil usually overlap with each other. These two factors will weaken the signal characteristic and therefore lead to a reduction in the performance of the calibration model. To enhance the signal characteristic, a second-derivative (SD) process may be a wise choice. The major benefit of the SD process is to increase the resolution of overlapping spectral bands, and then more characteristic information can be supplied to the calibration model (O’Haver et al., Citation1982).
In general, fluorescence measurements are performed by using an intensity-based approach (Bradley B. Collier & McShane, Citation2013). However, these intensity-based measurements are questionable due to fluorophore inhomogeneity, optoelectronic drift, photobleaching, or fluorescence quenching, all of which can result in concentration measurement inaccuracies (Bradley B. Collier & McShane, Citation2013; M. I. Stich, Fischer, & Wolfbeis, Citation2010). Obviously, inaccuracies in the concentration measurement will lead to a decrease in the accuracy of the adulterant concentration analysis, which is based on Beer’s law and a linear mixture model. One way to reduce the influence of these problems is to use an intensity-based ratiometric approach (B. B. Collier, Singh, & Mcshane, Citation2011). The measurement indicator of this approach is a unitless ratio value, which is obtained by dividing the intensity of one wavelength band by the intensity of a different wavelength band (Bradley B. Collier & McShane, Citation2013). Generally, the ratiometric approach only focuses on one analyte of interest and uses only one ratiometric value. In addition, the ratiometric approach is usually accomplished by adding two exogenous fluorescence dyes into the matrix, one of which is sensitive to the concentration of the analyte and the other of which serves as an insensitive reference (Kostov, Harms, & Rao, Citation2001). However, the adulterant concentration analysis of vegetable oil based on chemometrics is usually a pattern recognition process that is based on the full spectrum. The intensity value of each wavelength is measured and used in the calibration process. Thus, only one ratiometric value is insufficient to reduce the influence of intensity-based problems on the full spectrum. Moreover, the utilization of an exogenous fluorescence dye is not exactly an appropriate choice for non-intrusive monitoring. Hence, a ratiometric approach based on a full spectrum should be established by using the fluorescence of an endogenous fluorophore as the insensitive reference.
Recently, laser-induced fluorescence (LIF) has come into service for food safety and quality analysis (Noh & Lu, Citation2007). High sensitivity, fast operation, and portable size make LIF a technique that is suitable for on-site detection. However, to the best of our knowledge, reports regarding the qualitative and quantitative analyses of CO adulteration based on the combination of SD processing and LIF have not yet been published. In this work, second-derivative LIF spectroscopy (SD-LIF) was employed for the identification of three pure oils and two blended oils. Additionally, intrinsic-ratiometric laser-induced fluorescence spectroscopy (IR-LIF) was established based on SD-LIF and effectively validated to reduce the influence of fluorescence concentration quenching on the full spectrum. Then, IR-LIF was successfully employed for the quantitative analysis of adulterants in CO. Due to their high sensitivity and fast operation, SD-LIF and IR-LIF can be applied to other food safety detection methods as well.
Materials and methods
Samples
CO, SO, and RO were purchased from local markets or online (). To guarantee the quality and botanical origin, only brands with a good reputation were considered. It is necessary to point out that the refining process may result in several unpredictable effects; therefore, only extra virgin grade oils were selected. The adulterated samples were prepared by adding SO or RO to the CO at an amount ranging from 1% to 50% (v/v) with a gradient of 1%. Then, 50 adulterated samples were generated for each adulteration group. These two adulterated groups are referred to as SCO and RCO in this article. Additionally, 50 samples of CO, SO, and RO were also prepared. To avoid inner filter effects, the samples were dissolved by mixing 50 mg of the oil into 9.95 mL of 95% (v/v) n-hexane. Thus, the optical density of the samples at the excitation wavelength did not exceed 0.1 (Smyk, Amarowicz, Szabelski, Gryczynski, & Gryczynski, Citation2009). All samples were prepared in triplicate and were stored at −4°C in a refrigerator prior to the experiments and analysis.
