ABSTRACT
The production and quality of wheat are affected by abiotic constraints including water stress and soil nutrient deficiencies. It is imperative to develop drought-tolerant wheat varieties with high yield potential and enhanced grain protein content for food security. Silicon (Si) is important for plant growth and development but its role in abiotic stress tolerance has been overlooked in breeding programs. Identifying the underlying functional genes controlling drought tolerance, protein content and grain yield is essential for wheat improvement, especially under drought stress. Silicon uptake is conditioned by several Si transporter genes such as Lsi1, Lsi2 and Lsi6 and aquaporins, which facilitate transport of silicon and water between cells. The objectives of this review are to examine the role of Si in improving plant nutrition and drought tolerance, and to appraise the genetic control of Si uptake and breeding methods for improving Si uptake for drought adaptation and improved grain yield and quality. The review highlights the limited progress made in breeding for drought tolerance in wheat, especially in sub-Sahara Africa where the challenge is prevalent. Limited understanding of the genetic basis for Si uptake and physiology contribute to the limited progress in its exploitation in wheat improvement programs.
Introduction
Bread wheat (Triticum aestivum L., 2n = 6x = 42, AABBDD) is one of the most widely cultivated and traded crops in the world. The global production of wheat is estimated at 215 million hectares with an output of 763 million tonnes of grain (CGIAR Citation2017). It accounts for over 35% of the global food demand, providing carbohydrates, proteins, minerals and vitamins (Igrejas and Branlard Citation2020). Wheat has a superior nutritional value, containing 60–80% starch and 8–15% protein, compared to maize (60% starch, 9–10% protein) or rice (80% starch, and 7–8% protein) (Nevo et al. Citation2002; Chaudhari et al. Citation2018). However, wheat production in most parts of the world is projected to fall short of demand due to several abiotic constraints, including the multiple impacts of climate change, and poor soil fertility.
Water availability and poor soil fertility are particularly important constraints to wheat production in sub-Sahara Africa (SSA) (Fahad et al. Citation2017). Due to climate change, the average rainfall in SSA countries has decreased, and rainfall distribution has become more erratic (Worku et al. Citation2018). Dryland wheat production is carried out under winter rainfed systems that depend on residual moisture availability. However, low rainfall and erratic rainfall patterns impact on winter rainfed production systems, especially when terminal drought stress occurs post-anthesis (Shavrukov et al. Citation2017). The progressive decline in rainfall also limits the availability of water for irrigated systems. The widespread distribution of degraded soils with poor mineral content exacerbates the impact of drought stress on wheat production (Assefa et al. Citation2018). Most soils in SSA are degraded, acidic and highly leached of essential nutrients to support sustainable crop production, including low levels of plant-available Si (Stewart et al. Citation2020). It is imperative to develop drought-tolerant wheat varieties for SSA, coupled with improved nutrient absorption capacity, that can better tolerate production under conditions of drought stress and soils with poor mineral fertility.
Developing drought-tolerant varieties has been pursued using various methods, including selection for agronomic traits and grain yield, genetic markers, and genomic selection, with various levels of success. One agronomic trait that has gained recent attention is silicon (Si) uptake. Silicon is a micronutrient that has been shown to improve agronomic performance and nutrient uptake in some plant species under abiotic and biotic stresses. Improved agronomic performance is important for drought tolerance, while enhanced nutrient uptake is vital to ensure high grain quality. Despite information on the role of Si in plant nutrition and drought tolerance, genetic variation for Si uptake has not been exploited in breeding programmes, especially those aiming to improve drought tolerance and grain quality. The hypothesis of this review is that increased Si uptake in wheat will improve nutrition and water uptake, resulting in improved grain yield and quality under a range of stress conditions. Plant breeders have not specifically set a breeding objective to exploit the potential role of Si in improving plant nutrition and drought stress tolerance. The impact of enhanced Si uptake by the plant on grain quality has also been highlighted as a potential benefit. However, Si uptake efficiency has not been widely studied in wheat breeding, compared to investigations for other agronomic traits. The main reason for the limited exploitation of Si in wheat breeding programmes is a lack of knowledge on the role of Si, the nature of the underlying genetics and the development of suitable selection methods. Therefore, the objectives of this review are to examine the role of Si in improving plant nutrition and drought tolerance, and to appraise the current knowledge of genetic control of Si uptake, and breeding methods for improving Si uptake for drought tolerance, and improved grain yield and quality.
Silicon uptake mechanism in plants
Silicon mostly exists in the soil in solid crystalline forms such as quartz and micas, microcrystalline minerals or as secondary silicate minerals (Cornelius and Delvaux Citation2016; Souri et al. Citation2020). Under aqueous conditions, mineral Si dissolves to form monosilicic and polysilicic acids (H4SiO4) (Sauer et al. Citation2006). Polysilicic acid is predominant at early stages of dissolution, while monosilicic acid is the more abundant at low saturations. Silicic acid is taken up via the primary and secondary root hair of the plant root system (). From the root hairs, it moves via cortical cells to the xylem using influx and efflux transporter genes (Ma et al. Citation2011) and into plant tissues using silicon transporters conditioned by a number of genes, such as those denoted as Lsi1, Lsi2, Lsi3, and Lsi6 (Gaur et al. Citation2020). There are species-specific variations in the uptake, movement and deposition of Si in plant tissues. For instance, species of the family Gramineae tend to deposit Si in endodermal cell walls and stegmata cells of sclerenchyma fibres. Endodermal silicification occurs in a radial pattern, mainly in storage tissues, vascular tissues, sclerenchyma, fibres and epidermal tissue (Mandlik et al. Citation2020). The specific location of the Si deposits facilitate improved root strength, root length, thickness, volume, biomass, lateral root numbers, nodulation and nitrogen fixation (Lux et al. Citation2020). These morphological changes allow roots to penetrate enhanced soil depths for effective nutrient and water uptake, and improved plant anchorage. Silicon may also be deposited in cell wall polysaccharides, increasing cell wall strength and protecting against plant pathogens (Guerriero et al. Citation2016).
Figure 1. The uptake and distribution of Si from soil through to the deposition in silicified cell walls and silica bodies in wheat. LSi 1, LSi 2 and LSi 6 denote genes that condition Si influx and efflux transporters, Si (OH)4 = orthosilicic acid. Source: Adapted from Farooq and Dietz, (Citation2015).
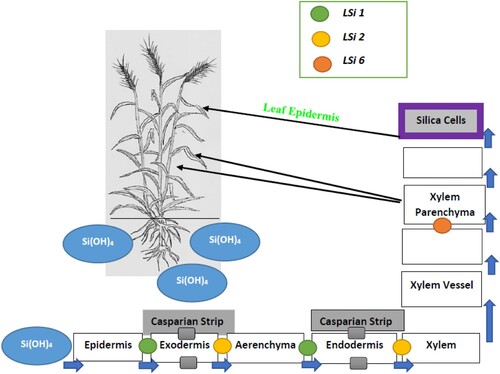
Silicon deposits in above-ground plants parts have been associated with improved photosynthetic activity, and decreased insect and herbivore damage (Ma and Yamaji Citation2008). The Si is deposited to form a silicon bilayer between the cuticle and leaf epidermis of young and mature leaves, which is associated with an increased content of cellulose, fibre and lignin. The Si deposits also enhance physiological activities such as gaseous exchange, maintenance of leaf water potential, enhanced water and nutrient transportation, and improved carbohydrate production, which are critical processes in plant growth and development (Gou et al. Citation2020).
