Abstract
The glycemic carbohydrates we consume are currently viewed in an unfavorable light in both the consumer and medical research worlds. In significant part, these carbohydrates, mainly starch and sucrose, are looked upon negatively due to their rapid and abrupt glucose delivery to the body which causes a high glycemic response. However, dietary carbohydrates which are digested and release glucose in a slow manner are recognized as providing health benefits. Slow digestion of glycemic carbohydrates can be caused by several factors, including food matrix effect which impedes α-amylase access to substrate, or partial inhibition by plant secondary metabolites such as phenolic compounds. Differences in digestion rate of these carbohydrates may also be due to their specific structures (e.g. variations in degree of branching and/or glycosidic linkages present). In recent years, much has been learned about the synthesis and digestion kinetics of novel α-glucans (i.e. small oligosaccharides or larger polysaccharides based on glucose units linked in different positions by α-bonds). It is the synthesis and digestion of such structures that is the subject of this review.
Hyperglycemia and health
There is abundant evidence that postprandial hyperglycemia is an indicator for risk of coronary heart disease (CHD), stroke, and overall mortality (Almdal et al. Citation2004 Hanefeld et al. Citation1996); rapid rise in blood glucose levels increases low-level inflammatory effects, oxidative stress, and harmful effects on beta cells which decreases insulin sensitivity () (Ceriello et al. Citation2004; Wallander et al. Citation2005). Hyperglycemia lasting more than 2 hours postprandial has been shown to be a better predictor of CHD, stroke, and overall mortality than hemoglobin A1c levels (Jackson, Yudkin, and Forrest Citation1992; Meigs et al. Citation2002). Many have hypothesized that postprandial hyperglycemia contributes to diabetes complications through damaging the vasculature tissue, such as retinopathy and nephropathy, which is likely why the cardiovascular disease incidence is so highly correlated to poorly controlled diabetes (Ceriello et al. Citation2004; Meigs et al. Citation2002). Even in non-diabetics, a meal high in rapidly digestible starches allows for some postprandial and post-challenge hyperglycemia that may contribute to cardiovascular damage (Hanefeld and Schaper Citation2007). Poor insulin secretion and decreased insulin sensitivity are key characterizations of type 2 diabetes, as well as progressive beta cell dysfunction (Wallander et al. Citation2005).
In addition to vascular damage caused by acute postprandial hyperglycemia, there may be an increase in oxidative stress when excessive blood glucose fluctuations occur pre- and postprandially, with quick absorption of glucose from meals (Monnier et al. Citation2006). Furthermore, activation of protein kinase C, MAP-kinase, and NF-kB are promoted with intracellular hyperglycemia, increasing reactive oxygen species (Brownlee Citation2001; Monnier et al. Citation2006). It has been reported that “overproduction of superoxide by the mitochondrial electron-transport chain” is the common factor linking the increased cell abnormalities in diabetes (Brownlee Citation2001; Campos Citation2012; Du et al. Citation2000; Nishikawa et al. Citation2000).
Value of slowly digestible carbohydrates to health
Inhibition of the enzymes that digest starch, such that glucose release into the bloodstream is slowed, is one treatment method for type 2 diabetes. Currently, acarbose is used as a competitive inhibitor affecting the activities of enzymes in the human digestive tract, i.e. pancreatic α-amylase and various α-glucosidases (Hanefeld and Schaper Citation2007; Martin and Montgomery Citation1996). While enzyme inhibition is one method clinically used to slow the glucose release of carbohydrate digestion, as mentioned, this review will examine the potential to modulate glycemic response through the use of α-glucans with structures and glycosidic linkages that do not allow for immediate hydrolysis.
In addition to the effect of modulating glycemic response, slowly digestible carbohydrates which are digested into the ileal (distal) region of the small intestine may stimulate endocrine L-cells to reduce appetite and slow gastric emptying. This has recently been demonstrated in rats using fabricated slowly digestible carbohydrate microspheres that were shown to digest into the ileum. Long-term feeding (11 weeks) of the microspheres to diet-induced obese rats produced lower daily food intake and reduced gene expression of the hypothalamic appetite-stimulating neuropeptides (Hasek et al. Citation2017).
Glycemic carbohydrates and their structure
Oligo- and polysaccharides are built of multiple sugar molecules, or monosaccharides, joined by glycosidic linkages occurring between the hydroxyl group of one monosaccharide with an anomeric carbon of another. The human digestive tract, as well as mammals in general, contains carbohydrate-digesting enzymes consisting of α-amylases secreted in salivary and pancreatic fluids that digest starch and starch products to linear di-, tri- and tetra-oligosaccharides (maltose, maltotriose, maltotetraose) and branched α-limit dextrins, mixtures of D-glucopyranose units linked by (α1→4) or (α1→6) glycosidic bonds. Then the small intestine mucosal α-glucosidases [maltase-glucoamylase (MGAM) and sucrase-isomaltase (SI)] that hydrolyze these and other digestible glycans to monosaccharides prior to their absorption (Zhang and Hamaker Citation2009). The human body absorbs and metabolizes glucose, fructose, and galactose. The common glycosidic bonds that are digestible by humans include (α1→4) and (α1→6) glucose-glucose that make up (iso)maltose, (iso)maltooligosaccharides, and starch; sucrose, (α1→2) glucose-fructose; and lactose, (β1→4) galactose-glucose. Uncommon linkages that can be hydrolyzed by the α-glucosidases include (α1→1), (α1→2), and (α1→3) glucose-glucose; and (α1→3), (α1→4), (α1→5), and (α1→6) glucose-fructose (Lee et al. Citation2016). When multiple monosaccharides are linked together, they form polysaccharides, which are used for energy storage and structure. For example, glycogen is a storage polysaccharide within the human body, consisting of (α1→4)-linked glucose molecules that are relatively highly branched through (α1→6) linkages. Starch, which is the glucose storage form in plants and the most common dietary polysaccharide, consists of two types of polymers: Amylose and amylopectin. Amylose, is principally a linear (α1→4)-linked glucan, whereas in amylopectin the (α1→4) linear chains are connected via (α1→6) branching points. β-Glucans (polymers of glucose with β-glycosidic linkages), such as cellulose [(β1→4)-linked] and β-glucan [in cereals, (β1→4)- and (β1→3)-linked], is another commonly consumed polysaccharide; humans lack cellulase enzymes to digest these β-bonds.
The enzymes responsible for mammalian α-glucan digestion
To digest the dietary available carbohydrates to the monosaccharides glucose, fructose, and galactose, the mammalian body employs the salivary and pancreatic α-amylases (EC 3.2.1.1.) and the small intestine mucosal two-enzyme complexes of maltase-glucoamylase (MGAM) (EC 3.2.1.20 and 3.2.1.3) and sucrose-isomaltase (SI) (EC 3.2.148 and 3.2.10). The α-amylases are classified in glycoside hydrolase (GH) family GH13, and the four catalytic subunits of MGAM and SI in GH31 (Nichols et al. Citation2003). The four enzyme subunits of these α-glucosidases have different roles in the conversion of glycemic carbohydrates to glucose (and of sucrose to glucose and fructose). α-Glucans with structures and linkages that are less easily hydrolyzed by these enzymes potentially are of interest as slowly digestible carbohydrates. Following digestion of starch by salivary and pancreatic α-amylase to linear maltooligosaccharides and branched α-limit dextrins, in the small intestine the α-linked glucans and sucrose are hydrolyzed by the mucosal α-glucosidases, MGAM and SI, into free glucose (Zhang and Hamaker Citation2009). Each α-glucosidase has a unique role, and understanding their roles can aid in designing carbohydrates with low digestibility carbohydrates. The α-glucosidase dimers are composed of N- and C-terminal subunits; for MGAM the N- and C-terminal subunits are maltase and glucoamylase, and for SI these are isomaltase and sucrase, respectively (Quezada-Calvillo et al. Citation2008). In the literature, they are also termed N- and C-terminal MGAM and SI. The N-terminal domains of both α-glucosidase complexes are connected to the enterocyte membrane, with the C-terminal subunits linked and facing the internal cavity of the intestine. The domains are anchored by an O-glycosylated stalk that branches out of the N-terminal domain (Lee et al. Citation2014; Sim et al. Citation2010) ().
Glucoamylase digests (α1→4) glucosidic bonds and acts faster on longer chain α-glucans than maltase does, and even can digest native dispersed starch (Lin et al. Citation2012). The ability of the mammalian α-glucosidases to hydrolyze different α-linked glucose-glucose disaccharides has been studied using recombinantly expressed and purified enzymes. Glucoamylase was shown to act (enzyme efficiency of glucose generation) on maltose (α1→4) (Kcat/Km 51.0 mM−1s−1), kojibiose (α1→2) (Kcat/Km 0.9 mM−1s−1), and nigerose (α1→3) (Kcat/Km 2.7 mM−1s−1); with higher maltase activity than the maltase subunit itself (Kcat/Km 51.0 vs. 12.7 mM−1s−1) (Lee et al. Citation2016). Glucoamylase is reported to be inhibited by maltotriose and maltotetraose at high concentrations (Quezada-Calvillo et al. Citation2008).
In addition for digesting maltose, kojibiose, and nigerose, maltase has minor activity towards isomaltose (α1→6) (Kcat/Km 0.1 mM−1s−1). Maltase does not digest larger maltooligosaccharides efficiently; under in vitro experimental conditions, full hydrolysis by maltase took place in 60 min for maltotriose, maltotetraose, and maltopentaose, while maltose was hydrolyzed in 20 min (Lee et al. Citation2014). Compared to glucoamylase, or the SI subunits, maltase has a higher hydrolyzing activity on kojibiose and nigerose ().
Table 1. Kinetic Parameters of Each Recombinant Mucosal α-Glucosidase on Differently α-Linked Disaccharides with Two Glucoses. Adapted from Lee et al. (Citation2016).
Per their common names, sucrase hydrolyzes sucrose (α1→2, glucose-fructose) and isomaltase hydrolyzes isomaltose (α1→6, glucose-glucose). However, the SI complex is notably responsible for the majority of mucosal maltase activity, because the human intestine may contain 40-50 times more SI than MGAM (Lee et al. Citation2013; Quezada-Calvillo et al. Citation2007). Recombinant mammalian α-glucosidase, sucrase and isomaltase had Kcat/Km values for the hydrolysis of maltose of 2.7 and 1.7, respectively; both enzymes showed some, though lesser, hydrolysis activity for kojibiose and nigerose compared to the MGAM subunits (Lee et al. Citation2013) (). Some sucrose hydrolyzing activity was also found for glucoamylase, and interestingly isomaltulose (α1→6, glucose-fructose) was hydrolyzed mostly by isomaltase. Both SI subunits digested longer linear maltooligosaccharides (e.g. G5 compared to G2) quite slowly compared to the MGAM subunits (Lee et al. Citation2013). Lee et al. (Lee et al. Citation2014) proposed that selective inhibition to target MGAM subunits would slow down the release of glucose to the rates of the SI subunits, as MGAM digests more efficiently maltooligosaccharides than SI.
Heymann et al. (Heymann, Breitmeier, and Gunther Citation1995), upon measuring SI kinetics, proposed that the sucrase subunit has maximal activity against maltose (α1→4)-G2, and the isomaltase subunit has high activity against (α1→6) branched oligosaccharides composed of up to 4 glucopyranose residues (Heymann, Breitmeier, and Gunther Citation1995). Lee et al. (Lee et al. Citation2014) showed though that sucrase and isomaltase have similar hydrolyzing capabilities for maltose.
It is known that (α1→6)-linked branched structures, such as the α-limit dextrins, the digested products of salivary and pancreatic α-amylases, are hydrolyzed at a much slower rate than (α1 →4)-linked linear glucans (Zhang and Hamaker Citation2009). Studies on α-amylase showed that optimal enzyme activity occurs when five or more (α1→4)-linked glucose molecules are in the substrate. (α1→6)-branched linkages of amylopectin molecules hinder α-amylase activity (Quezada-Calvillo et al. Citation2008).
The precise roles of the individual α-glucosidases are now well documented, and a synergistic picture of their concerted action seems to appear. Larger starch digestion products are effectively digested by the outer located glucoamylase enzyme and the small linear and branched maltooligosaccharide products are further digested to glucose by the inner located maltase and isomaltase (). Glucoamylase and maltase can digest unusual α1→2- and α1→3-linked glucans, though maltase has higher capacity.
Inhibition of the α-glucosidases
While carbohydrate molecular structure influences digestion rate by α-glucosidases, phytochemical inhibitory molecules that are present at the time of digestion may also influence the rate of glucose release by these enzymes. Plant phenols (e.g. chlorogenic acid, caffeic acid and gallic acid) are a family of metabolites reported to have inhibitory effects on SI and MGAM. Chlorogenic acid has been shown to have α-glucosidase inhibitory effects when consumed with maltose or sucrose; one study by Ishikawa et al. (Ishikawa et al. Citation2007) showed that, when consumed before a meal, leaves of Nerium indicum (oleander) lowered postprandial glucose levels by inhibiting α-glucosidases (Ishikawa et al. Citation2007). Coffee contains chlorogenic acid, and green tea contains caffeic acid and catechins which are known α-glucosidase inhibitors (Adisakwattana et al. Citation2009; Nguyen et al. Citation2012). In a study by Simsek et al. (Simsek et al. Citation2015), chlorogenic acid, epigallocatechin gallate, (+)-catechin, caffeic acid, and gallic acid were examined for their effect on the kinetics of maltose digestion and mechanism of inhibition against each α-glucosidase subunit. It was found that the inhibition constants (Ki) for epigallocatechin gallate against glucoamylase (1.7 ± 0.7 μM) and chlorogenic acid against sucrase (1.8 ± 0.3 μM) were the lowest compared to any other phenolics acting on any other subunits (Simsek et al. Citation2015). Therefore, there is benefit in focusing further research on the effect of epigallocatechin gallate and chlorogenic acid on slowing the α-glucosidase C-terminal subunit digestion.