Table 1. Edible vegetable oils investigated in this study.
Tabla 1. Aceites vegetales comestibles investigados en este estudio.
Instrumentation
A typical LIF system is shown in . Briefly, a 485 nm laser module was used as the excitation light source. The test sample was contained in a cuvette. The front face illumination and cuvette underwent 45° rotation to decrease the inner filter effect. An optical fibre linked to a spectrograph was used to transfer the fluorescence signals. A 485 nm long-pass edge filter was used to avoid the elastic light. The spectra ranged from 515 to 800 nm at 1 nm intervals, and the integration time was 1 s. Finally, the fluorescence data of different samples were analysed by a computer. All the modules were assembled into one for portability.
Statistical analysis
Generation of SD-LIF spectra
The spectral data were processed by Matlab 2011a (MathWorks, Inc., USA). Samples were measured in triplicate, and averaged spectra were used as the original spectra. Then, the standard normal variate algorithm was adopted to rule out the possibility of a baseline shift and global intensity changes in all the fluorescence data. Furthermore, all original spectra were normalized to the maximum fluorescence intensity. Notably, the SD process makes the spectra highly sensitive to noise. Thus, the Savitzky–Golay algorithm with a window of five points was employed to decrease the noise as much as possible. Then, the SD process was performed on the original spectra, and the SD-LIF spectra were obtained.
Establishment and validation of IR-LIF
To find an appropriate reference to establish IR-LIF, two conditions should be satisfied: the fluorescence of the reference should be separated from the other fluorophores, and the reference should be insensitive to the concentration of the adulterant (Kostov et al., Citation2001). In SD-LIF, the fluorescence intensities of chlorophyll b in CO, SO, and RO were successfully separated from the total chlorophyll fluorescence. The peak fluorescence intensity of chlorophyll b at 646 nm was found to be almost the same in the three pure oils, indicating that the intensity was substantially insensitive to the concentration of the adulterant at this wavelength. Thus, the chlorophyll b fluorescence at 646 nm was considered appropriate to serve as the insensitive reference in the full spectrum. Then, the IR-LIF curve was established by dividing the SD-LIF curve by the fluorescence intensity of chlorophyll b at 646 nm.
To validate the effectiveness of IR-LIF in adulterant concentration analysis, each ratiometric value in the IR-LIF curves was fitted against that in the adulteration concentration curves. Then, the linearity and sensitivity of these fitted curves were evaluated. For simplicity, one ratiometric value, the fluorescence of chlorophyll a at 672 nm to that of chlorophyll b at 646 nm (Ra/b), was validated and displayed. Other ratiometric values in the IR-LIF curve were validated in the same way. Thus, the feasibility and effectiveness of IR-LIF can be validated.
Model calibration
Before the establishment of the calibration model, principal component analysis (PCA) was first applied to compress the data scale and check the intrinsic variables of the spectral data. For identification, a linear discriminant analysis (LDA) method was employed to discriminate samples by using the SD-LIF values as inputs. Partial least squares regression (PLSR) was selected as the method for adulteration concentration analysis based on the IR-LIF curves. Cross-validation and external validation were applied to evaluate the predictive ability of the model. For each kind of adulterant, 50 adulterated samples, which comprised the training set (n = 50), were arranged by the adulterant concentration. Another 10 adulterated samples with contents ranging from 5–50% (v/v) with a gradient of 5% were prepared and put into the test set (n = 10). The root-mean square-error of calibration (RMSEC), the root-mean-square error of cross-validation (RMSECV), the root-mean-square error of prediction (RMSEP) and the corresponding coefficients of determination for the calibration (), cross-validation (
) and prediction (
) were calculated. For comparison, LDA and PLSR were also built based on the LIF data.