Silicon uptake by plants is influenced by plant genetics, Si particle size, soil conditions and climatic factors. Previously, it was proposed that Si uptake was achieved through active, passive or rejective uptake (Takahashi et al. Citation1990; Ma et al. Citation2001). Active uptake of Si occurs via the root system, whilst passive Si uptake refers to the absorption of Si through stems and leaves (Chiba et al. Citation2009; Hemmati Citation2017). Examples of crop species that actively take up Si are rice and wheat. Solanaceous crops were previously considered to reject Si uptake (Ma et al. Citation2001). However, different modes of Si uptake and transport have since been proposed using studies on rice and Arabidopsis (Brugiére and Exley Citation2017; Putra et al. Citation2020). Silicon concentration varies from 0.1 to 10% of the plant dry matter in different plant species and genotypes (Epstein Citation1999). Broadly, crops can be divided into three categories based on their ability to accumulate Si in their tissue. One category consists of high Si accumulators that have the ability to absorb Si efficiently. High Si accumulators contain >1% Si on a dry weight basis. Moderate or intermediate Si accumulators are categorised as plants with a Si content of between 0.5 and 1% of plant dry weight. Non-Si accumulators are plants with a limited ability to extract Si from the soil, or to deposit the silicon in their tissues. Non-accumulators have Si concentration below 0.5% of plant dry weight (Liang et al. Citation2007; Meena et al. Citation2021).
In the soil, the availability of Si depends on the Si particle size, soil pH, soil temperature, concentration of cations such as magnesium, potassium and calcium in the soil, soil organic matter and moisture content. The monosilicic acid molecule is the simplest form, made up of Si bound to four hydroxyl groups in a tetrahedral shape (Alvarez and Sparks Citation1985). This is the easiest form of Si for plant uptake. It usually occurs in the soil at low concentrations of below 100 mg kg−1 soil, while soil Si concentrations between 100 and 200 mg kg−1 can cause aggregation of these monomers to form large molecules (Currie and Perry Citation2007). The Si uptake by plants decreases when negatively charged polymers larger than 1-3 nm are formed. The formation of these polymers from monosilicic acid depends on soil conditions, especially soil pH (Rashad and Hussien Citation2018).
The growth stage at which Si is applied as a fertiliser influences the levels of Si uptake by plants (Maghsoudi et al. Citation2016). Dorairaj et al. (Citation2020) found that the application method and formulation of Si fertiliser, and the growth stage at which Si fertiliser was applied, had a significant impact on the effectiveness of Si fertiliser on rice growth and development. Application of Si at sowing improved germination (Siddiqui and Al-Whaibi Citation2014), while applications at tillering and anthesis provided significant tolerance to drought stress (Maghsoudi et al. Citation2016). Split applications of Si at transplanting, tillering and panicle initiation in rice was found to be the most effective approach to improve plant growth (Rehman et al. Citation2019). The Si application must coincide with important growth stages to improve physiological functions, and to catalyse a range of biological processes, including nutrient uptake and assimilation. In addition, the formulation of Si is also important. In general, soil-applied Si was more effective in improving plant growth than foliar-applied Si (Cacique et al. Citation2013). Silicon fertilisers are available as granular, powder or liquid formulations. Granules are applied to the soil and are taken up by the plant through the root system after dissolution. The effectiveness of granular Si is affected by soil conditions that control soil biogeochemical reactions and the root characteristics of the plant. Liquid formulations can be applied as drench or foliar sprays. The effectiveness of foliar applied Si is affected by ambient conditions such as temperature and wind, and plant characteristics (Laane Citation2018). Silicon fertilisers have been used to increase Si availability to plants, but effective uptake of Si is affected by the method of Si application, Si formulation, its particle size and concentration, the plant’s growth stage at application and genotypic differences. The distribution of foliar-applied Si is limited to plant leaves, whereas soil-applied Si is assimilated and distributed throughout the plant (Pilon et al. Citation2013). In addition, the uptake of foliar-applied Si in plants is lower compared to soil-applied Si (Cacique et al. Citation2013). Roots are more effective at assimilating Si due to their specialised role and adaptation to acquire nutrients. It would be valuable to determine the genetic basis of root traits in wheat that result in enhanced Si uptake. There are indications that root traits have been neglected in wheat breeding (White et al. Citation2015; Fradgley et al. Citation2020; Svoboda et al. Citation2020), which could compromise nutrient uptake for improved drought tolerance, grain yield and quality breeding. The factors that affect the uptake of Si must be considered to devise suitable strategies for effective selection and breeding of genotypes with an enhanced capacity for Si uptake. For instance, the Si formulation and frequency of application must be consistent to ensure repeatability of phenotyping in multi-environment trials. And these need to relate to the commercial realities of the costs of various formulations of Si fertilisers and their application to a relatively low-value field crop such as wheat.
Silicon fertilisation has been used in hydroponic and field systems to boost plant growth (Gerami and Rameeh Citation2012). However, very few incidences of Si toxicity have been reported (Kamenidou et al. Citation2008; Mburu et al. Citation2016). Mburu et al. (Citation2016) found that weekly applications of between 500 and 1000 mg Si had phytotoxic effects on bananas. Stunting, flower deformation and delayed flowering were observed in sunflowers after application of high concentrations of sodium silicate (Kamenidou et al. Citation2008). However, Kamenidou et al. (Citation2010) could not determine whether the stunting in gerbera flowers could be attributed entirely to Si, or to the sodium (Na) effects, which are well known to induce stunting when applied in excess. Excessive application of Si is also discouraged in fodder crop production. Elevated levels of silica and lignin reduce palatability and digestibility of stover used as animal fodder, which would require chemical treatment to improve nutrient availability to livestock (Aquino et al. Citation2020).
Silicon (Si) fertilisation and its role in improving drought tolerance and grain quality
Silicon (Si) is the second most abundant element on earth and its role in plant nutrient uptake, nutrient remobilisation, and protection against biotic and abiotic stresses has received increased attention. Silicon is involved in the physiological activity and structural integrity of higher plants exposed to abiotic and biotic stresses (Liang et al., Citation2013). A series of biochemical reactions including the release of oxidative chemicals are activated in plants under stress. Silicon alleviates stress by altering osmolyte concentrations and enhancing the production of anti-oxidant enzymes to break down oxidative chemicals (Sapre and Vakharia Citation2016). For instance, the germination rate under drought stress conditions can be increased by up to 24% by applying Si as a seed priming agent (Shi et al. Citation2016; Ayed et al. Citation2021).
The role of Si in conferring drought tolerance and improving nutrient uptake in plants has been inferred from its role in maintaining plant water balance, photosynthetic activity and the turgidity of leaves and xylem under high transpiration (Melo et al. Citation2003; Hattori et al. Citation2005; Ahmad et al. Citation2007). Water balance in a plant is important to maintain cell turgidity that drives cell expansion and division for growth. Phenotypic traits such as plant height, dry matter production, leaf area, shoot growth, plant shoot nutrient uptake and thousand grain weight in wheat that are all positively correlated to grain yield depend on cell expansion (Gong et al. Citation2003). Yield increase due to Si uptake is partially as a result of enhanced chlorophyll concentrations, sustained photosynthetic activity and the maintenance of leaf architecture for effective light interception (Abbas et al. Citation2017). Si deposits appear to play a role in preventing the collapse of xylem vessels and maintaining stomatal conductance under drought and heat stresses, which is important to sustain water and nutrient transport throughout the plant under stress conditions (Sapre and Vakharia Citation2016).
There are indirect effects of Si on yield that accrue through improved accumulation of macro and micro-nutrients in plants with improved Si uptake (Neu et al. Citation2017). Improved carbon (C) and nitrogen (N) assimilation due to increased Si uptake result in an increased tolerance to both biotic and abiotic stresses in wheat. Improved C sequestration and assimilation in cellulose and carbohydrate production in grass species increases the energy efficiency of Si accumulators (Schoelynck et al. Citation2010). Most research on Si uptake has focused on improving agronomic yield, mitigating biotic and abiotic stresses, and improving nutrient uptake (). Grain quality improvement is usually inferred as an indirect effect of nutrient uptake and stress alleviation. However, the role of Si uptake in grain quality has not been investigated thoroughly.
Table 1. The effect of different Si formulations and concentrations on nutrient uptake, agronomic traits, and abiotic stresses of various crops.