Acarbose is produced by some Gram-positive bacteria and is a potent selective inhibitor of the C-terminal α-glucosidase subunits (Lee et al. Citation2012). 1-Deoxynojirimycin which is found in the mulberry plant bark and root was shown to be a potent competitive MGAM inhibitor (Breitmeier, Günther, and Heymann Citation1997), but against which subunit has yet to be determined (Breitmeier, Günther, and Heymann Citation1997; Hanefeld and Schaper Citation2007; Liu et al. Citation2015; Martin and Montgomery Citation1996). The glucoamylase C-terminal MGAM subunit acts very rapidly on smaller starch units and its inhibition could potentially be used to slow the rate of hydrolysis on longer chain maltooligosaccharides.
α-Glucans: Current and future ingredients for the food industry
Health concerns are important drivers of consumer preferences and demands on food product development are based on taste, nutritional value, and healthfulness. As mentioned, digestible carbohydrates such as starch and its derivatives (maltodextrins and maltooligosaccharides) are the predominant α-glucans in our diets, and have recently come under scrutiny for their postulated negative impact on health, particularly when consumed in excess. α-Glucan ingredients that have slowly digestible or non-digestible profiles constitute a healthful alternative to highly processed starches and its derivatives. There is a wide range of synthetic or naturally-occurring α-glucan ingredients that differ in digestibility profiles and technological properties. The α-glucans include a large group of linear, branched or cyclic oligo- and polysaccharides that are composed of glucose moieties joined via (α1→2), (α1→3), (α1→4), and (α1→6) glycosidic linkages. In this section, we describe the most prominent α-glucan ingredients with current or potential uses in the food industry.
Commercially available α-glucan ingredients
Starch
Starch is the most abundant α-glucan as it is the main storage carbohydrate in cereals, pulses and tubers. Normal starches are composed of around 20% of amylose and 80% of amylopectin, but this ratio differs depending on the starch source (van der Maarel and Leemhuis Citation2013; van der Maarel et al. Citation2002). Also, the branching pattern and the average length of the (α1→4) chains varies with the origin of the starch. Based on the rate of digestion, starch is classified into rapidly digestible starch (RDS), slowly digestible starch (SDS) and resistant starch which largely defines its nutritional quality (Englyst, Kingman, and Cummings Citation1992). In its native form, starch is characterized by a granular structure that renders it slowly digestible or resistant (Oates Citation1997; Valk et al. Citation2015). It has been shown, for example, that native cereal starches are slowly digestible due to their layered structure constituted by crystalline and amorphous regions (Zhang, Ao, and Hamaker Citation2006). As mentioned, the health benefits of SDS mainly pertain to a slower rate of glucose release that results in a reduced postprandial glycemia (Lehman and Robin Citation2007). However, as starch is cooked and gelatinized it loses its granular structure which results in a significant increase in its digestibility (Bornet et al. Citation1989). When discussing gelatinized starches that have lost their crystalline structure, their molecular features then become the main determinant factors for digestibility. In particular, the fine structure of amylopectin (e.g. average length of (α1→4) chains and branching pattern) and the ratio of amylose to amylopectin are the main determinants of gelatinized starch physical state and digestibility. Amylopectin molecules with either higher amounts of long or short chains were found to have a similar high content of SDS due to two different mechanisms. Whereas the presence of long chains leads to the formation of physical structures that decrease enzyme accessibility, a highly branched structure limits itself the accessibility of digestive enzymes (Zhang, Ao, and Hamaker Citation2008). Starches that are high in amylose content have a resistant starch character both in their native state and after gelatinization and retrogradation, the latter caused by amylose formation of long-chain double-helical crystallites that are resistant to digestion (Jiang et al. Citation2010). Consumption of resistant starch has been shown to positively influence bowel health, blood lipids profile, and to reduce the glycemic and insulinemic responses (Nugent Citation2005).
Isomaltulose
Isomaltulose is a disaccharide composed of glucose and fructose linked by an (α1→6)-glycosidic linkage (). It has about 42% of the sweetness of sucrose, and has been identified to occur naturally at low levels in honey and sugar cane extract (Siddiqui and Furgala Citation1967; Takazoe Citation1985). It is commercially produced by the enzymatic rearrangement of sucrose (glucose- (α1→2)-β-fructose) using a sucrose mutase (EC.5.4.99.11) found in bacteria e.g. Protaminobacter rubrum (Pelzer et al. Citation2012). It is commonly used as a non-cariogenic sucrose replacer. In vitro studies of the digestibility properties of isomaltulose with mammalian intestinal α-glucosidases (including human) indicate that the rate of hydrolysis of this sugar is significantly slower than that of sucrose and maltose (Tsuji et al. Citation1986). Animal studies have shown that isomaltulose is completely digested and absorbed in the small intestine, but the postprandial glycemic and insulinemic responses rise at a slow rate, with maximum concentrations of glucose and insulin being lower than for sucrose (Kawai, Okuda, and Yamashita Citation1985; van Can et al. Citation2012). This slowly digestible property makes isomaltulose a suitable ingredient for products targeted to consumers with diabetic and pre-diabetic dispositions. Furthermore, due to its complete digestion, clinical studies have shown that both healthy and diabetic subjects can tolerate doses of up to 50 g without presenting intestinal discomfort. Isomaltulose is a white crystalline substance, characterized by a similar sweetness profile as sucrose which leaves no aftertaste. It has a melting point of 123 to 124 °C, lower to that of sucrose, and is stable under acidic conditions (Irwin and Sträter Citation1991).
Table 2. α-Glucan ingredients: Chemical structures, properties and applications in food industry.
Isomaltooligosaccharides (IMOs)
IMOs are found naturally in various fermented foods such as miso, sake, or soy sauce but also in honey (Playne and Crittenden Citation2004). Commercial IMOs are produced enzymatically and are the market leader in the dietary carbohydrate sector of functional foods (Mountzouris, Gilmour, and Rastall Citation2002). They are generally obtained industrially from starch hydrolysates (maltose and maltodextrins) through the action of α-transglucosidases (EC 2.4.1.24) (Roper and Koch Citation1988; Yasuda, Takaku, and Matsumoto Citation1986), or from sucrose using dextransucrases (Paul et al. Citation1992; Remaud-Simeon et al. Citation1994). IMOs, also called glucosyl saccharides, not only containing (α1→6) linkages, but also (α1→4) linkages, and nigerooligosaccharides comprised of (α1→3) and/or kojioligosaccharides comprised of (α1→2) linkages, can be found in commercially available products (Chaen et al. Citation2001; Kobayashi et al. Citation2003; Konishi and Shindo Citation1997; Yamamoto et al. Citation2004; Yun, Lee, and Song Citation1994) ().
IMOs are considered as non-digestible carbohydrates with prebiotic properties with benefit to the human microbiome (Goffin et al. Citation2011; Rycroft et al. Citation2001). Commercial IMO ingredients are used as a source of a soluble dietary fiber, a prebiotic and/or a low-calorie sweetener. For instance, Gu et al. (Gu et al. Citation2003) observed an increase of reproduction of Bifidobacterium and Lactobacillus and an inhibition of growth of Clostridium perfringens after ingestion of IMOs in mice and humans. However, other studies have shown that IMOs are hydrolyzed at least partially by mammalian digestive enzymes before they reach the colon (Hodoniczky, Morris, and Rae Citation2012; Kohmoto et al. Citation1992; Tsunehiro et al. Citation1999). More specifically, in vitro studies using mammalian brush border intestinal enzymes showed that glucose disaccharides containing (α1→6), (α1→4), (α1→2) and (α1→3) are digested by the different small intestine mucosal α-glucosidases (Lin, Lee, and Chang Citation2016). A recent survey of commercially available IMO-based ingredients challenges current labeling as “dietary fibers”, “low glycemic” and “zero calorie”. The authors performed a two-subject blood glucose response acute study in which a commercial IMO ingredient proved as glycemic as glucose (Madsen et al. Citation2017).
Resistant maltodextrins (RMDs)
RMDs are formed when liquefied starch is heated under acidic conditions in order to rearrange the glycosidic linkages. The treatment causes an increase in the proportion of (α1→6) linkages and introduces (α1→2) and/or (α1→3)-linkages as well as linkages of β-anomeric configuration (Dermaux and Wills Citation2007) (). It is the combination of these linkages that render them poorly digestible. During heat treatment, starch undergoes pyroconversion, which involves hydrolysis, transglucosidation, and, in some cases, repolymerization of the branched oligosaccharides generated in the previous reactions. The rearrangement of glycosidic linkages that occurs during pyroconversion is what renders these dextrins resistant to digestion. They are popular ingredients in the food industry because they are soluble, have low viscosity, and have little to no impact on taste and color. Depending on their level of purification, resistant dextrins can contain up to 92% non-digestible carbohydrate content. In addition, they exhibit a high degree of thermal and pH stability (Ohkuma and Wakabayashi Citation2000). This ingredient is predominantly used to increase the dietary fiber content of food products without conferring negatively-perceived sensory attributes. RMDs are also suitable replacers of fully-caloric carbohydrates such as sucrose (4 kcal/g) since the caloric value for resistant dextrins is estimated to range between 1 and 2.5 kcal/g (Panasevich et al. Citation2015). Due to this property, this ingredient can also be used to replace fully-digestible maltodextrins in the formulation of food products of medium to low glycemic index.
Polydextrose
Polydextrose is a synthetic, soluble, and non-viscous glucan polymer that is manufactured by melt condensation of glucose and sorbitol in acidic and vacuum conditions. This manufacturing process results in a highly-branched, low molecular weight polymer (Mwn = 2000 Da; Degree of Polymerization [DP] = 12) constituted by glucose units linked by (α1→2), (α1→3), (α1→4), or (α1→6) glycosidic bonds, present in both α- and β-anomeric configuration (Rennhard Citation1973) (). Polydextrose is poorly digested in the small intestine and, thus, has reduced caloric availability. Its other applications in food include glazing agent, humectant, stabilizer and thickener (Srisuvor et al. Citation2013; Voragen Citation1998). Its complex structure also results in its poor degradation by microbes making it non-cariogenic and only a small fraction is metabolized by cecal/colonic microbiota.
Cyclodextrins (CDs)
CDs are cyclic oligosaccharides of glucose molecules linked by (α1→4)-glycosidic linkages. They are classified depending on the number of glucose units, namely, α-, β- and γ-CDs for 6, 7, and 8 glucose units, respectively (). CDs are formed enzymatically from hydrolyzed starch by cyclodextrin glycosyltransferases (CGTases; E.C.2.4.1.19), enzymes that catalyze the cleavage of (α1→4) linkages in starch and the subsequent transfer of the newly produced reducing end to a non-reducing end of the same molecule (Thiemann et al. Citation2004). Purified α-, β- and γ-CDs have been approved for their use as food additives (EFSA Citation2007; JECFA Citation1993, Citation1995, Citation1999, Citation2002, Citation2006). Different applications of CDs include flavor encapsulation, taste modification by elimination of bitter or off-flavors as well as odors, food preservation, and as cholesterol sequestrants (Astray et al. Citation2009) in a variety of food products as indicated by the Codex Alimentarius, General Standard for Food Additives (CODEX Alimentarius Commission for International Food Standards Citation2017). More specifically, the use of β-CD is limited to a few food categories (i.e., chewing gum, pre-cooked pastas and noodles, starch-based snacks, water-based, and flavored drinks) (Thiemann et al. Citation2004). α- and β-CDs are not hydrolyzed by gastrointestinal enzymes, but fermented by the colon microbiota. According to the EFSA Health Claim (EFSA Panel on Dietetic Products, Nutrition and Allergies Citation2012), α-CDs have been shown to effect a significant reduction in postprandial glycemic response at a dose of at least 5 g per 50 g of starch. The reduction in glycemic response is due to the tight helix of the α-CD, the inhibitory effect of α-CD on pancreatic amylase, and that α-CD may delay gastric emptying (Buckley et al. Citation2006; Gentilcore et al. Citation2011; Koukiekolo et al. Citation2001; Larson, Day, and McPherson Citation2010). In contrast, γ-CD is readily digested in the gastrointestinal tract yielding mainly maltose, maltotriose and glucose. Therefore, the metabolism of γ-CD closely resembles that of starch and maltodextrins. Although they are rapidly metabolized and absorbed in the small intestine, γ-CDs have also been reported to impact glycemic response with a similar inhibitory effect on pancreatic α-amylase as α-CD (Koukiekolo et al. Citation2001; Wolf, Chow, and Lai Citation2006).