Results and discussion
Identification of pure and blends oils
LIF spectra of CO, SO, and RO are shown in . In the range from 515 to 600 nm, the fluorescence profiles of the three pure oils were different from each other. However, the fluorescence in this range was at a relatively low intensity level, and no characteristic peaks appeared. Fluorescence at such a low intensity level might result from the destruction of some fluorophores, although the oil manufacturers all claimed that the products were of extra virgin grade. In addition, the fluorescence of all the oils in this range slightly changed. All these data indicated the absence of characteristic information. Hence, the fluorescence in this range was considered inadequate to illuminate the differences between the three pure oils. In the range from 600 to 800 nm, the total chlorophyll fluorescence at 659 nm of the three pure oils increased in the order from SO to CO to RO. However, the spectra of the pure oils still overlapped with each other in part of the range and on the whole resembled each other to some degree, although they were not supposed to in terms of botanical origin. The similarity was in the origin, which led to a decrease in the performance of the LDA model, and there was an increasing possibility for the two kinds of oils to be assigned to one group. Hence, the SD process, which focuses on the resolution increase in the overlapping spectral bands, was expected to explore more characteristic information in these spectra with the hope of enhancing the identification ability of the LDA model.
Figure 2. Laser-induced fluorescence spectra of oils, including camellia, sunflower and rapeseed oil. The sample amount of each oil is 50.
Figura 2. Espectros fluorescentes inducidos por láser de aceites, incluyendo aceites de camelia, girasol y canola. La cantidad de muestras de cada aceite es 50rapeseed oil. The sample amount of each oil is 50.
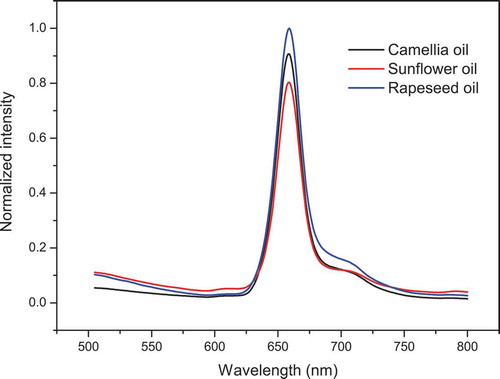
SD-LIF spectra of the three pure oils are shown in . In the range from 600 to 800 nm, the chlorophyll fluorescence was split into three characteristic parts. The maximal fluorescence of chlorophyll b and chlorophyll a appeared at 646 nm and 672 nm, respectively (Trytek et al., Citation2011). The chlorophyll b fluorescence at 646 nm of the three pure oils was almost the same. The chlorophyll a fluorescence at 672 nm ranged in a sequence from SO to CO to RO, and the total chlorophyll fluorescence at 659 nm also obeyed this order, indicating that the total chlorophyll fluorescence might be dominated by chlorophyll a. Notably, the Ra/b values were different among the three pure oils (CO: 1.25, SO: 1.13 and RO: 1.52), which might be an explanation for the botanical origin differences. With the SD process, more characteristic information appeared, which will increase the identification ability of the LDA model.
Figure 3. Second derivative laser-induced fluorescence spectra of oils, including camellia, sunflower and rapeseed oils. Inset graph is partial enlargement of second derivative laser-induced fluorescence spectra of the three pure oils ranging from 641 to 650 nm.
Figura 3. Espectros fluorescentes de segunda derivada inducidos por láser de aceites, incluyendo aceites de camelia, girasol y canola. La gráfica del recuadro es una ampliación parcial de los espectros fluorescentes de segunda derivada inducidos por láser de los tres aceites puros, oscilando entre 641 y 650 nm.
