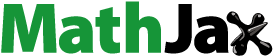
ABSTRACT
The presentation of high pressure processing (HPP) on corn starch has been reported, but the effect on protein in corn gluten meal (CGM) is rarely explored. The purposes of this study were to investigate the effect of HPP treatment on structural and functional properties of the protein in CGM. Protein was treated at 200–600 MPa for 5–15 min. Results showed that HPP treatment increased protein solubility, water and oil binding capacities, emulsion stability, foaming and foam stability. Protein which treated at 400 MPa for 15 min had the more enhanced functionality. Moreover, under this condition, the protein aggregates gradually dissociated, and the particle size decreased. These results indicated that the functional groups and structure of CGM protein were affected by the HPP treatment. We hope to convert an underutilized material into a valuable one through HPP in food industry.
1. Introduction
Corn gluten meal (CGM) is a by-product of the wet milling process used for corn starch production, the major protein components include zein, glutelin and globulin, which are rich in hydrophobic amino acids such as isoleucine, leucine, proline, alanine and glutamine (X. Zheng et al., Citation2014). However, it is highly hydrophobic which limits its application in food Kopparapu et al. (Citation2022). The annual production of CGM in China exceeds 200,000 tons, which contains about 60% protein and 20% starch. CGM is often used as animal feed and is underutilized in the food industry, due to the poor functionality and low digestibility of its natural protein, which causes the wastage of resources. Therefore, overcoming its limitations and improving the solubility in the aqueous systems is necessary (Jin, et al., Citation2015a, Citation2015b; Zheng et al., Citation2015). Zhou et al. (Citation2022) reported that UHP treatment can increase solubility, emulsion activity and stability, foaming capacity and stability of buckwheat 13S globulin. J. Zheng et al. (Citation2020) found the UHP treatment obviously improved the speed and degree of glycation and the composite-modified phycocyanin showed high solubility and good emulsifying and foaming performance. CGM protein hydrolysates could significantly improve oxidative stability, and could be a potential functional antioxidant for food emulsion applications (Shen et al., Citation2019). Ultra-high pressure (UHP) can affect corn starch architecture and induce starch gelatinization at the room temperature (Pu et al., Citation2021).
Razi et al. (Citation2024) studied the effect of high hydrostatic pressure (HHP) on the structural properties of whey protein, and found it can affect solubility and particle size of protein increased; a more crystalline structure was observed with 600MPa. Structural and functional properties of soy protein, kabuli chickpea protein can also be modified by HHP (Y. Xu et al., Citation2024; Zengin et al., Citation2024). HHP might improve the soy protein unfolding and the exposure of its hydrophobic groups, and it may principally decrease the emulsion stability index (Dehnad et al., Citation2024).
In recent years, the application of HPP in food processing has been increasing. HPP rarely changes the nutritional and sensory quality of food and is a promising technology (Zou et al., Citation2019), which has been proved to be an effective method to modify protein (Tang & Ma, Citation2009). Protein structure can be changed by HPP, and the interaction between proteins and small molecules can also be affected (Puppo et al., Citation2004). Stress tolerance varies with the protein size and structure, with a change in the protein structure, some of its functional properties, such as solubility, water and oil binding capacities, emulsion stability, and foam capacity and foam stability, also change (Dehnad et al., Citation2024).
CGM is a rich source of protein that is insoluble in water and has limited applications in the food industry (Mirzaee et al., Citation2022; Shen et al., Citation2019). In this study, we aimed to investigate the effect of HPP on the structural and functional properties, including solubility of CGM protein, to increase its application in the food and feed industry.
2. Materials and method
2.1. Materials and reagents
CGM was provided by the Rongcheng Corn Development Co. Ltd. (Inner Mongolia, China). Spectrum-pure potassium bromide (KBr) was purchased from Tianjin Hengchuang Lida Technology Development Co., Ltd. All other analytical-grade chemicals were obtained from Tianjin Yongda Chemical Regent Co., Ltd.