The rheological properties of wheat are important indicators of grain quality. These properties include starch, lipids, and protein content, which are the major components of the wheat endosperm, and minerals, vitamins and anti-oxidants (Kumar et al. Citation2016). Enhanced Si uptake in wheat maintains the balance of nitrogen and carbon, which is important in ensuring high grain quality under stressed conditions. The balance of carbon and nitrogen is important in the synthesis of the gliadins and glutenin, which are the main components of protein gluten in wheat. The integrity of the protein gluten is also maintained by disulphide bonds, making sulphur an integral component (Réthoré et al. Citation2020). Therefore, since Si uptake has cascading effects on carbon, nitrogen, and sulphur uptake, it indirectly affects the rheological properties and grain quality of wheat produced under stress conditions. The inclusion of selection for enhanced Si uptake as a breeding objective in wheat will improve plant growth and nutrient acquisition, especially in marginal soils that are highly depleted of micro and macro-nutrients, including Si. Some countries in Asia and the Americas incorporate Si fertilisation as standard practices for sustainable agricultural crop production (Yan et al. Citation2018). While this is feasible in developed countries, the sub-optimal application of fertilisers in developing countries remains a widespread challenge due to the lack of financial resources and access to agricultural inputs for most of these farmers. The incorporation of Si in inorganic fertiliser could enhance wheat productivity in developing countries but the impact would be limited for smallholder farming communities if they cannot afford the fertilisers. It is thus important to breed for enhanced Si uptake and utilisation in wheat cultivars for deployment among resource poor farming communities, and also for regions where Si is not incorporated into popular fertilisers.
Silicon genes and aquaporins and their potential use in marker-assisted breeding
The genetic basis of Si uptake and distribution has been investigated most intensively in rice and Arabidopsis (Ma et al. Citation2006; Brugiére and Exley Citation2017; Putra et al. Citation2020), which have less complex and smaller genomes than wheat. The uptake and distribution of Si in plant tissue is controlled by Si transporter genes, namely, low silicon 1 (Lsi1) and low silicon 2 (Lsi2) (Rao and Sushmitha Citation2017). The Lsi1 belongs to the nodulin 26-like major intrinsic protein (NIP) III subgroup and is a permeable channel, while Lsi2 is a member of an uncharacterised anion transporter family that acts as an efflux transporter (Ma and Yamaji Citation2015). The transporter Lsi1 belongs to the aquaporin family that controls Si accumulation and is expressed in plant roots (Luu and Maurel Citation2005; Ma et al. Citation2006). The Lsi1 and Lsi2 transporters are situated at different sites on the plasma membrane of root cells and are integral for the effective movement of Si within the exodermis and endodermis or root cells (Ma et al. Citation2006; Hernandez-Apaolaza Citation2014). Low silicon genes encode for the influx (Lsi1 and Lsi6) and efflux (Lsi2 and Lsi3) transport proteins (Ma et al. Citation2006; Ma and Yamaji Citation2015). These genes were originally found in rice, but have also been found in other crops, including tomato (Solanum lycopersicum L.), sorghum (Sorghum bicolor [L.] Moench), grape (Vitis vinifera L.) and maize (Zea mays L.) (Vatanserver et al. Citation2017). Aquaporins (membrane water transport proteins) facilitate Si uptake in the root from soil and radial transportation within the root and xylem. These transporter genes and aquaporins have been found in various crops where they facilitate Si uptake and distribution (). The expression of Lsi1 and Lsi2 genes varies significantly. The expression levels of Si transporter genes have been correlated with the levels of Si absorption in rice (Ma et al. Citation2007). Despite the discovery of these genes, their introgression in new breeding populations has not been pursued for improving drought tolerance or grain quality in wheat.
Table 2. List of reported aquaporins and transporter genes which facilitate Si uptake and distribution in various crops.
Si-utilisation is also facilitated by aquaporins that are responsible for balancing water relations in plant cells during water stress (Zargar et al. Citation2019). Aquaporins are membrane channels from a family of major intrinsic proteins, approximately 26–30 kDa in size, that facilitate the transport of different substrates, including small neutral solutes such as H4SiO4, urea and water across membranes (Xu et al. Citation2013). Aquaporin expression is influenced by water availability but is generally higher in leaves than in roots and is lowest in shoots (Nada and Abogadallah Citation2014; Gambeta et al. Citation2017). Aquaporins are located in the peribacterioid membrane of N2 fixing root nodules (NIPs); the cell membrane (plasma membrane intrinsic proteins (PIPs)); endoplasmic reticulum (small basic intrinsic membranes (SIPs)) and tonoplasts (tonoplast intrinsic proteins (TIPs)) (Nada and Abogadallah Citation2014).
The number of aquaporin locations signify their integral role in regulating water movement, even at a cellular level. The aquaporins limit plant water loss to the soil environment by rapidly down-regulating under water-stressed conditions (Vandeleur et al. Citation2005), and their expression can be exploited in marker-assisted breeding for drought stress tolerance (Pandey et al. Citation2013). Approximately, 35 aquaporin genes have been identified in the wheat genome (Huang et al. Citation2014). Understanding the role of aquaporins in root hydraulic control can contribute to effective genotypic selection (Gambeta et al. Citation2017). Although the Lsi1 gene is associated with aquaporins (NIP III subgroup), the Lsi2 and Lsi3 and Lsi6 genes have not yet been associated with any aquaporins. Establishing whether aquaporins are associated with these genes could potentially facilitate effective selection for Si use efficiency in wheat breeding.
The NIP2 subfamily of aquaporins can be used to predict Si uptake in different plant species using a unique amino acid selective filter (Deshmukh and Bélanger Citation2016). Marker-assisted breeding based on the genetic make of the amino acid filter will improve selection efficiency for improving Si uptake in wheat. Ma (Citation2004) mapped the markers RM5303 and EST-PCR marker E60168 on Chromosome 2, which were linked to the Si transporter gene in rice. Identifying similar or related markers in wheat will open opportunities for selection of the transporter genes for improved drought tolerance in wheat, considering that homoeologous relationships exist between rice and wheat (Ahn et al. Citation1993). Genome-wide analysis studies have shown that aquaporins are important in drought stress management and improving breeding programmes (Putpeerawit et al. Citation2017; Madrid-Espinoza et al. Citation2018). Genetic sequencing to screen wheat genotypes for silicon genes and aquaporins is a promising approach to accelerate the introgression of genes for enhanced silicon uptake to improve both drought tolerance and grain quality. There is a need for further research to establish whether Si use efficiency would be improved by selecting for Si uptake genes only, or whether they should be selected for in combination with selected aquaporins.
Breeding for drought tolerance
Breeding for drought tolerance has long been identified as a sustainable and economic strategy to improve wheat production and productivity in marginal regions, especially as farmers try to cope with the impacts of climate change. It has been shown that drought tolerance can be improved by indirectly selecting for traits such as early flowering, plant height, tiller number, thousand kernel weight and grain yield (Mwadzingeni et al. Citation2016b). Early flowering has been the most exploited trait for drought escape. This allows genotypes to complete their life cycle before the onset of catastrophic drought stress. This strategy is effective in environments such as SSA where terminal drought stress is common due to the farmers’ dependence on winter rainfall and residual moisture. The main challenge encountered when breeding for drought tolerance using this strategy is that early flowering can lead to a yield penalty and reduction in other agronomic traits such as plant height and plant biomass, due to the shortened plant life cycle.
Other strategies to manage drought such as slowing biological processes, or by enhancing drought tolerance by accumulating solutes such as proline, have been pursued but with relatively little success (Mwadzingeni et al. Citation2016b). High proline accumulation under drought stress has been identified as a proxy trait for drought tolerance but its use has been limited by difficulties encountered in chemical analysis (Maghsoudi et al. Citation2018). In addition, the genetic basis of these strategies is not well understood. There is a need for innovative approaches to incorporate other traits and strategies to accelerate breeding progress. For instance, Si has been reported to improve growth in drought-stressed plants through its role in stabilising the leaf membrane, maintaining high relative water content, enhancing antioxidant production and reducing oxidative damage (Ahmed et al. Citation2016). However, Si uptake has not been used as a proxy trait for drought tolerance breeding.