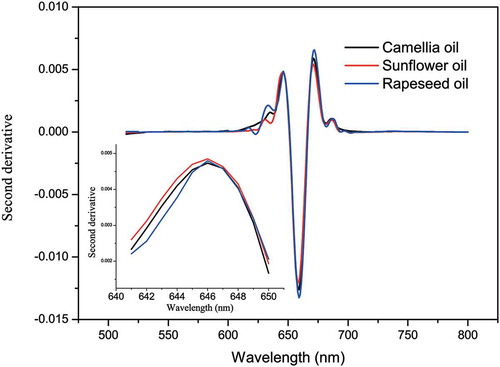
PCA was used to compress the data scale and find the intrinsic variables before identification. Then, the LDA method was used to identify the pure and blended oils. The samples were successfully assigned into the five groups shown in . The first two principal components, which explained 94.9% of the total variance (71.2% and 23.7%), covered sufficient information for identification. The intervals between SO and CO and CO and RO were occupied by SCO and RCO, respectively. This finding was an indication that the blended oils, which were mixed by pure oils, displayed the mutual properties of the two pure oils. Finally, an identification accuracy of 100% was obtained. However, the identification accuracy of LDA was only 94.7% based on the LIF data (). More characteristic information from SD-LIF might account for the enhancement in the effectiveness and sensitivity of the LDA model during identification.
Table 2. Identification accuracy of linear discriminant analysis (LDA) based on second derivative laser-induced fluorescence (SD-LIF) and laser-induced fluorescence (LIF), respectively.
Tabla 2. Precisión de identificación del análisis discriminante lineal (LDA), basado en la fluorescencia de segunda derivada inducida por láser (SD-LIF) y la fluorescencia inducida por láser (LIF), respectivamente.
Figure 4. PCA score plot from SD-LIF spectra of oils, including camellia oil (CO), sunflower oil (SO), rapeseed oil (RO), camellia oil adulterated with sunflower oil (SCO), and camellia oil adulterated with rapeseed oil (RCO), for the purpose of identification.
Figura 4. Diagrama de puntos del análisis de componentes principales (PCA) de un espectro SD-LIF de aceites, incluyendo aceites de camelia (CO), girasol (SO), canola (RO), aceite de camelia adulterado con aceite de girasol (SCO) y aceite de camelia adulterado con aceite de canola (RCO), para fines de identificación.
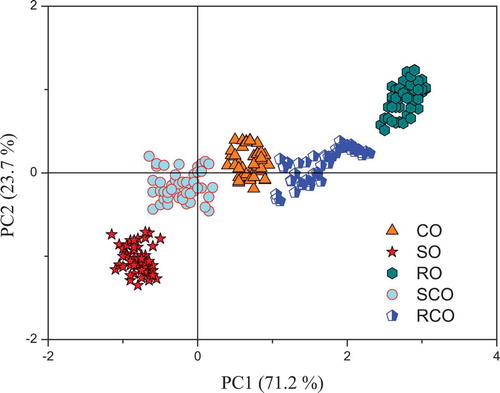
Concentration of adulterant analysis
The adulterant concentration analysis is another important task in this study. According to Beer’s law, the fluorescence intensity is proportional to the fluorophore concentration when it is analysed at a relatively low concentration level. In addition, this law is established for experiments under relatively mild conditions (e.g., pH, temperature, pressure), and matrix interference can also be ignored. Chlorophyll played an important role in the estimation of the adulterant concentration. In SCO, the chlorophyll fluorescence at 659 nm decreased with increasing adulterant concentration of SO (). In RCO, the chlorophyll fluorescence at 659 nm increased with increasing adulteration concentration of RO in a relatively linear way when the adulteration concentration was lower than 20% (). However, the fluorescence at 659 nm of RCO was observed to progress in a nearly horizontal manner when the adulteration concentration was higher than 40%. One possible explanation for this phenomenon is that fluorescence concentration quenching occurred. Fluorescence concentration quenching means that the fluorescence is quenched as a result of the formation of a ground-state complex, which is a combination of a fluorophore and a quencher (Lakowicz & Masters, Citation2006). When the complex absorbs light, it immediately goes back to the ground state as a result of mechanisms other than fluorescence. Hence, non-fluorescence emission will be observed from the ground-state complex, and the total fluorescence intensity partially decreases. Notably, fluorescence concentration quenching is a probability event that depends on the concentration, which means that quenchers at a high concentration level have more opportunities to combine with fluorophores, which leads to a greater decrease in the fluorescence intensity. The quenching complexes are varied, and the same kind of fluorophore can also form a ground-state complex such as a dimer or a polymer. In our study, chlorophyll itself might have served as the quencher, and the chlorophyll fluorescence was quenched because of the formation of a chlorophyll complex (Duffy, Ruban, & Barford, Citation2008; Shi, Barber, & Zhao, Citation2013). Specifically, no fluorescence concentration quenching occurred when CO was adulterated with SO. This supposition was easily derived from the fact that the chlorophyll concentration of SO was lower than that of CO. Hence, the chlorophyll in CO was diluted by SO, and the possibility of the formation of a ground-state complex decreased. However, the opposite case occurred when CO was adulterated with RO. The chlorophyll concentration of RO was higher than that of CO, and therefore, the chlorophyll concentration of CO was increased by the addition of RO. Hence, the possibility of the formation of a ground-state complex increased, which led to an inaccurate measurement.