Novel α-glucan ingredients
Neo-amyloseTM
Neo-amyloseTM is an α-glucan obtained through the polymerization of sucrose by the action of amylosucrase (E. C. 2.4.1.4) derived from Neisseria polysaccharea (Büttcher et al. Citation1997). It is a water insoluble, unbranched polymer composed of (α1→4) glucosidic linkages with a chain length ranging from 35 up to 100 glucose units (Peters, Rose, and Moser Citation2010). It is an ingredient classified as a type 3 resistant starch (retrograded starch formed by cooking followed by cooling) that is around 90% non-digestible which makes it a suitable ingredient for use as a dietary fiber (Bengs and Brunner Citation2000).
Cyclic cluster dextrin (CCD)
CCD is made from amylopectin through the cyclization reaction of glycogen branching enzyme (E.C 2.4.1.18) from the hyperthermophilic bacterium Aquifex aeolicus (Takata et al. Citation2003). It has relatively long chains which adopt a helical conformation enabling the formation of inclusion complexes with guest molecules such as organic acids (). CCD is typically used in baked products, beverages, powder soups made from fruit and vegetables. It has also been used as a spray-drying aid. CCDs have received an FDA GRAS status (FDA 2010; Takata et al. Citation1996) and animal studies have shown that they may accelerate gastric emptying and that they are slowly digestible (Takii et al. Citation1999).
Dextran
Luis Pasteur discovered dextran in wine and van Tieghem (Van Tiegham Citation1878) designated the dextran-producing bacterium as Leuconostoc mesenteroides. Dextrans are microbial α-glucans with 50–97% (α1→6) linkages (). They are produced from sucrose via large scale fermentation or enzymatic synthesis involving extracellular dextransucrase (E.C. 2.4.1.5) enzymes (Jeanes et al. Citation1954). Alternatively, these dextrans can be produced from starch by dextrin dextrinases (E.C. 2.4.1.2) (Yamamoto, Yoshikawa, and Okada Citation1993). Dextrans are approved as GRAS ingredients for their use in food products and feed. Also, the European Commission approves the use of dextran in baked goods at levels up to 5%; dextrans of high molecular weight are used in sourdough baking to produce good quality bread (SCO Citation2000; Maina et al. Citation2011). Furthermore, dextran was described as a thickening agent, an alternative cryostabilizer, fat replacer, or low-calorie bulking agent of interest for the food processing industry (Park and Khan Citation2009). Dextran is only partially hydrolyzed to monosaccharides by small intestine α-glucosidases. The remaining dextran that escapes small intestine digestion is fermented in the colon resulting in short chain fatty acid production. Due to its low digestibility by intestinal enzymes, this polysaccharide represents a source of dietary fiber (Dahlqvist Citation1963; Dahlqvist Citation1961; Jeanes Citation1975).
Alternan and alternan-oligosaccharides
Alternan is a α-glucan consisting of alternating (α1→6) and (α1→3) linkages with a low degree of branching (). It is produced from sucrose by an enzyme of the glucansucrase family, known as alternansucrase (EC 2.4.1.140), that is found mainly in Leuconostoc mesenteroides (Côté and Robyt Citation1982; Leemhuis, Pijning, et al. Citation2013; Park and Khan Citation2009). (Côté and Robyt Citation1982; Leemhuis, Pijning, et al. Citation2013; Park and Khan Citation2009). Alternan is resistant to most known microbial and mammalian enzymes and can only be hydrolyzed by isomaltodextranases, and alternanases. Due to its resistance to digestion it can be used for production of ingredients for functional foods such as prebiotics (Leathers, Hayman, and Côté Citation1997; Park and Khan Citation2009). It has also been reported that alternan oligosaccharides derived from alternanase activity are used as low-glycemic sweeteners. Some studies state that these oligosaccharides are potential prebiotics (Leathers, Hayman, and Côté Citation1997), however in vivo trials in humans showed that they are slowly but fully digestible by human digestive enzymes in the gastrointestinal track (2008, Grysman, Carlson, and Wolever Citation2008; Vanschoonbeek et al. Citation2009). A recent study on α-glucan oligomers containing (α1→6) and (α1→3) linkages with DP ranging from 3-12, showed similar glucose and insulin response curves as the dextrose control in humans. In the same study, (α1→2) branching points were introduced into these oligomers, resulting in increased resistance to digestion in vivo in mice. However, this observation hasn’t been confirmed in humans (Hasselwander et al. Citation2017).
Highly branched α-glucans
It has been shown that the shorter side chains make highly-branched starch less prone to α-amylase degradation resulting in a slower glucose release rate (Le et al. Citation2009; van der Maarel and Leemhuis Citation2013). Different enzymatic modification strategies have been successfully used to increase the degree of (α1→6) branching points in starch (e.g. treatment with branching enzymes). In experiments by Ao et al. (Ao, Quezada-Calvillo, et al. Citation2007; Ao, Simsek, et al. Citation2007), normal corn starch was modified through treatment with β-amylase, or β-amylase- and transglucosidase (BAMTG), or maltogenic α-amylase and transglucosidase (MAMTG). In vitro methods were used to analyze digestion rates of the derived starches by pancreatic α-amylase, pancreatin, and amyloglucosidase. Starch treated with MAMTG showed an in vitro digestion rate, measured by reducing sugars produced, after 10 (69%) and 180 min (55%) of digestion, compared to control untreated gelatinized corn starch (100%). Analysis of the slowest digesting starch from this experiment showed considerably greater proportions of low molecular weight starch molecules with reduced amylopectin B1 and B2 branch chains (short and medium linear chain lengths in amylopectin), and lower ratios of (α1→4) to (α1→6)-linkages (13% (α1→6) in BAMTG-modified starch and 23% in MAMTG-modified starch). Thus, increased (α1→6)-linkages in shorter molecular size starch molecules had a slow and extended digestion (Le et al. Citation2009; van der Maarel and Leemhuis Citation2013). In a study by Lee et al in 2013, rats gavaged with a highly branched maltodextrin, made with maltogenic α-amylase together with β-amylase, had higher and extended blood glucose levels 1 hour postprandial than did those gavaged with unmodified starch or starch modified with just branching enzyme alone (Lee et al. Citation2013).
Pullulan
Pullulan is a linear polysaccharide consisting of maltotriose units interconnected by single (α1→6) glycosidic linkages (). This alternating pattern is responsible for the slow digestibility of pullulan in humans (Wolf et al. Citation2003). It is a white soluble polymer without taste and odor (Khan, Park, and Kwon Citation2007). It is produced from starch by the fungus Aureobasidium pullulans (Bender, Lehmann, and Wallenfels Citation1959). As a food additive, it is known by the E number E1204.
Enzymatic tools for the synthesis of novel α-glucans from sucrose and starch
In view of the above there remains a need for novel α-glucans from sucrose and starch that are less or only slowly digestible in the human body. Microbial α-transglycosylase enzymes acting on starch and sucrose substrates are known to convert the high glycemic index sucrose and starch carbohydrates into α-glucans of interest for the food industry. Some relevant commercial examples are the dextran and CDs, described above. In general, these α-tranglycosylase enzymes are stable, they act on renewable cheap substrates, and display high regio- and stereo-selectivity. Other strategies for the production of α-glucans do not have these advantages. For example, chemical approaches require many tedious protection and deprotection steps of the hydroxyl groups in order to achieve regio- and stereo-selectivity, toxic catalysts and solvents. Other enzymatic strategies are based on the use of “Leloir” glycosyl transferases, which require expensive nucleotide-activated sugars (e.g. uridine diphosphate glucose) and will not be the focus of this review. According to the amino acid sequence-based CAZy classification system (http://www.cazy.org/), most of these sucrose- and starch-acting α-transglycosylases (EC 2.4.) are members of families GH13, GH31, GH57, GH70 and GH77, also covering hydrolases (i.e. amylase, α-glucosidases, E.C. 3.2.) and isomerases (i.e. isomaltulose synthase, trehalose synthase, E.C. 5.4.). Despite the fact that they differ in reaction and product specificity, members of these families employ a similar double displacement α-retaining mechanism which involves the cleavage of the α-glycosidic bond of the glucose donor substrate and the formation of a covalent enzyme-glycosyl intermediate. In a second step, the enzyme-glycosyl intermediate reacts with an acceptor substrate, which can be a water molecule or an acceptor carbohydrate, yielding substrate hydrolysis or a new transglycosylation α-glucan product, respectively (). In α-glycosidases or hydrolases (EC 3.2.), water usually acts as acceptor, whereas in α-transglycosylases the binding of an acceptor carbohydrate is favored; consequently, these latter enzymes naturally display very efficient transglycosylation activities (Light et al. Citation2017). Although α-glycosidases/hydrolases catalyze the hydrolysis of α-glucans in vivo, many of these enzymes display a certain degree of transglycosylation activity that can be used for synthesis of glycosidic bonds in vitro. For example, the transglycosylation activity of α-glucosidases (EC 3.2.1.20), neopullulanases (EC3.2.1.135) and maltogenic amylases (EC 3.2.1.133) has been used for the production of isomaltooligosaccharides and branched oligosaccharides (Goffin et al. Citation2011; Lee et al. Citation1995; Niu et al. Citation2017; Yoo et al. Citation1995). Many sucrose- and starch-acting enzymes have been characterized as natural α-transglycosylases with different specificities (http://www.cazy.org/). Interestingly, protein structure studies have provided important insights in the molecular basis for product specificity of several α-transglycosylases. This also has resulted in valuable clues for subsequent steps aiming to develop tailor-made enzyme variants capable of synthesizing any desirable α-glucan structures. Most of these sucrose- and starch-acting enzymes belong to the GH13, GH70 and GH77 families that constitute the GH-H clan. Members of the GH-H clan are evolutionarily related, displaying similar protein structures and activity mechanisms. GH13, GH70 and GH77 enzymes share a catalytic (β/α)8 barrel domain and have four conserved amino acid sequence motifs containing the three catalytic Asp, Glu and Asp residues and some of the substrate binding residues (Leemhuis, Pijning, et al. Citation2013; Meng, Gangoiti, Pijning, and Dijkhuizen Citation2015) (). GH31 enzymes differ in their catalytic residues, but they adopt a similar (β/α)8 fold and were found to display a remote evolutionary relatedness with GH-H clan members (Janecek, Svensson, and MacGregor Citation2007). GH57 family enzymes, however, have a catalytic (β/α)7 barrel domain and possess their own five conserved motifs and catalytic machinery (Suzuki and Suzuki Citation2016). The main types of natural α-transglycosylases used for the production of slowly digestible or less digestible α-glucans will be illustrated in the following sections. Particular attention will be given to the variety of existing sucrose- and starch-acting GH70 enzymes, reviewing the surge of new enzymes that have been characterized in recent years. Due to their broad product specificity, GH70 enzymes represent attractive enzymatic tools for the synthesis of tailor-made α-glucans with a defined glycemic response.
Figure 4. Topology diagram models of family GH70 Glucansucrases (GS) with a circularly permutated (β/α)8 barrel (a) and the family GH13 α-amylase (β/α)8 barrel (b). Cylinders represent α-helices and arrows represent β-strands. The equivalent α-helices and β-strands in GH70 GSs and GH13 α-amylases are numbered the same. The different domains in GH70 and GH13 enzymes are indicated. Domain C of GH70 GSs is inserted between α-helix 8 and β-strand 1 while that of GH13 family α-amylase locates C-terminally of the (β/α)8 barrel. Domain B of GH13 α-amylases is inserted between β-strand 3 and α-helix 3 while that of GH70 GS is formed by two discontinuous polypeptide segments from both the N- and C-termini. The same is true for domains IV and V of GH70 GS. A variable region (VR) is present in the N-terminus of GH70 GSs. The four conserved sequence motifs (I–IV) which are located in β-strands 3, 4, 5, and 7, respectively, and are shared between family GH70 GS and GH13 enzymes, are indicated within the β-strand. The structure of the catalytic domain in the GH70 GS representative GTF180-ΔN (c, PDB: 3KLK) of L. reuteri 180 and in the GH13 representative α-amylase of Bacillus licheniformis (d, PDB: 1BPL). The (β/α)8 barrel is colored for a better representation. α-Helices and β-strands are numbered, and the conserved sequence motifs (I–IV) are indicated at the corresponding β-strand. The circularly permutated (β/α)8 barrel of GH70 GS is formed by two separate polypeptide segments (N-terminal parts colored in deep blue and C-terminal parts colored in cyan), which is caused by the insertion of domain C.

Cyclodextrin glucanotransferases
Cyclodextrin glucanotransferases (CGTase; EC 2.4.1.19) mainly convert starch into cyclic (α1→4)-linked oligosaccharides, named cyclodextrins (CDs). Depending on their main CD product, as discussed above, these enzymes are classified as α-, β-, and γ-CGTases, with 6, 7 and 8 glucose units, respectively. Apart from their main cyclization activity, CGTases also catalyze hydrolysis and intermolecular transglycosylation reactions using linear (α1→4) glucans (disproportionation reaction) or their own CD products (coupling reaction) as substrates, yielding linear products (van der Veen et al. Citation2000). CGTases are found in Bacteria and Archaea, and based on their sequences they are placed in the GH13 family, which is the largest family of glycoside hydrolases acting on starch and related α-glucans. Several three-dimensional structures of CGTases from bacteria of different genera (e.g. Bacillus, Thermoanaerobacterium and Geobacillus) are currently available without or complexed with several substrates (Han et al. Citation2014; Leemhuis, Kelly, and Dijkhuizen Citation2010). CGTase enzymes consist of 5 domains: A, B, C, D and E. Domains A, B and C are present in most GH13 family members (). Domain A comprises the catalytic (β/α)8 barrel, and together with domain B forms the substrate binding groove. Domains C and E participate in starch binding, whereas the role of domain D has remained unclear. The reaction mechanisms and substrate-binding subsites of CGTases have been analyzed in detail allowing identification of amino acid residues determining CD size specificity (Uitdehaag et al. Citation1999; Uitdehaag et al. Citation2000; van der Veen et al. Citation2000). Most CGTases synthesize a mixture of CD of different sizes, and thus, a selective purification step is required to obtain a single type of CD only (Leemhuis, Kelly, and Dijkhuizen Citation2010). Using protein engineering approaches many CGTase variants with an improved α-, β-, and γ-CD selectivity have been obtained (Han et al. Citation2014; Leemhuis, Kelly, and Dijkhuizen Citation2010).