2.2. Preparation of protein dispersions
CGM (500 g) was suspended in 5000 mL of distilled water, and the pH was adjusted to 12 using 0.5 M sodium hydroxide. The mixture was stirred and incubated for 1.5 h at 37°C after resting for 10 min. The alkaline CGM solution was centrifuged at 4000 × g for 20 min, the supernatant was retained and its pH was adjusted to 5.5 using 1 M hydrochloric acid. After resting for 10 min, the solution centrifugated at 4000 × g for 20 min, and the precipitate was rinsed using deionized water. The protein concentrate was lyophilized and stored at −20°C; purity of lyophilized product was 85.87% (Ma et al., Citation2021).
2.3. HPP treatment
The HPP treatment was given in a laboratory-scale high pressure processing unit (UHP-600-5 L, Kefa High-Pressure Food Processing Inc., Baotou, China) with a 5 L cylindrical pressure chamber capable of operating up to the pressure-selected levels of 600 MPa. The method described by Ma et al. (Citation2021) was used with minor modifications. The freeze-dried protein (3 g), after mixing with distilled water (1.2 mL), was vacuum-packed in a food-grade polyethylene bag (7 cm × 10 cm, brand of Aiboshi, locally manufactured, Dongguan, China), and soaked for 4 h. The HPP treatment was given one at a time, respectively, at 200, 400, or 600 MPa for 5, 10 or 15 min (holding time) at 20°C. The unit’s pressurization rate was approximately 50 MPa/s, and the depressurization rate was 100 MPa/s. The protein extract was placed onto a plate and lyophilized after the HPP treatment.
2.4. Protein solubility
The solubility of CGM protein concentrate was determined according to the method described by Gao et al. (Citation2023) with some modifications. CGM protein (500 mg) was mixed in 50 mL of distilled water, oscillated for 30 min in a water bath, and centrifuged at 4000 × g for 20 min. The supernatant was retained, for determining the protein levels by the Biuret method, and the protein solubility was expressed as the percentage ratio of the supernatant protein content to the total protein content.
2.5. Water binding capacity
The water binding capacity was determined using the method described by Malomo et al. (Citation2014) with some modifications. The protein sample (500 mg) was mixed in 10 mL of 0.01 M phosphate buffer at pH7. The mixture was vortexed for 5 min, allowed to stand for 30 min and centrifuged at 4000 × g for 20 min. The pellets were recorded and weighed (Mpwet). The pellet was then dried in an oven (105°C for 24 h) and weighed again (Mpdry). Three replicates were analyzed per sample.
2.6. Oil binding capacity
2.5g of CGM protein (Misolate) was combined with 7.5 g of soybean oil in a falcon tube until a homogenous mixture was obtained. The mixture was vortexed for 5 min, allowed to stand for 30 min and centrifuged at 4000 × g for 20 min. The supernatants were decanted, and the remaining unbound oil removed by setting the tube upside down on filter paper for 10 min. Three replicates were analyzed per sample. The pellet containing the bound oil was weighed (Mpoil), and oil binding capacity was calculated using EquationEquation (2)(2)
(2) (Schwenke et al., Citation1981):
2.7. Determining emulsion stability
According to the method described by Zhou et al. (Citation2022) with slight modification. Dispersions containing 5 mL of 0.5 g/100 g protein were homogenized with 5 mL of soybean oil at 10,000 r/min for 2 min. The sample collected from the bottom of the emulsion was diluted in sodium phosphate buffer containing 0.1% sodium dodecyl sulfate (SDS) at pH 7. Emulsions were kept at room temperature for 30 min without agitation, and the oil droplet size distribution and mean particle diameter were measured again to assess emulsion stability.