Drought tolerance is a polygenic trait and is subject to genotype by environment interactions, limiting the genotype response to selection efficiency during phenotyping (Souza et al. Citation2004). The slow progress in developing drought-tolerant cultivars has also been attributed to a lack of robust and repeatable methods for screening and maintaining of consistent drought stress conditions (Nhemachena and Kirsten Citation2017). As a result, higher genetic gains for yield have been achieved under optimal growing conditions but not as efficiently under drought stress conditions (Ahmed et al. Citation2007).
Selection of genotypes under both drought-stressed and non-stressed drought conditions has the potential to achieve high genetic gains during selection for developing wheat cultivars for the drought-prone environments (Trethowan et al., Citation2002). Screening for drought-tolerant genotypes has been achieved using drought-based grain yield indices under drought conditions in comparison to non-stressed conditions. Despite the associated large environmental variances, phenotyping remains fundamental in selecting wheat genotypes that exhibit drought adaptive and constitutive characteristics (Monneveux et al. Citation2012; Passioura Citation2012; Mwadzingeni et al. Citation2016a). Evaluating a large panel of genotypes in representative test environments and using robust selection tools has been proposed to counter genotype by environment interactions that confounds phenotypic selection. Multiple environment trials enable the quantification of genetic and environmental components, and the calculation of yield stability (Mohamed Citation2013). Previously, selection for drought tolerance was mainly based on grain yield per se, but the inclusion of selection indices has improved selection efficiency (Crespo-Herrera et al. Citation2018). For instance, indices such as the mean productivity index, stress susceptibility index and stress tolerance index have been developed for improving selection efficiency. These indices have been developed to improve selection efficiency and assist in identifying drought-tolerant genotypes (Grzesiak et al. Citation2019).
Selection for drought tolerance is complex and confounded by environmental variance. Hence, conventional breeding must be complemented with molecular breeding to improve selection efficiency. Natural and controlled environments can be used during phenotypic screening to account for environmental variance (Mwadzingeni et al. Citation2016b). Subsequently, molecular data of the genotypes can be generated to identify any markers linked to drought tolerance. The phenotypic and genetic data can then be used in combination to establish marker-trait associations for marker-assisted selection (Motawea et al. Citation2015). The availability of genetic data has improved tremendously in recent years due to the advent of the next generation sequencing (NGS) technologies such as genotyping by sequencing (GBS) for single nucleotide polymorphism (SNP) discovery (Tian et al. Citation2015). However, the lack of computing capabilities to analyse large-scale data and large panels of individuals remains an obstacle for the full utilisation of these technologies in resource-poor countries.
Successful breeding for drought tolerance will depend on the availability of adequate genetic variation for selection. Genetic variation for agronomic traits that could be useful for improving tolerance to drought has been reported previously (Ghaed-Rahimi et al. Citation2017). World wheat genetic resources are available for improving drought tolerance in locally adapted varieties, including modern and obsolete cultivars, landraces, breeding populations and wild relatives, and are maintained and catalogued by several international and national research programmes and genebanks (Mwadzingeni et al., Citation2017). National research programmes should consider assessing the genetic variation for Si uptake and its impact on drought tolerance among the germplasm maintained at these centres to augment other drought tolerance breeding programmes. This approach will add a different dimension to the current efforts and will contribute to genetic gains in drought tolerance breeding.
Integrating silicon-use efficiency in breeding programmes for improved grain yield and quality under stress conditions
Successful breeding for enhanced Si uptake depends on the availability of genetic variation for Si uptake in the breeding population. The effect of Si is most widely studied under stressed conditions (Zargar et al. Citation2019). Genetic variation for Si-utilisation under drought stress conditions has not been widely exploited for improving drought tolerance, despite knowledge of its important role in plant nutrient uptake and remobilisation, and protection against biotic and abiotic stresses (Gokulraj et al. Citation2018; Prasanna et al. Citation2018). McLarnon et al. (Citation2017) reported significant genotypic variation for Si uptake in grasses. The authors discovered that Si uptake increased under stress, which enhanced drought tolerance. Enhanced Si uptake could be combined with other drought response mechanisms such as drought escape, avoidance, and tolerance to improve wheat productivity in marginal conditions. Efficient Si uptake can be used as a proxy trait for drought tolerance and improved nutritional quality simultaneously. Selecting parental lines with superior Si uptake could provide a basis for developing breeding populations for improved Si uptake. There are indications that Si-use efficiency in wheat is a heritable trait, controlled mostly by additive genes (Jackson et al. Citation2019), which provide opportunities for early generation selection.
The role of Si in improving drought tolerance in cereal crops and its additive inheritance pattern opens opportunities for exploitation in breeding for drought tolerance and grain quality in wheat. For instance, mandatory incorporation of Si in fertiliser could be encouraged because Si-enhanced fertiliser application could be adopted by farmers as a way to increase yields, grain quality and enhance drought tolerance. However, it would then be imperative for breeders to identify and exploit genetic variation for Si uptake to develop varieties that will efficiently utilise the applied Si for improved drought tolerance, yield and grain quality. This will have positive and cascading effects on wheat productivity, food security and economic gains. Si-use efficiency research has predominantly focused on rice, with recent genome-wide studies being carried out on multiple crops (Vatanserver et al. Citation2017).
There are efforts to elucidate the genetic basis and inheritance of Si-use efficiency, which will facilitate the establishment of successful breeding programmes (Jackson et al. Citation2019). However, the large and complex wheat genome could pose a significant challenge in breeding for Si-use efficient wheat. So far, the genetic basis underlying Si uptake and its inheritance have not been established. It is, thus, important to draw insights on the underlying genetics of Si in other crops and infer possible implications for wheat breeding.
Most studies on Si uptake focused on agronomy and physiological responses. However, breeding methods and genetic control of Si uptake in wheat can be improved by adapting knowledge from other related cereal crops, particularly rice. Reportedly, Si uptake is a polygenic trait and its inheritance is conditioned by many minor genes (Gómez-Merino et al. Citation2020). Also, the expression of Si uptake is highly affected by genotype by environment interactions, suggesting the need for multi-environmental selection of test genotypes to identify varieties that are efficient in Si uptake, and are high yielding and stable candidates for production or breeding. Due to the additive genetic effect for Si uptake, this review proposes early generation selection of candidate lines after initial crosses are performed. This selection method would enable high genetic gains for superior Si uptake and yield response. Recurrent selection for transgressive segregants would enhance the response to selection for Si uptake. Simultaneous selection for Si uptake, and root biomass and root hairs (which house the Si transporters) would reduce linkage drag of undesirable genes, if any. This may need other complementary breeding tools including mutation induction and molecular marker-assisted breeding. Genetic progress in the breeding for Si uptake is limited by differential responses to different Si formulations, concentrations and rates of Si application, and a lack of knowledge on the genetics of Si-uptake efficiency and genotype by environment interaction effects.
Outlook and recommendations
The main conclusions drawn from this review are:
Drought stress and poor soil fertility are major challenges to crop production in SSA, especially in the context of the growing climate crisis, driving the need for climate-smart agriculture and improved varieties.
The narrowing crop genetic diversity in modern varieties is likely to hinder progress in breeding for drought tolerance and Si uptake.
There is a need to harness the genetic diversity of crop species and selection for novel traits such as enhanced Si uptake and improved root systems.
Enhancing Si-uptake efficiency in crop species such as wheat is a novel strategy to improve nutrient acquisition and utilisation. This is vital in SSA, where inorganic fertiliser use is low.
Genetic management of Si uptake in wheat requires greater knowledge of the genes involved, and the nature of gene action for Si uptake, to guide selection.
Transgressive selection, marker-assisted and mutation breeding techniques can be used to develop wheat ideotypes with superior Si-uptake efficiency and superior agronomic performance.