Figure 5. Fitted curves of Ra/b and 659 nm fluorescence intensity versus adulteration concentration, respectively. SCO refers to samples of camellia oil adulterated with sunflower oil; Ra/b refers to the ratiometric value: 672 nm chlorophyll a fluorescence versus 646 nm chlorophyll b fluorescence; FL refers to fluorescence intensity at 659 nm. In order to display clearly, only six fitted points are shown in each fitted curve with the form of mean ± SD of three replicates (n = 3).
Figura 5. Curvas ajustadas de Ra/b y 659 nm intensidad de fluorescencia versus la concentración de adulteración, respectivamente. SCO significa muestras de aceite de camelia adulterado con aceite de girasol; Ra/b es el valor ratiométrico: 672 nm fluorescencia de clorofila a versus 646 nm de fluorescencia de clorofila b; FL indica la intensidad de fluorescencia a 659 nm. Para una exposición clara, se muestran solo seis puntos ajustados en cada una de las curvas ajustadas, con la forma de medias ± DE de tres repeticiones (n = 3).
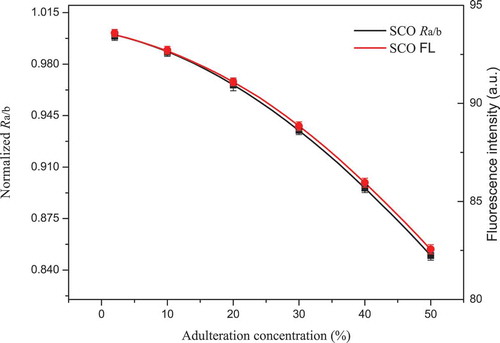
Figure 6. Fitted curves of Ra/b and 659 nm fluorescence intensity versus adulteration concentration, respectively. RCO refers to samples of camellia oil adulterated with rapeseed oil; Ra/b refers to the ratiometric value: 672 nm chlorophyll a fluorescence versus 646 nm chlorophyll b fluorescence; FL refers to fluorescence intensity at 659 nm. For the purpose of display clearly, only six fitted points are shown in each fitted curve with the form of mean ± SD of three replicates (n = 3).
Figura 6. Curvas ajustadas de Ra/b y 659 nm de intensidad de fluorescencia versus la concentración de adulteración, respectivamente. RCO significa muestras de aceite de camelia adulterado con aceite de canola; Ra/b es el valor ratiométrico: 672 nm fluorescencia de clorofila a versus 646 nm fluorescencia de clorofila b; FL indica la intensidad de fluorescencia a 659 nm. Para una exposición clara, se muestran solo seis puntos ajustados en cada una de las curvas ajustadas con la forma de medias ± DE de tres repeticiones (n = 3).