4-α-Glucanotransferases
4-α-Glucanotransferases (4-α-GTase; EC 2.4.1.25) also designated as amylomaltases, or D-enzymes, display disproportionating activity (see below) on starch-like substrates containing consecutive (α1→4) glycosidic linkages (e.g. amylose, amylopectin, maltodextrins and glycogen). These enzymes are distributed in plants and microorganisms, where they participate in starch biosynthesis and glycogen metabolism, respectively (Boos and Shuman Citation1998; Colleoni et al. Citation1999; Wattebled et al. Citation2003). Enzymes with 4-α-GTase activity are distributed in the GH13, GH57 and GH77 families, only the GH77 family contains exclusively 4-α-GTases. Three-dimensional structures in free form or in complex with substrates are available for several GH77 family enzymes, including industrially important thermostable and thermoactive enzymes from Thermus thermophilus HB8 (PDB codes 1FP8 and 1FP9) (Kaper et al. Citation2007), Thermus aquaticus ATCC 33923 (PDB code 1CWY (Przylas et al. Citation2000)); and Aquifex aeolicus VF5 (PDB code 1TZ7; Uitdehaag et al., unpublished work). The main structural feature that distinguish GH77 enzymes from the evolutionary related GH13 proteins is the lack of domain C. Crystal structures of 4-α-GTases belonging to the GH13 and GH57 protein families have also been solved from thermophilic organisms [e.g. the archaeon Thermococcus litoralis GH57 4-α-GTase (PDB codes 1K1W, 1K1X and 1K1Y (Imamura et al. Citation2003); and the bacterium Thermotoga maritima MSB8 GH13 4-α-GTase (PDB codes 1LWH, 1LWJ, 1GJU and 1GJW) (Roujeinikova et al. Citation2001; Roujeinikova et al. Citation2002). 4-α-GTases preferably catalyze the cleavage of an (α1→4)-glucan from the non-reducing end of a donor substrate and its transfer to the non-reducing end of an acceptor (α1→4)-α-glucan via the formation of a new (α1→4) glycosidic linkage. Amylomaltase activity on starch results in the formation of a starch derivative free of amylose (consumed as donor substrate) and consisting of an amylopectin product with a mixture of both shortened and elongated side-chains (Van der Maarel et al. Citation2005). This product (e.g. Etenia) can be used as a replacement of gelatin, which is an animal-derived product widely used as a hydrocolloid in food. The presence of these relatively long chains, and its relatively high molecular weight, provide this polymer with thermoreversible gelling properties (Hansen et al. Citation2008). 4-α-GTase treated corn starch is rather resistant to digestion (Jiang et al. Citation2014). Some 4-α-GTases also catalyze intramolecular transglycosylation reactions resulting in the formation of large cyclic (α1→4)-glucans, designated as cycloamyloses (Terada et al. Citation1999). Structurally, cycloamyloses resemble cyclodextrins, however, they have a higher degree of polymerization (from 16 to several hundreds of glucose units). In view of their large ring size, cycloamyloses are not expected to display the slowly digestible properties described for α- and β-cyclodextrins, however, they may have many other applications in the pharmaceutical and biotech industry due to their capacity to encapsulate hydrophobic guest molecules within their hydrophobic cavity (Roth et al. Citation2017). Interestingly, the 4-α-GTase disproportionation activity, in combination with maltogenic amylase and pullulanase, has been used for the synthesis of starch-derived IMOs and a resistant starch-like product, respectively (Lee et al. Citation2002; Norman et al. Citation2007).
Branching Enzymes
Branching enzymes (BE, EC 2.4.1.18) act on (α1→4) glucans, cleaving an internal (α1→4) linkage and transferring the cleaved-off part to the same or another (α1→4) glucan chain via an (α1→6) branch point (Roussel et al. Citation2013; Shinohara et al. Citation2001). BE are widespread in nature and can be found in bacterial, archaeal, and eukaryotic species, where they catalyze the formation of (α1→6)-branching points during glycogen or starch biosynthesis (Suzuki and Suzuki Citation2016). BE are therefore critical determinants of the structure and properties of these intracellular storage polysaccharides. BE have gained substantial interest for the production of different starch-derived products such as highly-branched α-glucans (Kittisuban et al. Citation2014; Lee et al. Citation2013), synthetic glycogen (Kajiura et al. Citation2006), and CCD (Takata et al. Citation2003). In particular, thermostable glycogen BE enzymes from Aquifex aeolicus and Rhodothermus obamensis are the most commercially exploited (Van der Maarel and Leemhuis Citation2013). BE are placed in the GH13 and GH57 family proteins, which differ in their overall 3-dimensional structures, catalytic residues and geometry around the active site (Suzuki and Suzuki Citation2016). Numerous BE have been characterized showing differences in their substrate specificity and generating products with varied frequency and branch chain length. Whereas, some BE preferentially convert amylose (Binderup, Mikkelsen, and Preiss Citation2000; Hayashi et al. Citation2015; Palomo et al. Citation2011; Roussel et al. Citation2013), others display higher activity on amylopectin substrates (Jo et al. Citation2015; Palomo et al. Citation2009). Also, the minimal length of the donor glucan, the length of the transferred glucan, and the distance between two successive branch points differ from one BE to another (Hayashi et al. Citation2015; Jo et al. Citation2015; Palomo et al. Citation2009; Roussel et al. Citation2013; Sawada et al. Citation2014; Takata et al. Citation2003). Several experimental approaches including site-directed mutagenesis (Hayashi et al. Citation2017; Liu et al. Citation2017), domain-swapping and truncation experiments (Jo et al. Citation2015; Palomo et al. Citation2009; Welkie, Lee, and Sherman Citation2015) and X-ray crystallography studies (Abad et al. Citation2002; Feng et al. Citation2015; Hayashi et al. Citation2015; Na et al. Citation2017; Pal et al. Citation2010; Palomo et al. Citation2011) have been used to understand the mechanism of branching activity. Very recently the Cyanothece sp. ATCC 51142 BE1 structure with an oligosaccharide bound in the active site cleft has been solved providing a better understanding of the reaction catalyzed by these enzymes (Hayashi et al. Citation2017). However, the relationship between the reaction specificity (e.g. preferred chain lengths transferred) and the BE protein structure remains unclear, and it is possible that variation exists in the mode of oligosaccharide binding depending on the BE species (Hayashi et al. Citation2017). This information may allow a better control of the degree of branching and the average length of branches of the reaction product by the engineering of BE with altered specificity.
Amylosucrases
Amylosucrases (AS, EC 2.4.1.4) catalyze the synthesis of linear (α1→4) glucans from sucrose, and have been characterized from various bacterial sources (see below). Although most sucrose-active α-transglycosylases belong to the GH70 family (Monsan, Remaud, and Andre Citation2010), in view of its amino acid sequence AS have been classified in the GH13 family, mostly containing starch-acting enzymes. AS activity with sucrose results in synthesis of an amylose-like polymer with a molecular weight that is significantly lower (DP up to 8) (Potocki-Veronese et al. Citation2005) than the α-glucan polysaccharides synthesized by sucrose-utilizing GH70 members (Moulis, Andre, and Remaud-Simeon Citation2016). This (α1→4) glucan is insoluble and shows a high degree of B-type crystallinity, which makes this product resistant to hydrolysis by digestive enzymes (Norman et al. Citation2007). In addition to the amylose-like polymer, sucrose isomers are usually also formed by AS, which reduce the α-glucan yield. It has been proposed that AS in vivo are involved in energy storage through glycogen elongation (Albenne et al. Citation2004). In in vitro studies, when glycogen and other polymers containing (α1→4) or (α1→4) and (α1→6) linkages are used as acceptor substrates, AS elongate some of their external chains yielding polymers with higher slowly digestible and resistant starch content (Kim et al. Citation2014; Rolland-Sabaté et al. Citation2004; Shin et al. Citation2010). With the aim of designing α-glucans with defined structures using only sucrose as raw material, the Neisseria polysaccharea AS has been combined with other α-transglycosylases, including the BE from Rhodothermus obamensis and the CGTase from Bacillus macerans, resulting in the production of glycogen and cyclodextrins, respectively (Grimaud et al. Citation2013; Koh et al. Citation2016). AS have been identified in various bacterial species (e.g. Deinococcus, Arthrobacter, Alteromonas, Methylobacillus, Synecochococcus); the AS from N. polysaccharea has been studied in most detail (Moulis, Andre, and Remaud-Simeon Citation2016). Crystal structures of AS in free form, and in complex with different substrates, have been solved (Guérin et al. Citation2012; Jensen et al. Citation2004; Mirza et al. Citation2001; Skov et al. Citation2013). In addition to the 3 domains A, B and C common to all GH13 family members (), AS have 2 extra domains named N and B’ that are unique to these enzymes. These 3D structures have allowed the identification of key residues involved in the polymerization process, and have guided the construction of AS mutants which produce in a controlled way either short maltooligosaccharides or insoluble amylose from sucrose (Albenne et al. Citation2004; Cambon et al. Citation2014; Schneider et al. Citation2009).
Dextran dextrinases
Some strains of Gluconobacter oxydans are known to produce an intracellular enzyme named dextran dextrinase (DDase, EC 2.4.1.2) catalyzing the synthesis of dextran using maltodextrins as substrate. DDase is able to transfer the non-reducing terminal glucosyl residues of maltodextrins to an acceptor substrate forming consecutive (α1→6) linkages (Naessens et al. Citation2005; Sadahiro et al. Citation2015). As a result of this activity a dextran polymer is produced, which contains some (α1→4) branches and (α1→4) linkages in (α1→6) glucosyl linear chains (Yamamoto, Yoshikawa, and Okada Citation1993). DDase also disproportionates (α1→6) glucans indicating that it is also active on its own products. The gene encoding the DDase from G. oxydans ATCC 11894 has been recently identified revealing that the protein encoded belongs to the GH15 family. With the exception of the DDase from G. oxydans ATCC 11894, all characterized GH15 family members employ an inverting mechanism yielding β-anomeric products (Pedro M. Coutinho, "Glycoside Hydrolase Family 15" in CAZypedia, available at URL http://www.cazypedia.org/, accessed 19 May 2017). In view of its α-retaining mechanism, the DDase enzyme is an atypical member of the GH15 family.
Generation of structurally diverse α-glucans by novel GH70 α-transglycosylases
The GH70 family was established for glucansucrases (GS), exclusively found in lactic acid bacteria (LAB), which convert sucrose into high molecular mass α-glucan polymers (Leemhuis, Pijning, et al. Citation2013; Monchois, Willemot, and Monsan Citation1999). GS catalyze polymer synthesis by the successive transfer of single glucosyl units from sucrose to the non-reducing end of a growing glucan chain. Alternatively, in the presence of a low-molecular mass acceptor substrate such as maltose, GS switch from polysaccharide to low molecular mass oligosaccharide synthesis, using sucrose as donor substrate. The various characterized GS synthesize a large diversity of α-glucan products from sucrose, and with all possible linkages (α1→2), (α1→3), (α1→4), and (α1→6). Also, these α-glucans may be linear or branched and may differ in their type and degree of branching, size, and conformation, resulting in oligo- and poly-saccharides with different physicochemical properties (e.g. viscosity, adhesiveness, solubility, etc.). The α-glucans synthesized by GS are classified into four categories based on their dominant glycosidic linkage type: dextran with a majority of (α1→6) linkages, mutan with a majority of (α1→3) linkages, reuteran with a majority of (α1→4), and alternan with alternating (α1→3) and (α1→6) linkages (). Three-dimensional structures are available for 5 GS proteins with different product specificity (Brison et al. Citation2012; Ito et al. Citation2011; Pijning et al. Citation2012; Vujicic-Zagar et al. Citation2010). These crystal structures show that in GS the catalytic (β/α)8-barrel domain is circularly permuted compared to GH13 and GH77 enzymes, confirming earlier predictions (MacGregor, Jespersen, and Svensson Citation1996). Interestingly, GS display a unique U-fold organized in five structural domains (A, B, C, IV and V) ( and ). The domains A, B and C form the catalytic core, and are also found in GH13 enzymes, whereas the remote domains IV and V are only present in GH70 enzymes. Thus far, 60 GS enzymes have been characterized (www.cazy.org, 21st September 2017), most of them producing dextran polymers. The industrially most relevant GS is the Leuconostoc mesenteroides NRR B-512F DSR-S dextransucrase converting sucrose into a polymer with 95% (α1→6) linkages in the main chains and 5% (α1→3) branch linkages (Monchois et al. Citation1997; Passerini et al. Citation2015). In addition, GH70 enzymes displaying dextran branching specificity have been identified providing novel tools for the production of dextrans with a controlled degree of (α1→2) and (α1→3) branching points (Brison et al. Citation2010, Citation2012; Vuillemin et al. Citation2016). First, L. mesenteroides NRRL B-1299 was found to encode two GH70 enzymes (named DSRE and BRS-A) specialized in (α1→2) transglycosylation from sucrose to a dextran acceptor substrate (Brison et al. Citation2012; Passerini et al. Citation2015). The gluco-oligosaccharides containing (α1→2) linkages synthesized by L. mesenteroides NRRL B-1299 DSRE were found to be highly resistant to the action of digestive enzymes in both humans and animals (Valette et al. Citation1993). Recently, genome analysis of Leuconostoc citreum NRRL B-742 allowed the identification of the first (α1→3) branching sucrase (named BRS-B) responsible for the high content of (α1→3) branching linkages present in the dextran produced by this strain (Vuillemin et al. Citation2016).