Gene editing using candidate genes such as the Lsi1 gene in a reference wheat genome could be a possible strategy to induce elevated Si uptake.
Application of Si fertilisers in a range of formulations could be used to improve its availability in SSA soils with low levels of plant-available Si. However, these would need to be affordable and cost-effective Si sources to make this approach viable.
Future research direction includes standardising and developing high throughput phenotyping platforms for Si uptake evaluation, optimising Si concentration and formulations for the target production environments, identifying QTLs governing Si uptake for marker-assisted selection, and developing breeding populations with high Si uptake efficiency, drought tolerance and enhanced grain yield and quality.
The above strategies will aid in the development and deployment of silicon-uptake efficient, drought-tolerant and high-quality wheat genotypes to promote sustainable production in drought-prone agro-ecologies.
Acknowledgements
The authors acknowledge the University of KwaZulu Natal for academic and research support, the National Research Foundation of South Africa (NRF) through the Agricultural Research Council-Small Grain Institute (ARC-SGI) for a bursary and research support from the Rockefeller Foundation through the African Centre for Crop Improvement at University of KwaZulu-Natal.
Disclosure statement
No potential conflict of interest was reported by the author(s).
Additional information
Funding
Notes on contributors
Marylyn M. Christian
Miss Marylyn M. Christian is a doctoral student at the African Centre for Crop Improvement at the University of KwaZulu-Natal.
Hussein Shimelis
Professor Hussein Shimelis is the deputy director of the African Centre for Crop Improvement and Professor and Chair of Crop Science.
Mark D. Laing
Professor Mark D. Laing is the Director of the African Centre for Crop Improvement and Professor and Chair in Plant Pathology.
Toi J. Tsilo
Professor Toi J. Tsilo is the Senior Manager at the Agricultural Research Council: Small Grains Institute in South Africa.
Isack Mathew
Doctor Isack Mathew is a postdoctoral research fellow at the African Centre for Crop Improvement at the University of KwaZulu-Natal.
References
- Abbas T, Sattar A, Ijaz M, Aatif M, Khalid S, Sher A. 2017. Exogenous silicon application alleviates salt stress in Okra. Hort Environ Biotechnol. 58:342–349. doi:10.1007/s13580-017-0247-5.
- Ahmad F, Rahmatullah TA, Maqsood MA, Mukkram TA, Kanwal S. 2007. Effect of silicon application on wheat (Triticum aestivum L.) growth under water deficiency stress. Emir J Food Agric. 19:1–7. doi:10.9755/ejfa.v12i1.5170.
- Ahmed M, Qadeer U, Ahmed ZI, Hassan F. 2016. Improvement of wheat (Triticum aestivum) drought tolerance by seed priming with silicon. Arch Agron Soil Sci. 62:299–315. doi:10.1080/03650340.2015.1048235.
- Ahmed N, Chowdhry MA, Khaliq I, Maekawa M. 2007. The inheritance of yield and yield components of five wheat hybrid populations under drought conditions. Ind J Agric Sci. 8:53–59. doi:10.21082/ijas.v8n2.2007.
- Ahn S, Anderson JA, Sorrels ME, Tanksley SD. 1993. Homoeologous relationships of rice, wheat and maize chromosomes. Mol Gen Genet. 241:483–490.
- Alvarez R, Sparks DL. 1985. Polymerization of silicate anions in solutions at low concentrations. Nature. 318(6047):649–651.
- Aquino D, Del Barrio A, Trach NG, Hai NT, Khang DN, Toan NT, Van Hung N. 2020. Rice straw-based fodder for ruminants. In: M. Gummert, N. Van Hung, P. Chivenge, B. Douthwaite, editor. Sustainable rice straw management. Cham: Springer; p. 111–130.
- Assefa T, Jha M, Reyes M, Worqlul AW. 2018. Modeling the impacts of conservation agriculture with a drip irrigation system on the hydrology and water management in Sub-Saharan Africa. Sustainability. 10:4763. doi:10.3390/su10124763.
- Ayed S, Othmani A, Bouhaouel I, Rasâa N, Othmani S, Amara HS. 2021. Effect of silicon (Si) seed priming on germination and effectiveness of its foliar supplies on durum wheat (Triticum turgidum L. ssp. durum) genotypes under semi-arid environment. Silicon. doi:10.1007/s12633-021-00963-2.
- Bokor B, Ondoš S, Vaculik M, Bokorová S, Weidinger M, Lichtscheidl I, Turňa J, Lux A. 2017. Expression of genes for Si uptake, accumulation, and correlation of Si with other elements in Ionome of maize kernel. Front Plant Sci. 8:1063. doi:10.3389/fpls.2017.01063.
- Brugiére T, Exley C. 2017. Callose-associated silica deposition in Arabidopsis. J Trace Elem Med Biol. 39:86–90.
- Cacique IS, Domiciano GP, Moreira WR, Rodrigues FA, Cruz MFA, Serra NS, Català AB. 2013. Effect of root and leaf applications of soluble silicon on blast development in rice. Bragantia. 72:304–309. doi:10.1590/brag.2013.032.
- CGIAR. 2017. Wheat in the world. 28 April 2020. https://wheat.org/wheat-in-the-world/.
- Chaudhari PR, Tamrakar N, Singh L, Tandon A, Sharma D. 2018. Rice nutritional and medicinal properties: a review article. J Pharmacogn Phytochem. 7:150–156.
- Chiba Y, Mitani N, Yamaji N, Ma JF. 2009. Hvlsi1 is a silicon influx transporter in barley. Plant J. 57:810–818. doi:10.1111/j.1365-313X.2008.03728.x.
- Cornelius JT, Delvaux B. 2016. Soil processes drive the biological silicon feedback loop. Funct Ecol. 30:1298–1310. doi:10.1111/1365-2435.12704.
- Crespo-Herrera LA, Crossa J, Huerta-Espino J, Vargas M, Mondal S, Velu G, Payne TS, Braun H, Singh RP. 2018. Genetic gains for grain yield in CIMMYT's semi-arid wheat yield trials grown in suboptimal environments. Crop Sci. 58:1890–1898. doi:10.2135/cropsci2018.01.0017.
- Currie HA, Perry CC. 2007. Silica in plants: biological, biochemical and chemical studies. Ann Bot. 100(7):1383–1389.
- Deshmukh R, Bélanger RR. 2016. Molecular evolution of aquaporins and silicon influx in plants. Funct Ecol. 30:1277–1285. doi:10.1111/1365-2435.12570.
- Dong Z, Li Y, Xiao X, Chen Y, Shen X. 2018. Silicon effect on growth, nutrient uptake, and yield of peanut (Arachis hypogaea L.) under aluminium stress. J Plant Nutr. 41:2001–2008. doi:10.1080/01904167.2018.1485163.
- Dorairaj D, Ismail MR, Sinniah UM, Tan KB. 2020. Silicon mediated improvement in agronomic traits, physiological parameters and fiber content in Oryza sativa. Acta Physiol Plant. 42. doi:10.1007/s11738-020-3024-5
- Epstein E. 1999. Silicon. Annu Rev Plant Physiol Plant Mol Biol. 50:641–664. doi:10.1146/annurev.arplant.50.1.641.
- Fahad S, Bajwa AA, Nazir U, Anjum SA, Farooq A, Zohaib A, Nasim W, Adkins S, Saud S, Ihsan MZ, et al. 2017. Crop production under drought and heat stress: plant responses and management options. Front Plant Sci. 8:1–16. doi:doi:10.3389/fpls.2017.01147.
- Farooq MA, Dietz KJ. 2015. Silicon as versatile player in plant and human biology: overlooked and poorly understood. Front. Plant Sci. 6:994–1008. doi:10.3389/fpls.2015.00994.
- Fradgley N, Evans G, Biernaskie JM, Cockram J, Marr EC, Oliver AG, Ober E, Jones H. 2020. Effects of breeding history and crop management on the roots architecture of wheat. Plant Soil. 452:587–600. doi:10.1007/s11104-020-04585-2.