Emulsion Stability (%) = Oil droplet size at 0 min/Oil droplet size after 30 min (3)
2.8. Determination of the foaming properties
According to the method described by Xiong et al. (Citation2018), with slight modification, the CGM protein concentrate solution (2%) was prepared, and the volume was recorded as V0. The solution was whipped for 2 min with a high-speed homogenizer at
10000 r/min, then transferred quickly to a 100 mL cylinder, and the foam volume V1 and V2 was recorded at 0 min and 30 min, respectively. The foaming capacity (%) and foam stability (%) were calculated using the following equations:
2.9. Microstructure by Scanning Electron Microscopy (SEM)
SEM (TM4000, Japan) was used to determine the morphological properties of CGM protein, according to the method described by Tian et al. (Citation2014) with minor modifications. The freeze-dried CGM protein sample, before and after the HPP treatment, was ground, mounted on a metallic stub, coated with a thin film of gold (10 nm) in a vacuum evaporator. Then, the produced powders were scanned by software (voltage of kV).
2.10. Fourier transform infrared spectroscopy
The KBr tablet method was used for the infrared spectroscopic analysis. The 150 mg of KBr powder (fixed weight) was mixed with 1.5 mg of protein sample, before and after HPP treatment was weighed and ground into a uniform powder with an agate mortar and pestle. A Fourier infrared spectrometer (Thermo Fisher Corporation, American) was used for scanning, with a wavelength of 4000–400 cm−1, and a resolution of 4 cm−1, and the sample was scanned 64 times.
2.11. Measurement of particle size distribution
Accurately weighed 1 g of protein was added to 100 mL of distilled water, and the laser particle size analyzer (BT-2002, Dandong Baxter Technology Co., Dandong, China) was used for the particle-size determination. The refractive index of the particles was 1.53, and the dispersant was 1.33 (Gravel et al. Citation2022).
2.12. Statistical analysis
All the tests were performed at least in duplicates. The statistical results are shown as mean ± standard deviation and were analyzed using Statistical Package for the Social Sciences 25.0 and Origin 2018 software.
3. Results and discussion
3.1. Protein composition and solubility
The protein content of CGM was 78.39% and mainly composed of zein (54.30%) and glutelin (44.68%). Protein solubility is directly proportional to its hydration capacity and is the basis of other properties of protein. Protein solubility can affect the emulsifying ability, foaming ability and other physical and chemical properties. The protein solubility of the ultra-high pressure treatment groups were higher than that of untreated sample (Dehnad et al., Citation2024) (). Protein solubility increased with the increase in pressure holding time at 200 MPa. The solubility of CGM protein increased from 55.60% to 64.58% at 200 MPa for 15 min. The solubility significantly increased to 68.88% (p < .05), at 400 MPa for 10 min and decreased to 63.49% when the pressure was maintained at 600 MPa for 15 min. High pressures could disrupt noncovalent bonds, including hydrophobic and hydrogen bonds and electrostatic interaction, which maintain protein three-dimensional conformation (Y. Xu et al., Citation2024).
Table 1. Effect of high pressure processing on solubility of corn gluten meal protein.
HPP treatment at pressures below 200 MPa causes reversible changes in the quaternary structure of some proteins to unfold, thereby enhancing solvent-protein interaction. It showed high solubility at 400 MPa for 10 min, probably related to protein unfolding and dissociation of preformed aggregates (Achouri & Boye, Citation2013; Yin et al., Citation2008). However, at 600 MPa for 15 min, solubility was decreased (), due to protein aggregation which occurs through hydrophobic interactions (Y. Xu et al., Citation2024).
3.2. Effect of HPP on the water binding capacity of CGM protein
The water binding capacity of the HPP groups was better than that of untreated sample (). The water binding capacity of protein was increased with the extension of pressure holding time at 200 MPa; however, it was decreased at 400 MPa and 600 MPa, but was still higher than that of the control group. When the pressure-holding time was 5 min, 10 min and 15 min, the water binding capacity of protein first increased and then decreased with increasing pressure. In general, the water binding capacity of protein was the highest at 4.50 mL/g and 200 MPa for 15 min. Water binding capacity is associated with the protein conformation and amino acid composition. The unfolding of proteins leads to stable uniformity and density of protein network structure, and this structure may bind to water in proteins, helping to enhance water binding capacity. However, the re-aggregation of loosened proteins with high pressure can decrease the water binding capacity (Nie et al., Citation2021).