Figure 5. Domain arrangement of sucrose- and starch-converting GH70 enzymes.
Crystal structures of the L. reuteri 121 GtfB 4,6-α-GTase (middle), and the L. reuteri 180 Gtf180 GS (right). Domains A, B, C, IV and V are highlighted in blue, green, magenta, yellow and red, respectively. Ig2-like domains are colored in grey. As apparent from the order of the conserved regions (indicated by grey rectangles), the catalytic barrel of the GH70 glucansucrases and GH70 GtfB-like enzymes is circularly permuted (order = II-III-IV-I).
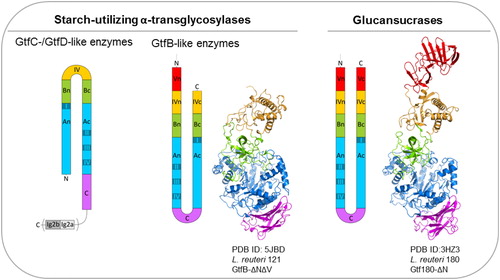
Table 3. Examples of products synthesized by sucrose- and starch-active GH70 enzymes.
In recent years, novel GH70 enzymes inactive on sucrose, but displaying clear disproportionating activity on starch/maltodextrin substrates, have been identified () (Gangoiti et al. 2015; Gangoiti, Pijning, and Dijkhuizen Citation2016; Gangoiti, Lamothe, et al. Citation2017; Gangoiti, van Leeuwen, Gerwig, et al. Citation2017; Gangoiti, van Leeuwen, Meng, et al. Citation2017; Kralj et al. Citation2011; Leemhuis, Dijkman, et al. Citation2013). Regarding their substrate specificity, these starch-converting GH70 enzymes resemble GH13 family enzymes also acting on starch-like substrates, however, they share higher amino acid sequence similarity with GS from the GH70 family. Based on their sequences, these enzymes were classified into 3 new GH70 subfamilies designated as GtfB, GtfC and GtfD, which differ in their microbial origin, product specificity and domain organization (Gangoiti et al. 2015; Gangoiti, Pijning, and Dijkhuizen Citation2016; Gangoiti, van Leeuwen, Gerwig, et al. Citation2017; Kralj et al. Citation2011), Whereas GtfB enzymes are mainly found in Lactobacillus strains and display a GS-like fold with a circularly permuted catalytic (β/α)8-barrel, GtfC and GtfD enzymes are present in non-LAB and possess a non-permuted domain organization resembling that of GH13-like enzymes () (Gangoiti et al. 2015; Gangoiti, Pijning, and Dijkhuizen Citation2016; Gangoiti, Lamothe, et al. Citation2017). In view of their intermediate position between GH13 α-amylases and GH70 GS, the emergence of GtfB, GtfC and GtfD type of enzymes have provided clues about the evolutionary history of GH70 family proteins. It has been proposed that evolution from an ancestor α-amylase to present-day GS occurred via these GtfB-, GtfC-, and GtfD-like intermediates (Gangoiti et al. 2015; Gangoiti, Pijning, and Dijkhuizen Citation2016; Gangoiti, van Leeuwen, Gerwig, et al. Citation2017; Kralj et al. Citation2011). In addition to their scientific relevance, GtfB, GtfC and GtfD type of enzymes represent very interesting and powerful enzymatic tools for the conversion of the starch present in food matrices into soluble novel dietary fibers and/or slowly-digestible carbohydrates, and thus, they have gained substantial interest for the development of healthier starchy food products (Gangoiti, van Leeuwen, Meng, et al. Citation2017). However, only few starch-converting GH70 enzymes have been characterized so far. Whereas GS display a broad linkage specificity, most of the starch-converting GH70 enzymes characterized act as 4,6-α-glucanotransferases (GTases), cleaving (α1→4) linkages and synthesizing new (α1→6) linkages (). Depending on the enzyme, different products are synthesized as a result of this 4,6-α-GTase activity on starch/maltodextrin substrates. For example, the Lactobacillus reuteri 121 GtfB and Exiguobacterium sibiricum 255-15 GtfC disproportionation activity on starch-like substrates results in the synthesis of linear IsoMalto-/Malto-Polysaccharides (IMMP) and IsoMalto-/Malto-Oligosaccharides (IMMO) (), respectively (Gangoiti et al. 2015; Leemhuis et al. Citation2014). Both IMMP and IMMO are ‘hybrid molecules’ and consist of linear (α1→6) glucan chains attached to the non-reducing ends of starch or malto-oligosaccharide fragments, but they significantly differ in their molecular mass. Unlike the L. reuteri 121 GtfB and E. sibiricum GtfC enzymes, the Azotobacter chroococcum and Paenibacillus beijingensis GtfD 4,6-α-GTases are unable of forming consecutive (α1→6) linkages. Using these GtfD enzymes, branched polymers containing mostly (α1→4) linkages and single (α1→6) bridges in linear and branched orientations are obtained from amylose () (Gangoiti, Pijning, and Dijkhuizen Citation2016; Gangoiti, Lamothe, et al. Citation2017; Meng, Gangoiti, Pijning, and Dijkhuizen Citation2016). These polymers resemble the reuteran polysaccharide synthesized by the L. reuteri 121 GtfA GS from sucrose (Dobruchowska et al. Citation2013; Meng, Dobruchowska, et al. Citation2016; Meng, Pijning, et al. Citation2016; van Leeuwen et al. Citation2008), described as a health-promoting food ingredient (Ekhart et al. Citation2006; Plijter et al. Citation2009). In vitro digestibility assays, using either pancreatic α-amylase and amyloglucosidase or rat intestinal maltase-glucoamylase and sucrase-isomaltase, simulating the digestive power of the gastrointestinal tract revealed that the IMMP and reuteran type of polymers have a high dietary fiber content (Gangoiti, Lamothe, et al. Citation2017; Leemhuis et al. Citation2014). The limited diversity in linkage specificity found within these starch-converting GH70 subfamilies of enzymes recently was expanded with the discovery of the Lactobacillus fermentum NCC 2970 GtfB displaying 4,3-α-GTase activity (Gangoiti, van Leeuwen, Gerwig, et al. Citation2017). This L. fermentum NCC 2970 GtfB converts amylose into a branched α-glucan composed of linear (α1→4) segments interconnected by single (α1→3) and (α1→3,4) linkages ().The characterization of this L. fermentum NCC 2970 GtfB 4,3-α-GTase represents an important breakthrough because its 4,3-α-glucan product is unique and different from other naturally occurring, synthetic and enzymatically produced α-glucans.
Figure 6. Composite models of representative novel α-glucans synthesized by starch-converting GH70 enzymes.
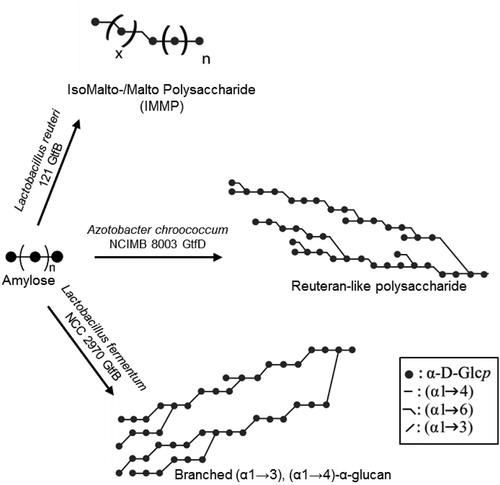
Compared to the limited linkage specificity displayed by other sucrose- and starch-converting α- -transglycosylases, glucans with different types of glycosidic linkages can be obtained [(α1→2), (α1→3), (α1→4) and (α1→6)] by using GS. Over the years, many studies have tried to elucidate what determines the glycosidic linkage specificity in GS enzymes, resulting in synthesis of such a large variety of α-glucans. Variations in product specificity were proposed to be determined by the way in which the acceptor α-glucan is guided into the catalytic center (Leemhuis, Pijning, et al. Citation2013; Vujicic-Zagar et al. Citation2010). The crystal structure of GTF180-ΔN in complex with maltose revealed the residues interacting with this acceptor substrate in subsites +1 and +2 (Vujicic-Zagar et al. Citation2010). Mutagenesis of these key residues have demonstrated that it is the interplay of different amino acid residues defining the acceptor binding subsites what determines the glycosidic linkage specificity in GS (Meng, Pijning, et al. Citation2015; Meng, Dobruchowska, et al. Citation2016; Meng et al. Citation2017). These studies also have shown that predicting the effects of mutations is still complicated. Nevertheless, mutant variants have been obtained producing α-glucans differing in their linkage type, degree of branching, and/or molecular weight. These studies have been recently reviewed by Meng, Gangoiti, Pijning, and Dijkhuizen (Citation2016). Overall, these efforts have significantly enlarged the panel of α-glucans that can be enzymatically produced. Despite the lack of data related to their physicochemical properties, this structural variability is expected to result in differences in a wide range of specific properties, such as solubility, viscosity and resistance to enzymatic hydrolysis by digestive enzymes. Another important advance in GH70 research was the elucidation of the L. reuteri 121 GtfB 4,6-α-GTase 3D structure (). However, binding of oligosaccharides at the acceptor binding subsites has not been observed yet in any of the L. reuteri 121 GtfB crystal structures obtained (Bai et al. Citation2017). It also remains to be studied whether the repertoire of α-glucans synthesized by the starch-converting GtfB, GtfC and GtfD GH70 enzymes could be further expanded by protein engineering approaches. Very recently, the structure of the DSR-M dextransucrase from Leuconostoc citreum NRRL B-1299, producing a single low molar mass dextran has been reported providing important clues about the structural features determining glucan size (Claverie et al. Citation2017).
Attempts to diversify the structure and product size distribution of the α-glucans synthesized by GS using reaction engineering approaches already have been reported. Specifically, the effects of sucrose and enzyme concentrations, temperature and pH have been evaluated. Studies by Robyt and co-workers have shown that the molecular size of dextran is inversely proportional to the concentration of dextransucrase, and directly proportional to the concentration of sucrose and the temperature (Falconer, Mukerjea, and Robyt Citation2011; Robyt, Yoon, and Mukerjea Citation2008). Thus, it was proposed that the best method to obtain α-glucans with a defined molecular mass was the selection of specific enzyme/sucrose concentrations and temperature values. In case of the L. mesenteroides B-512FMCM dextransucrase, changes in sucrose concentration and temperature were found to affect the product size distribution, whereas the pH of the reaction did not have a significant effect (Kim et al. Citation2003). In a more recent study, synthesis of reuteran by L. reuteri 121 GtfA GS at different sucrose concentrations revealed that the ratio of polysaccharide versus oligosaccharide can be modulated by sucrose concentrations (Meng, Dobruchowska, et al. Citation2015). With increasing sucrose concentrations, the production of polysaccharides decreased, while the amount of oligosaccharides increased. However, the linkage distribution and molecular mass of the polysaccharides synthesized at different sucrose concentration was not changed. Another strategy to direct the synthesis toward the production of oligosaccharides at the expense of polymer formation is by adding “good” acceptor substrates (e.g. maltose or isomaltose) to the reaction (Côté and Sheng Citation2006; López-Munguía et al. Citation1993; Meng, Gangoiti, Pijning, and Dijkhuizen Citation2016; Su and Robyt Citation1993). In the presence of low-molecular mass acceptor sugars, GS transfers the glucosyl moieties from sucrose to the acceptor molecule, forming a series of oligosaccharides. The final product distribution can be varied by changing the reaction ratios of sucrose, acceptor substrate and enzyme. A similar approach was used for the synthesis of dextrans with a controlled degree of (α1→2) and (α1→3) linkages using branching sucrases (Brison et al. Citation2010; Vuillemin et al. Citation2016). As dextran is the natural acceptor of branching sucrases, the amount of (α1→2) and (α1→3) side chains was found to be directly dependent on the initial [Sucrose]/[Dextran] molar ratio. Using a suitable [Sucrose]/[Dextran] ratio, dextran polymers grafted with up to 30% of (α1→2) linkages and 50% of (α1→3) linkages, were obtained using the GBD-CD2 and BRS-B enzymes, respectively (Brison et al. Citation2010; Brison et al. Citation2012; Vuillemin et al. Citation2016).