- Gambeta GA, Knipfer T, Fricke W, McElorne AJ. 2017. Root aquaporins and stress. In: F. Chaumont, S.D. Tyerman, editor. Plant aquaporins: from transport to signalling. Cham: Springer International Publishing. p. 133–153.
- Gaur S, Kumar J, Kumar D, Chauhan DK, Prasad SM, Srivastava PK. 2020. Fascinating impact of silicon and silicon transporters in plants: a review. Ecotoxicol Environ Saf. 202. doi:10.1016/j.ecoenv.2020.110885
- Gerami M, Rameeh V. 2012. Study of silicon and nitrogen effects on yield components and shoot ions nutrient composition in rice. Agriculture. 58:93–98. doi:10.2478/v10207-012-0011-x.
- Ghaed-Rahimi L, Heidari B, Dadkhodaie A. 2017. Construction and efficiency of selection indices in wheat (Triticum aestivum L.) under drought stress and well-irrigated conditions. Plant Breed Biotechnol. 5:78–87. doi:10.9787/PBB.2017.5.2.078.
- Gokulraj N, Ravichandran V, Boominathan P, Soundararajan R. 2018. Response of silicon on membrane stability, plant water status and yield of rice genotypes under drought. Int J Agric Sci. 10:6615–6618. https://www.bioinfopublication.org/jouarchive.php?opt=&jouid=BPJ0000217.
- Gómez-Merino FC, Trejo-Téllez LI, García-Jiménez A, Escobar-Sepúlveda HF, Ramírez-Olvera SM. 2020. Silicon flow from root to shoot in pepper: a comprehensive in silico analysis reveals a potential linkage between gene expression and hormone signaling that stimulates plant growth and metabolism. Peer J. 8. doi:10.7717/peerj.10053
- Gong HJ, Chen KM, Chen GC, Wang SM, Zhang CL. 2003. Effect of silicon on growth of wheat under drought. J Plant Nutr. 26:1055–1063. doi:10.1081/PLN-120020075.
- Gou T, Yang L, Hu W, Chen X, Zhu Y, Guo J, Gong H. 2020. Silicon improves the growth of cucumber under excess nitrate stress by enhancing nitrogen assimilation and chlorophyll synthesis. Plant Physiol Biochem. 152:53–61. doi:10.1016/j.plaphy.2020.04.031.
- Greger M, Landberg T. 2018. Silicon influences soil availability and accumulation of mineral nutrients in various plant species. Plants. 7:1–16.
- Grzesiak S, Hordyńska N, Szcyrek P, Grzesiak MT, Noga A, Szechyńska-Hebda M. 2019. Variation among wheat (Triticum aestivum L.) genotypes in response to the drought stress: I – selection approaches. J Plant Inter. 14:30–44. doi:10.1080/17429145.2018.1550817.
- Guerriero G, Hausman J-F, Legay S. 2016. Silicon and the plant extracellular matrix. Front Plant Sci. 7. doi:10.3389/fpls.2016.00463
- Hattori T, Inanaga S, Araki H, An P, Mortia S, Luxova M, Lux A. 2005. Application of silicon enhanced drought tolerance in Sorghum bicolor. Acta Physiol Plant. 123:459–466. doi:10.1111/j.1399-3054.2005.00481-x.
- Hemmati S. 2017. Physiological roles of aquaporins in the improvement of plant water and nutrient usage. Res Rev Biosci. 12:133–142.
- Hernandez-Apaolaza L. 2014. Can silicon partially alleviate micronutrient deficiency in plants? A review. Planta. 240:447–458. doi:10.1007/s00425-014-2119-x.
- Hu J, Li Y, Jeong BR. 2020. Putative silicon transporters and effect of temperature stresses and silicon supplementation on their expression and tissue silicon content in Poinsettia. Plants. 9:596. doi:10.3390/plants9050569.
- Huang C, Zhou S, Hu W, Deng X, Wei S, Yeng G, He G. 2014. The wheat aquaporin gene TaAQP7 confers cold tolerance in transgenic tobacco. Zeitschr Naturf C J Biosci. 69:142–148. doi:10.5560/znc.2013-0079.
- Igrejas G, Branlard G. 2020. The importance of wheat. In: G. Igrejas, T. M. Ikeda, C. Guzmán, editor. Wheat quality for improving processing and human health. Cham: Springer Nature; p. 1–8.
- Jackson VML, Rubaihayo P, Wasswa P, Hashim AT. 2019. Inheritance of silicon uptake ability in rice blast resistant varieties. Asian J Res Crop Sci. 4:1–6. doi:10.9734/ajrcs/2019/v4i230066.
- Jain S, Rai P, Singh J, Singh VP, Prasad R, Rana S, Deshmukh R, Tripathi DK, Sharma S. 2021. Exogenous application of silicon alleviates metsulfuron methyl induced stress in wheat seedlings. Plant Physiol Biochem. In press. doi:10.1016/j.plaphy.2021.07.031
- Kamenidou S, Cavins TJ, Marek S. 2008. Silicon supplements affect horticultural traits of greenhouse-produced ornamental sunflowers. HortScience. 43(1):236–239.
- Kamenidou S, Cavins TJ, Marek S. 2010. Silicon supplements affect floricultural quality traits and elemental nutrient concentrations of greenhouse produced gerbera. Sci Hortic. 123:390–394. doi:10.1016/j.scienta.2009.09.008.
- Khaliq A, Ali S, Hameed A, Farooq MA, Farid M, Shakoor MB, Mahmood K, Ishaque W, Rizwan M. 2016. Silicon alleviates nickel toxicity in cotton seedlings through enhancing growth, photosynthesis, and suppressing Ni uptake and oxidative stress. Arch Agron Soil Sci. 62:633–647.
- Kumar R, Kumar A, Sharma NK, Kaur N, Chundur V, Chawla M, Sharma S, Singh K, Garg M. 2016. Soft and hard textured wheat differ in starch properties as indicated by trimodal distribution, morphology, thermal and crystalline properties. PLoS One. 11:1–14. doi:10.1371/journal.pone.0147622.
- Laane H-M. 2018. The effects of foliar sprays with different silicon compounds. Plants. 7:1–22. doi:10.3390/plants7020045.
- Liang SJ, Li ZQ, Li XJ, Xie HG, Zhu RS, Lin JX, Xie HA, Wu H. 2013. Effects of stem structural characters and silicon content on lodging resistance in rice (Oryza sativa L.). Res. Crops 14:621–636
- Liang Y, Sun W, Zhu Y, Christie P. 2007. Mechanisms of silicon-mediated alleviation of abiotic stresses in higher plants: a review. Environ Pollut. 147. doi:10.1016/j.envpol.2006.06.008
- Ligaba-Osena A, Guo W, Choi SC, Limmer MA, Seyfferth AL, Hankoua BB. 2020. Silicon enhances biomass and grain yield in an ancient crop tef [Eragrostis tef (Zucc.) Trotter]. Front Plant Sci. 11. doi:10.3389/fpls.2020.608503
- Lin Y, Sun Z, Li Z, Xue R, Cui W, Sun S, Liu T, Zeng R, Song Y. 2019. Deficiency in silicon transporter Lsi1 compromises inducibility of anti-herbivore defense in rice plants. Front Plant Sci. 10. doi:10.3389/fpls.2019.00652
- Luu DT, Maurel C. 2005. Aquaporins in a challenging environment: molecular gears for adjusting plant water status. Plant Cell Environ. 28:85–96. doi:10.1111/j.1365-3040.2004.01295.x.
- Lux A, Lukačová Z, Vaculik M, Švubová R, Kohanová J, Soukup M, Martinka M, Bokor B. 2020. Silicification of root tissues. Plants. 9. doi:10.3390/plants9010111
- Ma JF, Miyake Y, Takahashi E. 2001. Silicon as a beneficial element for crop plants. In: L. E. Datnoff, G. H. Snyder, G. H. Korndörfer, editor. Silicon in agriculture. Amsterdam: Elsevier Science B.V; p. 17–39.