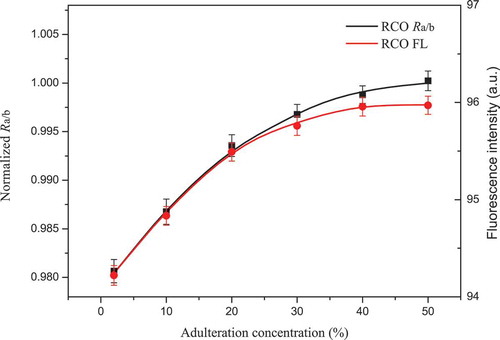
Compared to the intensity-based measurement, the ratiometric approach was considered the better alternative for adulteration concentration analysis, as mentioned earlier. Notably, the linearity and sensitivity in the fitted curves of Ra/b versus the adulteration concentration were higher than those at a fluorescence intensity of 659 nm ( and ). In SCO, a slight improvement in linearity and sensitivity appeared. No static quenching may have occurred, and therefore, the ratiometric value Ra/b performed slightly better than that of the intensity-based measurement at 659 nm. In RCO, a relatively significant improvement in the linearity and sensitivity appeared when the adulteration concentration of RO exceeded 40%. The ratiometric value Ra/b performed much better than that of the intensity-based measurement at 659 nm. However, it is worth noting that the influence of fluorescence concentration quenching was only diminished but not completely eliminated and that the ratiometric value Ra/b improved compared to that of the intensity-based measurement (Bradley B. Collier & McShane, Citation2013). According to Beer’s law, the high linearity demonstrated the feasibility of the ratiometric value Ra/b in the adulteration concentration analysis. Moreover, the high sensitivity to the change in the adulteration concentration demonstrated the superiority of the ratiometric method compared with the intensity-based measurement when fluorescence concentration quenching occurred. Other ratiometric values in IR-LIF were validated in the same way, and the results were satisfactory. Consequently, the feasibility and effectiveness of IR-LIF in adulterant concentration analysis were validated, and then the IR-LIF values were set as the inputs for the PLSR model.
Specifically, 50 samples were selected in the training set (n = 50), and 10 samples (n = 10) were selected in the test set. Then, PLSR was performed for a quantitative analysis of SCO and RCO. The predicted and observed values display a high linearity, as shown in and . The values of SCO and RCO were 0.98 and 0.97, respectively. The RMSEP values were lower than 2% and 3% in SCO and RCO, respectively. However, the
and RMSEP values of PLSR based on the LIF values of SCO and RCO performed relatively poorly (). These results demonstrated that the PLSR model was more effective for adulterant analysis based on IR-LIF.
Table 3. PLSR statistics for cross and external validation of concentration of adulterant analysis based on intrinsic-ratiometric laser-induced fluorescence (IR-LIF) and laser-induced fluorescence (LIF), respectively.
Tabla 3. Estadísticas PLSR de la validación cruzada y externa del análisis de concentración del adulterante, basado en la fluorescencia intrínseca-ratiométrica inducida por láser (IR-LIF) y la fluorescencia inducida por láser (LIF), respectivamente.
Conclusions
In conclusion, this study successfully discriminated three pure vegetable oils and two blended vegetable oils and determined the adulteration of CO with SO or RO. By the SD process, the chlorophyll b fluorescence was separated from the total chlorophyll fluorescence and selected as the insensitive reference for the IR-LIF measurements. Then, IR-LIF was proven to be an effective approach to reduce the influence of fluorescence concentration quenching. Regarding identification, an accuracy of 100% was achieved by performing LDA based on SD-LIF. In the adulterant concentration analysis, PLSR was successfully performed based on IR-LIF, and prediction errors of the adulteration concentration lower than 3% were achieved. It was demonstrated that SD-LIF and IR-LIF coupled with multivariate analysis are simple, fast and non-intrusive methods for qualitative and quantitative purposes, respectively. Due to the high sensitivity and no requirement for sample pretreatment, SD-LIF and IR-LIF are promising methods for identifying adulterants in vegetable oil and can be used in other fields.
Disclosure statement
No potential conflict of interest was reported by the authors.
Additional information
Funding
References
- Bumrungpert, A., Pavadhgul, P., & Kalpravidh, R. W. (2016). Camellia oil-enriched diet attenuates oxidative stress and inflammatory markers in hypercholesterolemic subjects. Journal of Medicinal Food, 19(9), 895–898.