Conclusions
The discovery of novel α-transglycosylases with diverse product specificities has provided important tools for the conversion of starch or sucrose into commercially-valuable slowly digestible or resistant α-glucans. These new carbohydrate ingredients hold a promise of providing digestion controlled materials that can modulate glycemic response or being new dietary fiber sources. At present, α-glucans with different structures (linkage type, branching degree) can be produced by using selected α-transglycosylases. These structural differences result in different digestion rates. A further understanding of the relationship between the structure of these α-glucans and their digestibility is key for the development of α-glucans with a tailored glycemic response. In parallel, a deeper comprehension of α-transglycosylases specificities at the molecular level is still needed to guide the rational design of enzyme variants capable of synthesizing any type of desirable α-glucan regardless of size, linkage type and degree of branching of the product. These joint efforts are expected to allow the synthesis of health-promoting α-glucans of interest for the food-industry.
Declaration of Conflicting Interests
The author(s) declared the following potential conflicts of interest with respect to the research, authorship, and/or publication of this article: LML and CV are employed by the Nestlé Research Center. BRH and LD have received grant/research support from Nestlé Research Center, at least partly used to employ JG and SFG. Authors have jointly filed multiple patent applications around this topic of research and potential products.
References
- Abad, M. C., K. Binderup, J. Rios-Steiner, R. K. Arni, J. Preiss, and J. H. Geiger. 2002. The X-ray crystallographic structure of Escherichia coli branching enzyme. Journal of Biological Chemistry 277(44):42164–70. doi: 10.1074/jbc.M205746200.
- Adisakwattana, S., P. Chantarasinlapin, H. Thammarat, and S. Yibchok-Anun. 2009. A series of cinnamic acid derivatives and their inhibitory activity on intestinal alpha-glucosidase. Journal of Enzyme Inhibition and Medicinal Chemistry 24 (5):1194–200. doi: 10.1080/14756360902779326.
- Albenne, C., L. K. Skov, O. Mirza, M. Gajhede, G. Feller, S. D'Amico, G. Andre, G. Potocki-Veronese, B. A. van der Veen, P. Monsan, and M. Remaud-Simeon. 2004. Molecular basis of the amylose-like polymer formation catalyzed by Neisseria polysaccharea amylosucrase. Journal of Biological Chemistry 279(1):726–34. doi: 10.1074/jbc.M309891200.
- Almdal, T., H. Scharling, J. S. Jensen, and H. Vestergaard. 2004. The independent effect of type 2 diabetes mellitus on ischemic heart disease, stroke, and death: A population-based study of 13,000 men and women with 20 years of follow-up. Archives of Internal Medicine 164 (13):1422–6. doi: 10.1001/archinte.164.13.1422.
- Ao, Z., R. Quezada-Calvillo, L. Sim, B. L. Nichols, D. R. Rose, E. E. Sterchi, and B. R. Hamaker. 2007. Evidence of native starch degradation with human small intestinal maltase-glucoamylase (recombinant). FEBS Letters 581 (13):2381–8. doi: 10.1016/j.febslet.2007.04.035.
- Ao, Z., S. Simsek, G. Zhang, M. Venkatachalam, B. L. Reuhs, and B. R. Hamaker. 2007. Starch with a slow digestion property produced by altering its chain length, branch density, and crystalline structure. Journal of Agricultural and Food Chemistry 55 (11):4540–7. doi: 10.1021/jf063123x.
- Astray, G., Gonzalez-Barreiro, C. Mejuto, J. C. Rial-Otero, R. Simal. G. J, and 2009. A review on the use of cyclodextrins in foods. Food Hydrocolloids 23 (7):1631–40. doi: 10.1016/j.foodhyd.2009.01.001.
- Bai, Y., J. Gangoiti, B. W. Dijkstra, L. Dijkhuizen, and T. Pijning. 2017. Crystal structure of 4,6-α-glucanotransferase supports diet-driven evolution of gh70 enzymes from α-amylases in oral bacteria. Structure 25(2):231–42.
- Bender, H., J. Lehmann, and K. Wallenfels. 1959. Pullulan, an extracellular glucan from pullularia pullulans. Biochimica et Biophysica Acta 36 :309–16.
- Bengs, H., and Brunner, A. (2000). α-amylase-resistant starch for producing foodstuff and medicaments. US 7097831 B1.
- Binderup, K., R. Mikkelsen, and J. Preiss. 2000. Limited proteolysis of branching enzyme from Escherichia coli. Archives of Biochemistry and Biophysics 377(2):366–71. doi: 10.1006/abbi.2000.1815.
- Boos, W., and H. Shuman. 1998. Maltose/maltodextrin system of Escherichia coli: Transport, metabolism, and regulation. Microbiology and Molecular Biology Reviews 62(1):204–29.
- Bornet, F. R., A. M. Fontvieille, S. Rizkalla, P. Colonna, A. Blayo, C. Mercier, and G. Slama. 1989. Insulin and glycemic responses in healthy humans to native starches processed in different ways: Correlation with in vitro alpha-amylase hydrolysis. The American Journal of Clinical Nutrition 50 (2):315–23.
- Breitmeier, D., S. Günther, and H. Heymann. 1997. Acarbose and 1-deoxynojirimycin inhibit maltose and maltooligosaccharide hydrolysis of human small intestinal glucoamylase–maltase in two different substrate-induced modes. Arch Biochem Biophys 346 (1):7–14.
- Brison, Y., E. Fabre, C. Moulis, J. C. Portais, P. Monsan, and S. M. Remaud. 2010. Synthesis of dextrans with controlled amounts of alpha-1,2 linkages using the transglucosidase GBD-CD2. Applied Microbiology and Biotechnology 86 (2):545–54. doi: 10.1007/s00253-009-2241-z.
- Brison, Y., T. Pijning, Y. Malbert, É. Fabre, L. Mourey, S. Morel, G. Potocki-Véronèse, P. Monsan, S. Tranier, M. Remaud-Siméon, and B. W. Dijkstra. 2012. Functional and structural characterization of alpha-(1->2) branching sucrase derived from DSR-E glucansucrase. Journal of Biological Chemistry 287 (11):7915–24. doi: 10.1074/jbc.M111.305078.
- Brownlee, M. 2001. Biochemistry and molecular cell biology of diabetic complications. Nature 414 (6865):813–20. doi: 10.1038/414813a.
- Buckley, J. D., A. A. Thorp, K. J. Murphy, and P. R. Howe. 2006. Dose-dependent inhibition of the post-prandial glycaemic response to a standard carbohydrate meal following incorporation of alpha-cyclodextrin. Annals of Nutrition and Metabolism 50 (2):108–14.
- Büttcher, V., T. Welsh, L. Willmitzer, and J. Kossmann. 1997. Cloning and characterization of the gene for amylosucrase from Neisseria polysaccharea: Production of a linear a-1,4-glucan. Journal of Bacteriology 179(10):3324–30.
- Cambon, E., S. Barbe, S. Pizzut-Serin, M. Remaud-Simeon, and I. Andre. 2014. Essential role of amino acid position 226 in oligosaccharide elongation by amylosucrase from Neisseria polysaccharea. Biotechnology and Bioengineering 111(9):1719–28. doi: 10.1002/bit.25236.
- Campos, C. 2012. Chronic hyperglycemia and glucose toxicity: Pathology and clinical sequelae. Postgraduate Medical Journal 124 (6):90–7. doi: 10.3810/pgm.2012.11.2615.
- Ceriello, A., M. Hanefeld, L. Leiter, L. Monnier, A. Moses, D. Owens, N. Tajima, and J. Tuomilehto. 2004. Postprandial glucose regulation and diabetic complications. Archives of Internal Medicine 164 (19):2090–5. doi: 10.1001/archinte.164.19.2090.
- Chaen, H., T. Nishimoto, T. Nakada, S. Fukuda, M. Kurimoto, and Y. Tsujisaka. 2001. Enzymatic synthesis of kojioligosaccharides using kojibiose phosphorylase. Journal of Bioscience and Bioengineering 92(2):177–82. doi:DOI 10.1263/jbb.92.177.
- Claverie, M., G. Cioci, M. Vuillemin, N. Monties, P. Roblin, G. Lippens, M. Remaud-Simeon, and C. Moulis. 2017. Investigations on the determinants responsible for low molar mass dextran formation by DSR-M dextransucrase. ACS Catalysis 7 (10):7106–19. doi: 10.1021/acscatal.7b02182.
- CODEX Alimentarius Commission for International Food Standards. (2017). General standard for food additive. 192-1995.
- Colleoni, C.,. Dauvill, D. e G. Mouille, A. Bul On, D. Gallant, B. Bouchet, M. Morell, M. Samuel, B. Delrue, C. d'Hulst, C., et al. 1999. Genetic and biochemical evidence for the involvement of alpha-1,4 glucanotransferases in amylopectin synthesis. Plant Physiology 120 (4):993–1004.
- Côté, G. L., and C. D. Skory. 2012. Cloning, expression, and characterization of an insoluble glucan-producing glucansucrase from Leuconostoc mesenteroides NRRL B-1118. Applied Microbiology and Biotechnology 93(6):2387–94.
- Côté, G. L., and J. F. Robyt. 1982. Isolation and partial characterization of an extracellular glucansucrase from Leuconostoc mesenteroides NRRL B-1355 that synthesizes an alternating (1 goes to 6), (1 goes to 3)-alpha-D-glucan. Carbohydrate Research 101(1):57–74.
- Côté, G. L., and S. Sheng. 2006. Penta-, hexa-, and heptasaccharide acceptor products of alternansucrase. Carbohydrate Research 341 (12):2066–72. doi: 10.1016/j.carres.2006.04.044.
- Dahlqvist, A. 1961. The location of carbohydrases in the digestive tract of the pig. Biochemical Journal 78 (2):282–8. doi: 10.1042/bj0780282.
- Dahlqvist, A. 1963. Rat-intestinal dextranase. Localisation and relation to the other carbohydrates of the digestive tract. Biochemical Journal 86(1):72–6.
- Dermaux, L.P., and D. Wills. 2007. Soluble, highly branched glucose polymers for enteral and parenteral nutrition for peritoneal dialysis. US Patent 8445460 B2.
- Dobruchowska, J. M., X. Meng, H. Leemhuis, G. J. Gerwig, L. Dijkhuizen, and J. P. Kamerling. 2013. Gluco-oligomers initially formed by the reuteransucrase enzyme of Lactobacillus reuteri 121 incubated with sucrose and malto-oligosaccharides. Glycobiology 23(9):1084–96. doi: 10.1093/glycob/cwt048.
- Du, X. L., D. Edelstein, L. Rossetti, I. G. Fantus, H. Goldberg, F. Ziyadeh, J. Wu, and M. Brownlee. 2000. Hyperglycemia-induced mitochondrial superoxide overproduction activates the hexosamine pathway and induces plasminogen activator inhibitor-1 expression by increasing Sp1 glycosylation. Proceedings of the National Academy of Sciences of the United States of America 97 (22):12222–6. doi: 10.1073/pnas.97.22.12222.
- EFSA Panel on Dietetic Products, Nutrition and Allergies. 2012. Scientific opinion on the substantiation of health claims related to alpha-cyclodextrin and reduction of post-prandial glycaemic responses (ID 2926, further assessment) pursuant to article 13(1) of regulation 21. No. 1924/20061. EFSA Journal 10 (6):2713.
- EFSA. 2007. Opinion of the scientific panel on dietetic products, nutrition and allergies on a request from the commission related to the safety of alpha-cyclodextrin. EFSA Journal 537 :1–21.
- Ekhart, P., van Geel-Schutten, G. H., Van Binsbergen, M., and Timmerman, E. 2006. Branched alpha-glucans for weight management. US Patent 20060100171 A1.
- Englyst, H. N., S. M. Kingman, and J. H. Cummings. 1992. Classification and measurement of nutritionally important starch fractions. European Journal of Clinical Nutrition 46 Suppl 2:S33–S50.
- Expert Panel Evaluation of Sucromalt for Cargill, lncorporated. 2008. GRAS expert panel evaluation of sucromalt https://www.fda.gov/downloads/Food/IngredientsPackagingLabeling/GRAS/NoticeInventory/UCM264259.pdf.
- Fabre, E., S. Bozonnet, A. Arcache, R.-M. Willemot, M. Vignon, P. Monsan, and M. Remaud-Simeon. 2005. Role of the two catalytic domains of DSR-E dextransucrase and their involvement in the formation of highly alpha-1,2 branched dextran. Journal of Bacteriology 187 (1):296–303. doi: 10.1128/Jb.187.1.296-303.2005.
- Falconer, D. J., R. Mukerjea, and J. F. Robyt. 2011. Biosynthesis of dextrans with different molecular weights by selecting the concentration of Leuconostoc mesenteroides B-512FMC dextransucrase, the sucrose concentration, and the temperature. Carbohydrate Research 346(2):280–4.
- Feng, L., R. Fawaz, S. Hovde, L. Gilbert, J. Chiou, and J. H. Geiger. 2015. Crystal structures of Escherichia coli branching enzyme in complex with linear oligosaccharides. Biochemistry 54(40):6207–18. doi: 10.1021/acs.biochem.5b00228.