- Ma, JF. 2004. Role of silicon in enhancing the resistance of plants to biotic and abiotic stresses. Soil Sci. Plant Nutr. 50:11--18.
- Ma JF, Tamai K, Yamaji N, Mitani N, Konishi S, Katsuhara M, Ishiguro M, Murata Y, Yano M. 2006. A silicon transporter in rice. Nature. 440:688–691. doi:10.1038/nature04590.
- Ma JF, Yamaji N. 2008. Functions and transport of silicon in plants. Cell Mol Life Sci. 65:6049–3057. doi:10.1007/s00018-008-7580-x.
- Ma JF, Yamaji N. 2015. A cooperative system of silicon transport in plants. Trends Plant Sci. 20:435–442. doi:10.1016/j.tplants.2015.04.007.
- Ma JF, Yamaji N, Mitani-Ueno N. 2011. Transport of silicon from roots to panicles in plants. Proc Japan Acad Series B Physiol Biol Sci. 87:377–385. doi:10.2183/pjab.87.377.
- Ma JF, Yamaji N, Tamai K, Mitani N. 2007. Genotypic difference in silicon uptake and expression of silicon transporter genes in rice. Plant Physiol. 145:919–924. doi:10.1104/pp.107.107599.
- Madrid-Espinoza J, Brunel-Salidias N, Guerra FP, Guttiérrez A, del Pozo A. 2018. Genome-wide identification and transcriptional regulation of aquaporin genes in bread wheat (Triticum aestivum L.) under water stress. Genes. 9. doi:10.3390/genes9100497
- Maghsoudi K, Emam Y, Ashraf M. 2016. Foliar application of silicon at different growth stages alters growth and yield of selected wheat cultivars. J Plant Nutr. 39:1194–1203. doi:10.1080/01904167.2015.1115876.
- Maghsoudi K, Emam Y, Niazi A, Pessarakli M, Arvin MJ. 2018. P5CS expression level and proline accumulation in the sensitive and tolerant wheat cultivars under control and drought stress conditions in the presence/absence of silicon and salicylic acid. J Plant Inter. 13:461–471. doi:10.1080/17429145.2018.1506516.
- Malav JK, Patel KC, Sajid M. 2015. Influence of silicon fertilization on yield and nutrients uptake (Si, P, K, S and Na) of rice (Oryza sativa L.). Ecoscan. 9:629–634.
- Mandlik R, Thakral V, Raturi G, Shinde S, Nikolic M, Tripathi DK, Sonah H, Deshmukh R. 2020. Significance of silicon uptake, transport and deposition in plants. J Exp Bot. 71:6703–6718. doi:10.1093/jxb/eraa301.
- Mburu K, Oduor R, Mgutu A, Tripathi L. 2016. Silicon application enhances resistance to Xanthomonas wilt disease in banana. Plant Pathol. 65(5):807–818.
- McLarnon E, McQueen-Mason S, Lenk I, Hartley SE. 2017. Evidence for active uptake and deposition of Si-based defenses in tall fescue. Front Plant Sci. 8:1–11. doi:10.3389/fpls.2017.01199.
- Meena VD, Dotaniya ML, Saha JK, Patra AS. 2021. Silicon potential to mitigate plant heavy metals stress for sustainable agriculture: a review. Silicon. doi:10.1007/s12633-021-01170-9.
- Melo SP, Korndorfer GH, Korndorfer CM, Lana RMQ, Santan DG. 2003. Silicon accumulation and water deficient tolerance in grasses. Sci Agric. 60:755–759. doi:10.1590/S0103-90162003000400022.
- Mohamed NEM. 2013. Genotype by environment interactions for grain yield in bread wheat (Triticum aestivum L.). J Plant Breed Crop Sci. 5:150–157. doi:10.5897/JPBCS2013.0390.
- Monneveux P, Jing R, Misra S. 2012. II. 1.2 Phenotyping wheat for adaptation to drought using physiological traits. Front Physiol. 3. doi:10.3389/fphys.2012.00429
- Monpetit J, Vivancos J, Mitani-Ueno N, Yamaji N, Belzile F, Ma JF, Bélanger RR. 2012. Cloning, functional characterization and heterologous expression of TaLsi1, a wheat silicon transporter gene. Plant Mol Biol. 79:35–46.
- Motawea MH, Said AA, Khaled AGA. 2015. ISSR marker-trait associations and stability analysis in bread wheat varieties. Plant Breed Biotechnol. 3:167–177. doi:10.9787/PBB.2015.3.2.167.
- Mwadzingeni L, Shimelis H, Dube E, Laing MD, Tsilo TJ. 2016a. Breeding wheat for drought tolerance: progress and technologies. J Integr Agric. 15:935–943. doi:10.1016/S2095-3119(15)61102-9.
- Mwadzingeni L, Shimelis H, Tesfay S, Tsilo TJ. 2016b. Screening of bread wheat genotypes for drought tolerance using phenotypic and proline analysis. Front Plant Sci. 7:1276. doi:10.3389/fpls.2016.01276.
- Mwadzingeni L, Shimelis H, Rees DJG, Tsilo TJ. 2017. Genome-wide association analysis of agronomic traits in wheat under drought-stressed and non-stressed conditions. PloS one 12:e0171692.
- Nada RM, Abogadallah GM. 2014. Aquaporins are major determinants of water use efficiency of rice plants in the field. Plant Sci. 227:165–180. doi:10.1016/j.plantsci.2014.08.006.
- Neu S, Schaller J, Dudel EG. 2017. Silicon availability modifies nutrient use efficiency and content, C: N: P stoichiometry and productivity of winter wheat (Triticum aestivum L.). Sci Rep. 7. doi:10.1038/srep40829
- Nevo, E, Korol AB, Beiles A, Fahima T. 2002. Evolution of Wild Emmer and Wheat Improvement: Population genetics, genetic resources and genome organization of wheat's progenitor, Triticum dicoccoides. Springer-Verlag Inc., Germany: Berlin. p. 1–232.
- Nhemachena CR, Kirsten J. 2017. A historical assessments of sources and uses of wheat varietal innovations in South Africa. South Afr J Sci. 113:1–8. doi:10.17159/sajs.2017/20160008.
- Othmani A, Ayed S, Bezzin O, Farooq M, Ayed-Slama O, Slim-Amara H, Younes MB. 2020. Effect of silicon supply methods on durum wheat (Triticum durum Desf.) response to drought stress. Silicon. doi:10.1007/s12633-020-00639-3.
- Ouellette S, Goyette M-H, Labbé C, Laur J, Gaudreau L, Gosselin A, Dorais M, Deshmukh RK, Bélanger RR. 2017. Silicon transporters and effects of silicon amendments in strawberry under high tunnel and field conditions. Front Plant Sci. 8:949.
- Pandey B, Sharma P, Pandey DM, Sharma I, Chatrath R. 2013. Identification of new aquaporin genes and single nucleotide polymorphism in bread wheat. Evolut Bioinform. 9:437–452. doi:10.4137/EBO.S12568.
- Pascual MB, Gonzalo MJ, Hernandez-Apaolaza L. 2016. Silicon addition to soybean (Glycine max L.) plants alleviates zinc deficiency. Plant Physiol Biochem. 108:132–138.
- Passioura J. 2012. Phenotyping for drought tolerance in grain crops: when is it useful to breeders? Funct Plant Biol. 39:851–859. doi:10.1071/FP12079.
- Pilon C, Sorrato RP, Moreno LA. 2013. Effects of soil and foliar application of soluble silicon on mineral nutrition, gas exchange, and growth of potato plants. Crop Sci. 53:1605–1614. doi:10.2135/cropsci2012.10.0580.
- Prasanna YL, Rajeswari VR, Rao PR, Umamahesh V, Prasad MS. 2018. Influence of silicon solubilizers on growth and yield of rice genotypes. J Chem Stud. 6:1090–1095. https://sasapjas.org/wp-content/uploads/2019/08/234.pdf.