3.3. Effect of HPP on the oil binding capacity of CGM protein
Oil binding capacity can affect the texture and taste of food. The oil binding capacity of protein which treated with HPP at 400MPa for 15 min is better than that of untreated sample (), which agrees with previously reported HPP-treated kabuli chickpea protein and peanut protein (He et al., Citation2014; Y. Xu et al., Citation2024). The oil binding capacity was at the minimum value of 3.95 mL/g at 200 MPa for 15 min. When the pressure was kept at 400 MPa for 15 min, the oil binding capacity increased to 4.70 mL/g. However, when the pressure was at 600 MPa for 15 min, it was decreased to 4.50 mL/g. This can be because the hydration ability of hydrophilic groups was increased at 200 MPa, and the lipophilicity of protein was weakened (Guan et al., Citation2020). At 400 MPa, exposure of hydrophobic groups and increased lipophilicity of protein made the absorption capacity of the protein to oil increase again. It is worth noting that oil binding capacity does not increase continuously with further increasing the pressure and holding time. The higher oil binding capacity of HPP-treated protein may be associated with higher surface hydrophobicity with more exposed hydrophobic amino acids to interact with the oil (Y. Xu et al., Citation2024).
Figure 1–2. Effect of high pressure processing on oil binding capacity of corn gluten meal protein.
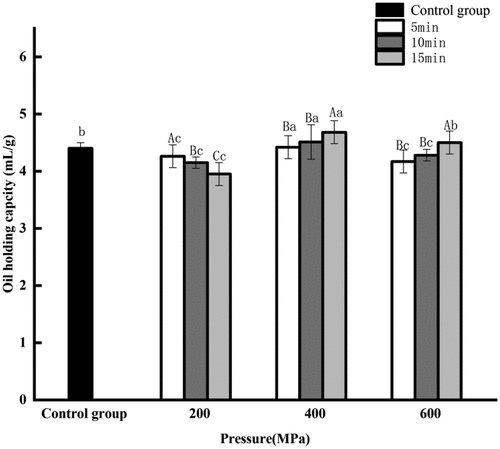
3.4. Effect of HPP on the emulsion stability of CGM protein
The emulsion stability of the HPP groups was better than that of untreated sample (). The emulsion stability of protein increased to 47.00% of the maximum value at 400 MPa for 5 min. It is thanks to the maximum unfolding of protein subunits at 300–400 MPa (Dehnad et al., Citation2024). The emulsion stability was the lowest when the pressure was kept constant at 600 MPa for 15 min.
The protein can attach to the surface of the oil droplet, forming a protective film to separate the water and oil, thereby improving its emulsion stability (He et al., Citation2014). A previous study showed that emulsion stability of buckwheat 13S globulin reached a maximum after 100 MPa and then slowly declined with the increase of pressure variation trend (Zhou et al., Citation2022).
3.5. Effect of HPP on the foaming capacity and foam stability of CGM protein
The foaming capacity and foaming stability of the HPP groups were better than that of untreated sample (). When the pressure holding time was extended from 5 min to 15 min, the foaming capacity and foam stability of protein first increased and then decreased with increasing pressure. At 400 MPa for 15 min, foaming capacity and foam stability reached the highest value, which were 45.20% and 54.50%, respectively. Because of UHP, the peptide chains unfolded, and the hydrophobic groups were exposed, and lots of hydroxyl groups were introduced into the protein at the same time. The interaction between hydrophobic and hydrophilic groups enhanced the foam stability of the protein (Medrano et al., Citation2009). As only the soluble part of protein is involved in the formation of bubbles, it resulted in the decline of foaming capacity at 600 MPa.