- Ferreira, L. M., A. D. Velasquez, S. R. Schaffazick, and L. Cruz. 2015. Pullulan: An advantageous natural polysaccharide excipient to formulate tablets of alendronate-loaded microparticles. Brazilian Journal of Pharmaceutical Sciences 51 (1):27–33. doi: 10.1590/S1984-82502015000100003.
- Gangoiti, J., L. Lamothe, S. van Leeuwen, C. Vafiadi, and L. Dijkhuizen. 2017. Characterization of the paenibacillus beijingensis DSM 24997 GtfD and its glucan polymer products representing a new glycoside hydrolase 70 subfamily of 4,6-α-glucanotransferase enzymes. Plos ONE 12(4):e0172622.
- Gangoiti, J., S. S. van Leeuwen, C. Vafiadi, and L. Dijkhuizen. 2016. The gram-negative bacterium Azotobacter chroococcum NCIMB 8003 employs a new glycoside hydrolase family 70 4,6-alpha-glucanotransferase enzyme (GtfD) to synthesize a reuteran like polymer from maltodextrins and starch. Biochimica et Biophysica Acta 1860(6):1224–36. doi: 10.1016/j.bbagen.2016.02.005.
- Gangoiti, J., S. S. van Leeuwen, G. J. Gerwig, S. Duboux, C. Vafiadi, T. Pijning, and L. Dijkhuizen. 2017. Glucanotransferase, a novel reaction specificity in glycoside hydrolase family 70 and clan GH-h. Scientific Reports 7(1):39761. 3-alpha doi: 10.1038/srep39761.
- Gangoiti, J., S. S. van Leeuwen, X. Meng, S. Duboux, C. Vafiadi, T. Pijning, and L. Dijkhuizen. 2017. Mining novel starch-converting glycoside hydrolase 70 enzymes from the nestlé culture collection genome database: the Lactobacillus reuteri NCC 2613 GTFB. Scientific Reports 7(1):9947. doi: 10.1038/s41598-017-07190-z.
- Gangoiti, J., T. Pijning, and L. Dijkhuizen. 2015. The Exiguobacterium sibiricum 255-15 GtfC enzyme represents a novel glycoside hydrolase 70 subfamily of 4,6-alpha-glucanotransferase enzymes. Applied and Environmental Microbiology 82(2):756–66. doi: 10.1128/AEM.03420-15.
- Gentilcore, D., L. Vanis, J. C. Teng, J. M. Wishart, J. D. Buckley, C. K. Rayner, M. Horowitz, and K. L. Jones. 2011. The oligosaccharide alpha-cyclodextrin has modest effects to slow gastric emptying and modify the glycaemic response to sucrose in healthy older adults. British Journal of Nutrition 106 (04):583–7. doi: 10.1017/S0007114511000444.
- Evoxx Technologies GmbH. 2017. Product fibermalt syrup. Certificate of analysis. Potsdam, Germany http://www.evoxx.com/en/products/carbohydrates/maos-fibermalt/.
- Goffin, D., N. Delzenne, C. Blecker, E. Hanon, C. Deroanne, and M. Paquot. 2011. Will isomalto-oligosaccharides, a well-established functional food in Asia, break through the European and American market? The status of knowledge on these prebiotics. Critical Reviews in Food Science and Nutrition 51(5):394–409.
- Grimaud, F., C. Lancelon-Pin, A. Rolland-Sabaté, X. Roussel, S. Laguerre, A. Viksø-Nielsen, J.-L. Putaux, S. Guilois, A. Buléon, C. D’Hulst, and G. Potocki-Véronèse. 2013. In vitro synthesis of hyperbranched a-glucans using a biomimetic enzymatic toolbox. Biomacromolecules 14(2):438–47. doi: 10.1021/bm301676c.
- Grysman, A., T. Carlson, and T. M. Wolever. 2008. Effects of sucromalt on postprandial responses in human subjects. European Journal of Clinical Nutrition 62 (12):1364–71. doi: 10.1038/sj.ejcn.1602890.
- Gu, Q., Y. Yang, G. Jiang, and G. Chang. 2003. Study on the regulative effect of isomaltooligosaccharides on human intestinal flora. Wei Sheng Yan Jiu 32 (1):54–5.
- Guérin, F., S. Barbe, S. Pizzut-Serin, G. Potocki-Véronèse, D. Guieysse, V. Guillet, P. Monsan, L. Mourey, M. Remaud-Siméon, I. André, and S. Tranier. 2012. Structural investigation of the thermostability and product specificity of amylosucrase from the bacterium Deinococcus geothermalis. Journal of Biological Chemistry 287(9):6642–54. doi: 10.1074/jbc.M111.322917.
- Han, R., J. Li, H. D. Shin, R. R. Chen, G. Du, L. Liu, and J. Chen. 2014. Recent advances in discovery, heterologous expression, and molecular engineering of cyclodextrin glycosyltransferase for versatile applications. Biotechnology Advances 32 (2):415–28. doi: 10.1016/j.biotechadv.2013.12.004.
- Hanada, N., and H. K. Kuramitsu. 1989. Isolation and characterization of the Streptococcus mutans GTFD gene, coding for primer-dependent soluble glucan synthesis. Infection and Immunity 57(7):2079–85.
- Hanefeld, M., and F. Schaper. 2007. The role of alpha-glucosidase inhibitors (acarbose). In: Pharmacotherapy of diabetes: New developments, (13), ed. C. E. Mogensen, 143–152. Boston, MA: Springer US.
- Hanefeld, M., S. Fischer, U. Julius, J. Schulze, U. Schwanebeck, H. Schmechel, H. J. Ziegelasch, and J. Lindner. 1996. Risk factors for myocardial infarction and death in newly detected NIDDM: the diabetes intervention study, 11-year follow-up. Diabetologia 39 (12):1577–83. doi: 10.1007/s001250050617.
- Hansen, M. R., A. Blennow, S. Pedersen, L. Norgaard, and S. B. Engelsen. 2008. Gel texture and chain structure of amylomaltase-modified starches compared to gelatin. Food Hydrocoll 22 (8):1551–66. doi: 10.1016/j.foodhyd.2007.10.010.
- Hasek, L. Y., R. J. Phillips, G. Zhang, K. P. Kinzig, C. Y. Kim, T. L. Powley, and B. R. Hamaker. 2017. Dietary slowly digestible starch triggers the gut-brain axis in obese rats with reduced food intake. Molecular Nutrition & Food Research in press.
- Hasselwander, O., R. DiCosimo, Z. You, Q. Cheng, S. C. Rothman, S. Suwannakham, Z. C. Baer, B. M. Roesch, K. D. Ruebling-Jass, J. P. Lai., et al. 2017. Development of dietary soluble fibres by enzymatic synthesis and assessment of their digestibility in in vitro, animal and randomised clinical trial models. International Journal of Food Sciences and Nutrition 68(7):849–64. doi: 10.1080/09637486.2017.1295027.
- Hayashi, M., R. Suzuki, C. Colleoni, S. G. Ball, N. Fujita, and E. Suzuki. 2015. Crystallization and crystallographic analysis of branching enzymes from cyanothece sp. ATCC 51142. Acta Crystallographica Section F Structural Biology Communications 71(8):1109–13. doi: 10.1107/S2053230X1501198X.
- Hayashi, M., R. Suzuki, C. Colleoni, S. G. Ball, N. Fujita, and E. Suzuki. 2017. Bound substrate in the structure of cyanobacterial branching enzyme supports a new mechanistic model. Journal of Biological Chemistry 292 (13):5465–75. doi: 10.1074/jbc.M116.755629.
- Heymann, H., D. Breitmeier, and S. Gunther. 1995. Human small intestinal sucrase-isomaltase: Different binding patterns for malto- and isomaltooligosaccharides. Biol Chem Hoppe Seyler 376 (4):249–53.
- Hodoniczky, J., C. A. Morris, and A. L. Rae. 2012. Oral and intestinal digestion of oligosaccharides as potential sweeteners: a systematic evaluation. Food Chemistry 132 (4):1951–8. doi: 10.1016/j.foodchem.2011.12.031.
- Imamura, H., S. Fushinobu, M. Yamamoto, T. Kumasaka, B. S. Jeon, T. Wakagi, and H. Matsuzawa. 2003. Crystal structures of 4-alpha-glucanotransferase from Thermococcus litoralis and its complex with an inhibitor. Journal of Biological Chemistry 278(21):19378–86. doi: 10.1074/jbc.M213134200.
- Irwin, W. E., and P. J. Sträter. 1991. Alternative sweeteners. New York: M. Dekker.
- Ishikawa, A.,. H. Yamashita, M. Hiemori, E. Inagaki, M. Kimoto, M. Okamoto, H. Tsuji, A. N. Memon, A. Mohammadi, and Y. Natori. 2007. Characterization of inhibitors of postprandial hyperglycemia from the leaves of nerium indicum. Journal of Nutritional Science and Vitaminology 53(2):166–73. doi: 10.3177/jnsv.53.166.
- Ito, K., S. Ito, T. Shimamura, S. Weyand, Y. Kawarasaki, T. Misaka, K. Abe, T. Kobayashi, A. D. Cameron, and S. Iwata. 2011. Crystal structure of glucansucrase from the dental caries pathogen Streptococcus mutans. Journal of Molecular Biology 408(2):177–86. doi: 10.1016/j.jmb.2011.02.028.
- Jackson, C. A., J. S. Yudkin, and R. D. Forrest. 1992. A comparison of the relationships of the glucose tolerance test and the glycated haemoglobin assay with diabetic vascular disease in the community. The islington diabetes survey. Diabetes Research and Clinical Practice 17 (2):111–23.
- Janecek, S., B. Svensson, and E. A. MacGregor. 2007. A remote but significant sequence homology between glycoside hydrolase clan GH-H and family GH31. FEBS Letters 581 (7):1261–8. doi: 10.1016/j.febslet.2007.02.036.
- Jeanes, A. 1975. Digestibility of food polysaccharides by man: A review. In: Physiological effects of food carbohydrates, 336–347. Washington, D.C.: American Chemical Society.
- Jeanes, A., W. C. Haynes, C. A. Wilham, J. C. Rankin, E. H. Melvin, M. J. Austin, J. E. Cluskey, B. E. Fisher, H. M. Tsuchiya, and C. E. Rist. 1954. Characterization and classification of dextrans from ninety-six strains of bacteria 1b. Journal of the American Chemical Society 76 (20):5041–52.
- Jensen, M. H., O. Mirza, C. Albenne, M. Remaud-Simeon, P. Monsan, M. Gajhede, and L. K. Skov. 2004. Crystal structure of the covalent intermediate of amylosucrase from Neisseria polysaccharea. Biochemistry 43(11):3104–10. doi: 10.1021/bi0357762.
- Jiang, H., J. Lio, M. Blanco, M. Campbell, and J. L. Jane. 2010. Resistant-starch formation in high-amylose maize starch during kernel development. Journal of Agricultural and Food Chemistry 58 (13):8043–7. doi: 10.1021/jf101056y.
- Jiang, H., M. Miao, F. Ye, B. Jiang, and T. Zhang. 2014. Enzymatic modification of corn starch with 4-α-glucanotransferase results in increasing slow digestible and resistant starch. International Journal of Biological Macromolecules 65 :208–14. doi: 10.1016/j.ijbiomac.2014.01.044.
- Jo, H. J., S. Park, H. G. Jeong, J. W. Kim, and J. T. Park. 2015. Vibrio vulnificus glycogen branching enzyme preferentially transfers very short chains: N1 domain determines the chain length transferred. FEBS Letters 589(10):1089–94. doi: 10.1016/j.febslet.2015.03.011.
- Joint FAO/WHO Expert Committee on Food Additives (JECFA). 1993. WHO food additives series 32: Beta-cyclodextrin.
- Joint FAO/WHO Expert Committee on Food Additives (JECFA). 1995. WHO food additives series 35: Beta-cyclodextrin.
- Joint FAO/WHO Expert Committee on Food Additives (JECFA). 1999. WHO food additives series 42: Gamma-cyclodextrin.
- Joint FAO/WHO Expert Committee on Food Additives (JECFA). 2002. WHO food additives series 48: Alpha-cyclodextrin.
- Joint FAO/WHO Expert Committee on Food Additives (JECFA). 2006. WHO food additives series 54: Alpha-cyclodextrin.
- Kadota, K.,. A. Senda, T. Ito, and Y. Tozuka. 2015. Feasibility of highly branched cyclic dextrin as an excipient matrix in dry powder inhalers. European Journal of Pharmaceutical Sciences 79 :79–86. doi: 10.1016/j.ejps.2015.09.006.
- Kajiura, H., Takata, H., Takaha, T., and Kuriki, T. (2006). Method of producing glycogen. EP 1813678 A.
- Kang, H. K., J. S. Oh, and D. Kim. 2009. Molecular characterization and expression analysis of the glucansucrase DSRWC from Weissella cibaria synthesizing a alpha(1->6) glucan. FEMS Microbiology Letters 292(1):33–41. doi: 10.1111/j.1574-6968.2008.01460.x.
- Kaper, T., H. Leemhuis, J. C. Uitdehaag, B. A. van der Veen, B. W. Dijkstra, M. J. van der Maarel, and L. Dijkhuizen. 2007. Identification of acceptor substrate binding subsites +2 and +3 in the amylomaltase from Thermus thermophilus HB8. Biochemistry 46(17):5261–9. doi: 10.1021/bi602408j.