- Putpeerawit P, Sojikul P, Thitamadee S, Narangajavana J. 2017. Genome-wide analysis of aquaporin gene family and their responses to water-deficit stress conditions in cassava. Plant Physiol Biochem. 121:118–127. doi:10.1016/j.plaphy.2017.10.025.
- Putra R, Powell JR, Hartley SE, Johnson SN. 2020. Is it time to include legumes in plant silicon research? Funct Ecol. 34(6):1142–1157.
- Rao GB, Sushmitha P. 2017. Silicon uptake, transportation and accumulation in rice. J Pharmacogn Phytochem. 6:290–293. http://biovedjournal.org/bv29(1)/37.pdf.
- Rashad RT, Hussien RA. 2018. Studying the solubility, availability, and uptake of silicon (Si) from some ore minerals in sandy soil. SAINS TANAH J Soil Sci Agroclimatol. 15(2):69–82.
- Réthoré E, Ali N, Yvin J-C, Hosseini SA. 2020. Silicon regulates source to sink metabolic homeostasis and promotes growth of rice plants under sulfur deficiency. Int J Mol Sci. 21. doi:10.3390/ijms21103677
- Sapre S, Vakharia DN. 2016. Role of silicon under water deficit stress in wheat: (biochemical perspective): a review. Agric Rev. 37:109–116. doi:10.18805/ar.v37i2.10736.
- Sauer D, Saccone L, Conley DJ, Herrmann L, Sommer M. 2006. Review of methodologies for extracting plant-available and amorphous Si from soils and aquatic sediments. Biogeochemistry. 80(1):89–108.
- Schoelynck J, Bal K, Backx H, Okruszko T, Meire P, Struyf E. 2010. Silica uptake in aquatic and wetland macrophytes: a strategic choice between silica, lignin and cellulose? New Phytol. 186:385–391. doi:10.1111/j.1469-8137.2009.03176.x.
- Shavrukov Y, Kurishbayev A, Jatayev S, Shvidchenko V, Zotova L, Koekemoer F, de Groot S, Soole K, Langridge P. 2017. Early flowering as a drought escape mechanism in plants: how can it aid wheat production? Front Plant Sci. 8:1–8. doi:10.3389/fpls.2017.01950.
- Shi Y, Zhang Y, Han W, Feng R, Hu Y, Guo J, Gong H. 2016. Silicon enhances water stress tolerance by improving root hydraulic conductance in Solanum lycopersicum L. Front Plant Sci. 7. doi:10.3389/fpls.2016.00196
- Siddiqui MH, Al-Whaibi MH. 2014. Role of nano-SiO2 in germination of tomato (Lycopersicum esculentum Mill.) seeds. Saudi J Biol Sci. 21:13–17. doi:10.1016/j.sjbs.2013.04.005.
- Soltani M, Kafi M, Nezami A, Taghiyari HR. 2018. Effects of silicon at nano and micro scales on the growth and nutrient uptake of potato minitubers (Solanum tuberosum var. Agria) in greenhouse conditions. BioNanoSci. 8:218–228. doi:10.1007/s12668-017-0467-2.
- Souri Z, Khanna K, Karimi N, Ahmad P. 2020. Silicon and plants: current knowledge and future prospects. J Plant Growth Regul. 40:906–925. doi:10.1007/s00344-020-10172-7.
- Souza EJ, Martin JM, Guttieri MJ, O'Brien KM, Habernicht DK, Lanning SP, McLean R, Carlson GR, Talbert LE. 2004. Influence of genotype, environment, and nitrogen management on spring wheat quality. Crop Sci. 44:425–432. doi:10.2135/cropsci2004.4250.
- Stewart ZP, Pierzynski GM, Middendorf BJ, Prasad PVV. 2020. Approaches to improve soil fertility in sub-Saharan Africa. J Exp Bot. 71:632–641. doi:10.1093/jxb/erz446.
- Svoboda P, Kurešova G, Raimanová I, Kunzová E, Haberle J. 2020. The effect of different fertilization treatments on wheat root depth and length density distribution in a long-term experiment. Agronomy. 10:1355. doi:10.3390/agronomy10091355.
- Swain R, Rout GR. 2020. Silicon mediated alleviation of salinity stress regulated by silicon transporter genes (Lsi1 and Lsi2) in Indica rice. Braz Arch Biol Technol. 63. doi:10.1590/1678-4324-2020180513
- Takahashi E, Ma JF, Miyake Y. 1990. The possibility of silicon as an essential element for higher plants. Comm Agric Food Chem. 2(2):99–102.
- Tian J, Deng Z, Zhang K, Yu H, Jiang X, Li C. 2015. Genetic analyses of wheat and molecular marker-assisted breeding: genetic map and QTL mapping. Beijing: Springer. doi:10.1007/978-94-017-7390-4
- Toledo MZ, Castro GSA, Crusciol CAC, Soratto RP, Cavariani C, Ishizuka MS, Picoli LB. 2012. Silicon leaf application and physiological quality of white oat and wheat seeds. Ciênc Agrár Lond. 33:1693–1702.
- Trethowan RM, van Ginkel M, Rajaram S. 2002. Progress in breeding wheat for yield and adaptation in global drought affected environments. Crop Sci. 42:1441–1446.
- Rehman MZU, Rizwan M, Rauf A, Ayub MA, Ali S, Qayyum MF, Waris AA, Naeem A, Sanaullah M. 2019. Split application of silicon in cadmium (Cd) spiked alkaline soil plays a vital role in decreasing Cd accumulation in rice (Oryza sativa L.) grains. Chemosphere. 226:454–462.
- Vandeleur R, Niemietz C, Tillbrook J, Tyerman SD. 2005. Role of aquaporins in root responses to irrigation: root physiology – from gene to function. Plant Soil. 274:141–161. doi:10.007/s11104-004-8070-z.
- Vatanserver R, Ozyigit II, Filiz E, Gozukara N. 2017. Genome-wide exploration of silicon (Si) transporter genes, Lsi1 and Lsi2 in plants; insights into Si-accumulation status/capacity of plants. Biometals. 30:185–200. doi:10.1007/s10534-017-9992-2.
- White CA, Sylvester-Bradley R, Berry PM. 2015. Root length densities of UK wheat and oilseed rape crops with implications for water capture and yield. J Exp Bot. 66:2293–2303. doi:10.1093/jxb/erv077.
- Worku T, Khare D, Tripathi SK. 2018. Spatiotemporal trend analysis of rainfall and temperature and its implication on crop production. J Water Clim Change. 10:799–817. doi:10.2166/wcc.2018.064.
- Xu C, Wang M, Zhou L, Quan T, Xia G. 2013. Heterologous expression of the wheat aquaporin gene TaTIP2;2. Compromises the abiotic stress tolerance of Arabidopsis thaliana. PLoS One. 8:e79618. doi:10.1371/journal.pone.0079618.
- Yamaji N, Chiba Y, Mitani-Ueno N, Ma JF. 2012. Functional characterization of a silicon transporter gene implicated in silicon distribution in barley. Plant Physiol. 160:1491–1497.
- Yan G-C, Miroslav N, Ye M-J, Xiao Z-X, Liang Y-C. 2018. Silicon acquisition and accumulation in plant and its significance for agriculture. J Integr Agric. 17:2138–2150. doi:10.1016/S2095-3119(18)62037-4.
- Younis AA, Khattab H, Emam MM. 2020. Impacts of silicon and silicon nanoparticles on leaf ultrastructure and TaPIP1 and TaNIP2 gene expressions in heat stressed wheat seedlings. Biol Plant. 64:343–352. doi:10.32615/bp.2020.030.
- Zargar SM, Mahajan R, Bhat JA, Nazir M, Deshmukh R. 2019. Role of silicon in plant stress tolerance: opportunities to achieve a sustainable cropping system. 3 Biotech. 9:73. doi:10.1007/s13205-019-1613-z.