Figure 2–3. Effect of high pressure processing on foam stability of corn gluten meal protein.
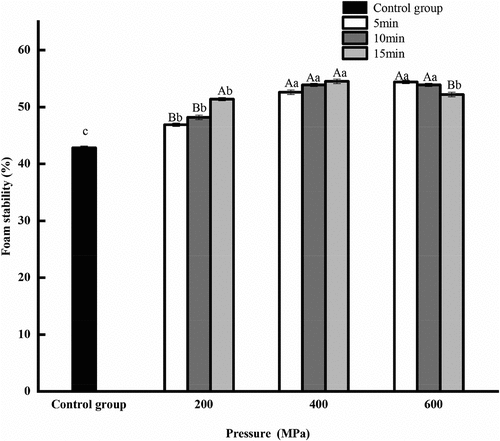
3.6. SEM results of CGM protein
The CGM protein structure of the control group was loose and unevenly distributed (). By applying pressure, the irregularity of shapes grew. The protein showed a converged network structure, and a few free protein particles still existed at 200 MPa and 400 MPa. At 600 MPa the microstructure was more homogeneous and compact (Peyrano et al., Citation2020). The extent of the pressure can change the compressibility of the protein. Excessive pressure also promoted the disintegration of aggregates, which is consistent with the results reported by (Liang et al., Citation2017).
3.7. Infrared spectrum results of CGM protein
A total of seven strong characteristic absorption peaks were found, namely 3736, 2934, 1665, 1530, 1240, 1153 and 1033 (). Of note, 3200–3600 cm−1 and 2800–3000 cm−1 represented the stretching vibration of -OH and -CH, respectively. Furthermore, 1600–1700 cm−1 (amide I band) represented the stretching vibration of C=O, and 1530–1550 cm−1 (amide II band) represented C-N and N-H bending vibration. The absorption peak at 2934 cm−1 represented the stretching vibration of -CH. Hypochromatic shifted to 2945 cm−1, the characteristic peak of C=O hypochromatic shifted from 1665 cm−1 to 1691 cm−1, the characteristic peak of C-N hypochromatic shifted from 1529 cm−1 to 1538 cm−1 and the other peaks did not shift with HPP treatment. After HPP treatment in all samples, especially treatment at 600 MPa, the band’s intensity increased which indicates that the secondary structure of CGM protein has changed (Zhang et al., Citation2020; J. Zheng et al., Citation2020).
3.8. Particle size of CGM protein
As shown in , with the extension of pressure (200–400 MPa) holding time, protein structure was disrupted and its particle size decreased (5 min). Then, with increasing time, CGM protein re-aggregated (10 min), and increased the CGM protein particle size. However, when pressure holding time extended to 15 min, the CGM protein was crushed into smaller aggregates. This is consistent with the results reported by Zhao (Citation2019). HPP causes different degrees of changes in the secondary and tertiary structures of proteins, and protein structure becomes loose, intermolecular hydrogen bonding and hydrophobic interactions change, thereby denaturing the protein and altering its functional characteristics (Li et al., Citation2011; Wang et al., Citation2008).
Table 2. Effect of high pressure processing on particle size of corn gluten meal protein.
4. Conclusions
In this study, we aimed to increase the functional and structural properties of CGM using HPP. We showed that the functional properties, including protein solubility, water and oil binding capacity, emulsion stability, foaming capacity and foam stability, increased with HPP treatment. The SEM and particle size analysis showed that HPP causes intermolecular hydrogen bonding and hydrophobic interactions in protein structure changed, thereby denaturing the protein and altering its functional characteristics. The infrared spectrum analysis showed that the functional groups and structure of protein were altered. To summarize, HPP, as a modification process for CGM protein, can be an efficient strategy, which can be used to convert an underutilized material into a valuable material in the food industry.
Disclosure statement
No potential conflict of interest was reported by the author(s).