- Christodouleas, D., Fotakis, C., Papadopoulos, K., Dimotikali, D., & Calokerinos, A. C. (2012). Luminescent methods in the analysis of untreated edible oils: A review. Analytical Letters, 45(5–6), 625–641.
- Collier, B. B., & McShane, M. J. (2013). Time-resolved measurements of luminescence. Journal of Luminescence, 144, 180–190.
- Collier, B. B., Singh, S., & Mcshane, M. (2011). Microparticle ratiometric oxygen sensors utilizing near-infrared emitting quantum dots. Analyst, 136(5), 962–967.
- Dankowska, A., & Malecka, M. (2009). Application of synchronous fluorescence spectroscopy for determination of extra virgin olive oil adulteration. European Journal of Lipid Science and Technology, 111(12), 1233–1239.
- Dou, X. J., Mao, J., Zhang, L. X., Xie, H. L., Chen, L., Yu, L., … Li, P. W. (2018). Multispecies adulteration detection of camellia oil by chemical markers. Molecules, 23(2), 241–251.
- Duffy, C. D. P., Ruban, A. V., & Barford, W. (2008). Theoretical investigation of the role of strongly coupled chlorophyll dimers in photoprotection of LHCII. Journal of Physical Chemistry B, 112(39), 12508–12515.
- Garcia-Gonzalez, D. L., Mannina, L., D’Imperio, M., Segre, A. L., & Aparicio, R. (2004). Using H-1 and C-13 NMR techniques and artificial neural networks to detect the adulteration of olive oil with hazelnut oil. European Food Research and Technology, 219(5), 545–548.
- Kostov, Y., Harms, P., & Rao, G. (2001). Ratiometric sensing using dual-frequency lifetime discrimination. Analytical Biochemistry, 297(1), 105–108.
- Lakowicz, J. R., & Masters, B. R. (2006). Principles of fluorescence spectroscopy (3rd ed.). New York, NY: Springer.
- Noh, H. K., & Lu, R. (2007). Hyperspectral laser-induced fluorescence imaging for assessing apple fruit quality. Postharvest Biology and Technology, 43(2), 193–201.
- O’Haver, T. C., Fell, A. F., Smith, G., Gans, P., Sneddon, J., Bezur, L., … Ahmad, T. A. (1982). Derivative spectroscopy and its applications in analysis. Analytical Proceedings, 19(1), 192–194.
- Salghi, R., Armbruster, W., & Schwack, W. (2014). Detection of argan oil adulteration with vegetable oils by high-performance liquid chromatography-evaporative light scattering detection. Food Chemistry, 153(24), 387–392.
- Shi, W. J., Barber, J., & Zhao, Y. (2013). Role of formation of statistical aggregates in chlorophyll fluorescence concentration quenching. Journal of Physical Chemistry B, 117(15), 3976–3982.
- Smyk, B., Amarowicz, R., Szabelski, M., Gryczynski, I., & Gryczynski, Z. (2009). Steady-state and time-resolved fluorescence studies of stripped Borage oil. Anal Chim Acta, 646(1–2), 85–89.
- Stich, M. I., Fischer, L. H., & Wolfbeis, O. S. (2010). Multiple fluorescent chemical sensing and imaging. Chemical Society Reviews, 39(8), 3102–3104.
- Su, M. H., Shih, M. C., & Lin, K. H. (2014). Chemical composition of seed oils in native Taiwanese Camellia species. Food Chemistry, 156(3), 369–373.
- Sun, X., Zhang, L., Li, P., Xu, B., Ma, F., Zhang, Q., & Zhang, W. (2015). Fatty acid profiles based adulteration detection for flaxseed oil by gas chromatography mass spectrometry. Lebensmittel-Wissenschaft und-Technologie, 63(1), 430–436.
- Trytek, M., Janik, E., Maksymiec, W., Fiedurek, J., Lipke, A., & Majdan, M. (2011). The spectral and catalytic studies of chlorophylls and pheophytins in mimetic biotransformation of α-pinene. Journal of Photochemistry and Photobiology A: Chemistry, 223(1), 14–24.