Additional information
Funding
References
- Achouri, A., & Boye, J. (2013). Thermal processing, salt and high pressure treatment effects on molecular structure and antigenicity of sesame protein isolate. Food Research International, 53(1), 240–7. https://doi.org/10.1016/j.foodres.2013.04.016
- Dehnad, D., Emadzadeh, B., Ghorani, B., Assadpour, E., Yang, N., & Jafari, S. M. (2024). The influence of high hydrostatic pressure on different properties of legume proteins with an emphasis on soy proteins; a comprehensive review. Food Hydrocolloids, 146, 109188. https://doi.org/10.1016/j.foodhyd.2023.109188
- Gao, Y., Hao, X., Hu, Y., Zhou, N., Ma, Q., Zou, L., & Yao, Y. (2023). Modification of the functional properties of chickpea proteins by ultrasonication treatment and alleviation of malnutrition in rat. Food and Function, 14(3), 1773–1784. https://doi.org/10.1039/D2FO02492F
- Gravel, A., Dubois-Laurin, F., & Doyen, A. (2022). Effects of hexane on protein profile and techno-functional properties of pea protein isolates. Food Chemistry, 406, 135069. https://doi.org/10.1016/j.foodchem.2022.135069
- Guan, H., Diao, X., Liu, D., Han, J., Kong, B., Liu, D., Gao, C., & Zhang, L. (2020). Effect of high-pressure processing enzymatic hydrolysates of soy protein isolate on the emulsifying and oxidative stability of myofibrillar protein-prepared oil-in water emulsions. Journal of the Science of Food and Agriculture, 100(10), 3910–3919. https://doi.org/10.1002/jsfa.10433
- He, X., Liu, H., Liu, L., Zhao, G., Wang, Q., & Chen, Q. (2014). Effects of high pressure on the physicochemical and functional properties of peanut protein isolates. Food Hydrocolloids, 36, 123–129. https://doi.org/10.1016/j.foodhyd.2013.08.031
- Jin, J., Ma, H., Wang, K., Yagoub, A., Owusu, J., Qu, W., He, R., Zhou, C., & Ye, X. (2015). Effects of multi-frequency power ultrasound on the enzymolysis and structural characteristics of corn gluten meal. Ultrasonics Sonochemistry, 24, 55–64. https://doi.org/10.1016/j.ultsonch.2014.12.013
- Jin, J., Ma, H., Zhou, C., Luo, M., Liu, W., Qu, W., He, R., Luo, L., & Yagoub, A. E. G. A. (2015). Effect of degree of hydrolysis on the bioavailability of corn gluten meal hydrolysates. Journal of the Science of Food and Agriculture, 95(12), 2501–2509. https://doi.org/10.1002/jsfa.6982
- Kopparapu, N., Duan, Y., Huang, L., & Katrolia, P. (2022). Review on utilisation of corn gluten meal, a by-product from corn starch industry for production of value-added products. International Journal of Food Science and Technology, 57(9), 5592–5599. https://doi.org/10.1111/ijfs.15541
- Li, H., Zhu, K., Zhou, H., & Peng, W. (2011). Effects of high hydrostatic pressure on some functional and nutritional properties of soy protein isolate for infant formula. Journal of Agricultural and Food Chemistry, 59(22), 12028–12036. https://doi.org/10.1021/jf203390e
- Liang, Y., Guo, B., Zhou, A., Xiao, S., & Liu, X. (2017). Effect of high pressure treatment on gel characteristics and gel formation mechanism of bighead carp (Aristichthys nobilis) surimi gels. Journal of Food Processing and Preservation, 41(5), e13155. https://doi.org/10.1111/jfpp.13155
- Ma, S., Zhang, M., Shi, Y., Wang, H., & Chu, H. (2021). Effects of ultrahigh pressure treatment on eating quality of steamed oat and oat protein structure. CyTA-Journal of Food, 19(1), 56–62. https://doi.org/10.1080/19476337.2020.1857847
- Malomo, S., He, R., & Aluko, R. (2014). Structural and functional properties of hemp seed protein products. Journal of Food Science, 79(8), C1512–C1521. https://doi.org/10.1111/1750-3841.12537
- Medrano, A., Abirached, C., Panizzolo, L., Moyna, P., & Añón, M. C. (2009). The effect of glycation on foam and structural properties of β-lactoglobulin. Food Chemistry, 113(1), 127–133. https://doi.org/10.1016/j.foodchem.2008.07.036
- Mirzaee, H., Gavlighi, H., Mehdi, N., Udenigwe, C., & Khodaiyan, F. (2022). Relation of amino acid composition, hydrophobicity, and molecular weight with antidiabetic, antihypertensive, and antioxidant properties of mixtures of corn gluten and soy protein hydrolysates. Food Science and Nutrition, 11(3), 1257–1271. https://doi.org/10.1002/fsn3.3160
- Nie, Y., Zhang, W., Tian, G., Wang, Z., Gan, W., & Gao, H. (2021). Optimized the emulsifying properties of quinoa protein by ultra-high pressure treatment. Chinese Journal of High Pressure Physics, 35(3), 035901–1–10.
- Peyrano, F., Lamballerie, M., Avanza, M., & Speroni, F. (2020). Gelation of cowpea proteins induced by high hydrostatic pressure. Food Hydrocolloids, 111, 106191. https://doi.org/10.1016/j.foddhyd.2020.106191
- Pu, H., Liu, G., Huang, M., Zhang, C., Niu, W., Chen, X., & Huang, J. (2021). Effect of annealing on ultra-high pressure induced gelatinization of corn starch. Innovative Food Science and Emerging Technologies, 74, 102849. https://doi.org/10.1016/j.ifset.2021.102849
- Puppo, C., Chapleau, N., Speroni, F., de Lamballerie-Anton, M., Michel, F., Anon, C., & Anton, M. (2004). Physicochemical modifications of high-pressure-treated soybean protein isolates. Journal of Agricultural and Food Chemistry, 52(6), 1564–1571. https://doi.org/10.1021/jf034813t
- Razi, S., Mohebbi, M., Mirzababaee, S., Hesarineja, M., & Movahed, M. (2024). The effect of high hydrostatic pressure on the structure of whey proteins-guar gum mixture. Heliyon, 10(1), e24140. https://doi.org/10.1016/j.heliyon.2024.e24140
- Schwenke, K. D., Prahl, L., Rauschal, E., Gwiazda, S., Dabrowski, K., & Rutkowski, A. (1981). Functional properties of plant proteins. Part 2. Selected physicochemical properties of native and denatured protein isolates from faba beans, soybeans, and sunflower seed. Food/Nahrung, 25(1), 59–69. https://doi.org/10.1002/food.19810250109
- Shen, Y., Hu, R., & Li, Y. (2019). Antioxidant and emulsifying activities of corn gluten meal hydrolysates in oil‐in‐water emulsions. Journal of the American Oil Chemists’ Society, 97, 175–185. https://doi.org/10.1002/aocs.12286
- Tang, C., & Ma, C. (2009). Effect of high pressure treatment on aggregation and structural properties of soy protein isolate. Lebensmittel-Wissenschaft und -Technologie- Food Science and Technology, 42(2), 606–611. https://doi.org/10.1016/j.lwt.2008.07.012
- Tian, Y., Zhao, J., Xie, Z., Wang, J., Xu, X., & Jin, Z. (2014). Effect of different pressure-soaking treatments on color, texture, morphology and retrogradation properties of cooked rice. LWT-Food Science and Technology, 55(1), 368–373. https://doi.org/10.1016/j.lwt.2013.09.020
- Wang, X., Tang, C., Li, B., Yang, X., Li, L., & Ma, C. (2008). Effects of high pressure treatment on some physicochemical and functional properties of soy protein isolate. Food Hydrocolloids, 22(4), 560–567. https://doi.org/10.1016/j.foodhyd.2007.01.027
- Xiong, T., Xiong, W., Ge, M., Xia, J., Li, B., & Chen, Y. (2018). Effect of high intensity ultrasound on structure and foaming properties of pea protein isolate. Food Research International, 109, 260–267. https://doi.org/10.1016/j.foodres.2018.04.044
- Xu, A., Alashi, A., Gbadamosi, S., Taiwo, K., Oyedele, D., Adebooye, O., Aluko, R. E. (2020). Comparative study of the structural and functional properties of protein isolates prepared from edible vegetable leaves. International Journal of Food Properties, 23(1), 955–970. https://doi.org/10.1080/10942912.2020.1772285
- Xu, Y., Sismour, E., Tucker, F., Rasberry, J., Zhao, W., Rao, Q., Zhao, Y., Haff, R., Yousuf, A., Gao, M., & Chen, A. (2024). Structural and functional properties of kabuli chickpea protein as affected by high hydrostatic pressures. ACS Food Science & Technology, 4(2), 528–536. https://doi.org/10.1021/acsfoodscitech.3c00640
- Yin, S., Tang, C., Wen, Q., Yang, X., & Li, L. (2008). Functional properties and in vitro trypsin digestibility of red kidney bean (Phaseolus vulgaris L.) protein isolate: Effect of high-pressure treatment. Food Chemistry, 110(4), 938–945. https://doi.org/10.1016/j.foodchem.2008.02.090
- Zengin, K., Ozel, B., Oztop, M., & Alpas, H. (2024). Effect of high hydrostatic pressure on the functional properties of soy protein isolate. Journal of Food Process Engineering, 47(3), e14578. https://doi.org/10.1111/jfpe.14578
- Zhang, Z., Li, Y., Lee, M., Ravanfar, R., Padilla-Zakour, O., & Abbaspourrad, A. (2020). The impact of high-pressure processing on the structure and sensory properties of egg white-whey protein mixture at acidic conditions. Food Bioprocess Technology, 13(2), 379–389. https://doi.org/10.1007/s11947-019-02397-6
- Zhao, F. (2019). Effects of physical pretreatment on structure and physicochemical properties of soybean protein isolate. Shandong Agricultural University, Taian, 53–62.
- Zheng, J., Yin, H., Shen, C., Zhang, L., Ren, D., & Lu, J. (2020). Functional and structural properties of spirulina phycocyanin modified by ultra-high-pressure composite glycation. Food Chemistry, 306, 125615. https://doi.org/10.1016/j.foodchem.2019.125615
- Zheng, X., Liu, X., Yu, S., Wang, X., Ma, Y., Yang, S., & Jing, S.-S. (2014). Effects of extrusion and starch removal pretreatment on zein proteins extracted from corn gluten meal. Cereal Chemistry, 91(5), 496–501. https://doi.org/10.1094/CCHEM-07-13-0141-R
- Zheng, X., Wang, J., Liu, X., Sun, Y., Zheng, Y., Wang, X., & Liu, Y. (2015). Effect of hydrolysis time on the physicochemical and functional properties of corn glutelin by protamex hydrolysis. Food Chemistry, 172, 407–415. https://doi.org/10.1016/j.foodchem.2014.09.080
- Zhou, Y., Quyang, B., Du, L., Wei, Y., Zhou, X., Xiao, Y., & Wang, Y. (2022). Effect of ultra-high-pressure treatment on the structural and functional properties of buckwheat 13S globulin. Food Science and Human Wellness, 11(4), 895–903. https://doi.org/10.1016/j.fshw.2022.03.008
- Zou, H., Xu, Z., Zhao, L., Wang, Y., & Liao, X. (2019). Effects of high pressure processing on the interaction of α-lactalbumin and pelargonidin-3-glucoside. Food Chemistry, 285, 22–30. https://doi.org/10.1016/j.foodchem.2019.01.129