- Kawai, K.,. Y. Okuda, and K. Yamashita. 1985. Changes in blood glucose and insulin after an oral palatinose administration in normal subjects. Endocrinologia Japonica 32 (6):933–6.
- Khan, T., J. K. Park, and J. H. Kwon. 2007. Functional biopolymers produced by biochemical technology considering applications in food engineering. Korean Journal of Chemical Engineering 24 (5):816–26. doi:DOI 10.1007/s11814-007-0047-1.
- Kim, B. K., H. I. Kim, T. W. Moon, and S. J. Choi. 2014. Branch chain elongation by amylosucrase: Production of waxy corn starch with a slow digestion property. Food Chemistry 152 :113–20. doi: 10.1016/j.foodchem.2013.11.145.
- Kim, D., J. F. Robyt, S. Y. Lee, J. H. Lee, and Y. M. Kim. 2003. Dextran molecular size and degree of branching as a function of sucrose concentration, ph, and temperature of reaction of Lactobacillus reuteri B-512 FMCM dextransucrase. Carbohydrate Research 338(11):1183–9. doi: 10.1016/S0008-6215(03)00148-4.
- Kittisuban, P., B. H. Lee, M. Suphantharika, and B. R. Hamaker. 2014. Slow glucose release property of enzyme-synthesized highly branched maltodextrins differs among starch sources. Carbohydrate Polymers 107 :182–91. doi: 10.1016/j.carbpol.2014.02.033.
- Kobayashi, I., M. Tokuda, H. Hashimoto, T. Konda, H. Nakano, and S. Kitahata. 2003. Purification and characterization of a new type of a-glucosidase from Paecilomyces lilacinus that has transglucosylation activity to produce α-(1,3)- and α-(1,2)-linked oligosaccharides. Bioscience, Biotechnology, and Biochemistry 67(1):29–35.
- Koh, D. W., M. O. Park, S. W. Choi, B. H. Lee, and S. H. Yoo. 2016. Efficient biocatalytic production of cyclodextrins by combined action of amylosucrase and cyclodextrin glucanotransferase. Journal of Agricultural and Food Chemistry 64 (21):4371–5. doi: 10.1021/acs.jafc.6b01080.
- Kohmoto, T., K. Tsuji, T. Kaneko, M. Shiota, F. Fukui, H. Takaku, Y. Nakagawa, T. Ichikawa, and S. Kobayash. 1992. Metabolism of (13)C-isomalto-oligosaccharides in healthy men. Bioscience, Biotechnology, and Biochemistry 56 (6):937–40. doi: 10.1271/bbb.56.937.
- Konishi, Y., and K. Shindo. 1997. Production of nigerose, nigerosyl glucose, and nigerosyl maltose by Acremonium sp. S4G13. Bioscience, Biotechnology, and Biochemistry 61(3):439–42.
- Koukiekolo, R., V. Desseaux, Y. Moreau, G. Marchis-Mouren, and M. Santimone. 2001. Mechanism of porcine pancreatic alpha-amylase. Inhibition of amylose and maltopentaose hydrolysis by alpha-, beta- and gamma-cyclodextrins. European Journal of Biochemistry 268(3):841–8.
- Kralj, S., E. Stripling, P. Sanders, G. H. van Geel-Schutten, and L. Dijkhuizen. 2005. Highly hydrolytic reuteransucrase from probiotic Lactobacillus reuteri strain ATCC 55730. Applied and Environmental Microbiology 71(7):3942–50. doi: 10.1128/AEM.71.7.3942-3950.2005.
- Kralj, S., G. H. van Geel-Schutten, H. Rahaoui, R. J. Leer, E. J. Faber, M. J. van der Maarel, and L. Dijkhuizen. 2002. Molecular characterization of a novel glucosyltransferase from Lactobacillus reuteri strain 121 synthesizing a unique, highly branched glucan with alpha-(1->4) and alpha-(1->6) glucosidic bonds. Applied and Environmental Microbiology 68(9):4283–91.
- Kralj, S., P. Grijpstra, S. S. van Leeuwen, H. Leemhuis, J. M. Dobruchowska, R. M. van der Kaaij, A. Malik, A. Oetari, J. P. Kamerling, and L. Dijkhuizen. 2011. Glucanotransferase, a novel enzyme that structurally and functionally provides an evolutionary link between glycoside hydrolase enzyme families 13 and 70. Applied and Environmental Microbiology 77(22):8154–63. 4,6-alpha doi: 10.1128/AEM.05735-11.
- Kralj, S., van, G.-S. Dondorff, G. H. M. M. Kirsanovs, S. van der, M. M. J. Dijkhuizen. and L. 2004. Glucan synthesis in the genus lactobacillus: Isolation and characterization of glucansucrase genes, enzymes and glucan products from six different strains. Microbiology 150(11):3681–90. doi: 10.1099/mic.0.27321-0.
- Larson, S. B., J. S. Day, and A. McPherson. 2010. X-ray crystallographic analyses of pig pancreatic alpha-amylase with limit dextrin, oligosaccharide, and alpha-cyclodextrin. Biochemistry 49 (14):3101–15. doi: 10.1021/bi902183w.
- Le, Q. T., C. K. Lee, Y. W. Kim, S. J. Lee, R. Zhang, S. G. Withers, Y. R. Kim, J. H. Auh, and K. H. Park. 2009. Amylolytically-resistant tapioca starch modified by combined treatment of branching enzyme and maltogenic amylase. Carbohydrate Polymers 75 (1):9–14. doi: 10.1016/j.carbpol.2008.06.001.
- Leathers, T. D., Hayman, G. T., and Côté, G. L. (1997). Microorganism strains that produce a high proportion of alternan to dextran. US Patent 5702942.
- Lee, B. H., A. H. Lin, B. L. Nichols, K. Jones, D. R. Rose, R. Quezada-Calvillo, and B. R. Hamaker. 2014. Mucosal c-terminal maltase-glucoamylase hydrolyzes large size starch digestion products that may contribute to rapid postprandial glucose generation. Molecular Nutrition & Food Research 58 (5):1111–21. doi: 10.1002/mnfr.201300599.
- Lee, B. H., D. R. Rose, A. H. Lin, R. Quezada-Calvillo, B. L. Nichols, and B. R. Hamaker. 2016. Contribution of the individual small intestinal alpha-glucosidases to digestion of unusual alpha-linked glycemic disaccharides. Journal of Agricultural and Food Chemistry 64 (33):6487–94. doi: 10.1021/acs.jafc.6b01816.
- Lee, B. H., R. Eskandari, K. Jones, K. R. Reddy, R. Quezada-Calvillo, B. L. Nichols, D. R. Rose, B. R. Hamaker, and B. M. Pinto. 2012. Modulation of starch digestion for slow glucose release through "toggling" of activities of mucosal alpha-glucosidases. Journal of Biological Chemistry 287 (38):31929–38. doi: 10.1074/jbc.M112.351858.
- Lee, B.-H., L. Yan, R. J. Phillips, B. L. Reuhs, K. Jones, D. R. Rose, B. L. Nichols, R. Quezada-Calvillo, S.-H. Yoo, and B. R. Hamaker. 2013. Enzyme-synthesized highly branched maltodextrins have slow glucose generation at the mucosal α-glucosidase level and are slowly digestible in vivo. Purdue University.
- Lee, H. S., J. H. Auh, H. G. Yoon, M. J. Kim, J. H. Park, S. S. Hong, M. H. Kang, T. J. Kim, T. W. Moon, J. W. Kim, and K. H. Park. 2002. Cooperative action of alpha-glucanotransferase and maltogenic amylase for an improved process of isomaltooligosaccharide (Imo) production. Journal of Agricultural and Food Chemistry 50 (10):2812–7. doi:jf011529y [pii].
- Lee, S. J., S. H. Yoo, M. J. Kim, J. W. Kim, H. M. Seok, and K. H. Park. 1995. Production and characterization of branched oligosaccharides from liquefied starch by the action of bacillus-licheniformis amylase. Starch - Stärke 47(4):127–34. doi:DOI 10.1002/star.19950470403.
- Leemhuis, H., J. M. Dobruchowska, M. Ebbelaar, F. Faber, P. L. Buwalda, M. J. E. C. van der Maarel, J. P. Kamerling, and L. Dijkhuizen. 2014. Isomalto/malto-polysaccharide, a novel soluble dietary fiber made via enzymatic conversion of starch. Journal of Agricultural and Food Chemistry 62 (49):12034–44. doi: 10.1021/jf503970a.
- Leemhuis, H., R. M. Kelly, and L. Dijkhuizen. 2010. Engineering of cyclodextrin glucanotransferases and the impact for biotechnological applications. Applied Microbiology and Biotechnology 85 (4):823–35. doi: 10.1007/s00253-009-2221-3.
- Leemhuis, H., T. Pijning, J. M. Dobruchowska, S. S. van Leeuwen, S. Kralj, B. W. Dijkstra, and L. Dijkhuizen. 2013. Glucansucrases: Three-dimensional structures, reactions, mechanism, alpha-glucan analysis and their implications in biotechnology and food applications. Journal of Biotechnology 163 (2):250–72. doi: 10.1016/j.jbiotec.2012.06.037.
- Leemhuis, H., W. P. Dijkman, J. M. Dobruchowska, T. Pijning, P. Grijpstra, S. Kralj, J. P. Kamerling, and L. Dijkhuizen. 2013. 4,6-alpha-glucanotransferase activity occurs more widespread in lactobacillus strains and constitutes a separate GH70 subfamily. Applied Microbiology and Biotechnology 97(1):181–93. doi: 10.1007/s00253-012-3943-1.
- Lehman, U., and F. Robin. 2007. Slowly digestible starch – its structure and health implications: A review. Trends in Food Science & Technology 18(7):346–55.
- Light, S. H., L. A. Cahoon, K. V. Mahasenan, M. Lee, B. Boggess, A. S. Halavaty, S. Mobashery, N. E. Freitag, and W. F. Anderson. 2017. Transferase versus hydrolase: the role of conformational flexibility in reaction specificity. Structure 25 (2):295–304. doi: 10.1016/j.str.2016.12.007.
- Lin, A. H., B. L. Nichols, R. Quezada-Calvillo, S. E. Avery, L. Sim, D. R. Rose, H. Y. Naim, and B. R. Hamaker. 2012. Unexpected high digestion rate of cooked starch by the ct-maltase-glucoamylase small intestine mucosal alpha-glucosidase subunit. PLoS One 7 (5):e35473. doi: 10.1371/journal.pone.0035473.
- Lin, A. H.-M., B.-H. Lee, and W.-J. Chang. 2016. Small intestine mucosal α-glucosidase: a missing feature of in vitro starch digestibility. Food Hydrocolloids 53 :163–71. doi: 10.1016/j.foodhyd.2015.03.002.
- Liu, C., W. Xiang, Y. Yu, Z. Q. Shi, X. Z. Huang, and L. Xu. 2015. Comparative analysis of 1-deoxynojirimycin contribution degree to alpha-glucosidase inhibitory activity and physiological distribution in morus Alba l. Industrial Crops and Products 70 :309–15. doi: 10.1016/j.indcrop.2015.02.046.
- Liu, Y., X. Ban, C. Li, Z. Gu, L. Cheng, Y. Hong, and Z. Li. 2017. Met349 mutations enhance the activity of 1,4-α-glucan branching enzyme from geobacillus thermoglucosidans STB02. Journal of Agricultural and Food Chemistry 65(28):5674–80.
- López-Munguía, A., V. Pelenc, M. Remaud, J. Biton, J. M. Michel, C. Lang, F. Paul, and P. Monsan. 1993. Production and purification of alternansucrase, a glucosyltransferase from Leuconostoc mesenteroides NRRL B-1355, for the synthesis of oligoalternans. Enzyme and Microbial Technology 15(1):77–85.
- MacGregor, E. A., H. M. Jespersen, and B. Svensson. 1996. A circularly permuted alpha-amylase-type alpha/beta-barrel structure in glucan-synthesizing glucosyltransferases. FEBS Letters 378 (3):263–6.
- Madsen, L. R., S. Stanley, P. Swann, and J. Oswald. 2017. A survey of commercially available isomaltooligosaccharide-based food ingredients. Journal of Food Science 82 (2):401–8. doi: 10.1111/1750-3841.13623.
- Maina, N. H., L. Virkki, H. Pynno¨Nen, H. Maaheimo, and M. Tenkanen. 2011. Structural analysis of enzyme-resistant isomaltooligosaccharides reveals the elongation of alpha-(1->3)-linked branches in Weissella confusa dextran. Biomacromolecules 12(2):409–18. doi: 10.1021/bm1011536.
- Martin, A. E., and P. A. Montgomery. 1996. Acarbose: An alpha-glucosidase inhibitor. American Journal of Health-System Pharmacy: Ajhp: Official Journal of the American Society of Health-System Pharmacists 53 (19):2277–90. quiz 2336-2277.
- Meigs, J. B., D. M. Nathan, R. B. D'Agostino, Sr., P. W. Wilson, and S. Framingham Offspring. 2002. Fasting and postchallenge glycemia and cardiovascular disease risk: the framingham offspring study. Diabetes Care 25(10):1845–50. doi: 10.2337/diacare.25.10.1845.
- Meng, X. F., J. Gangoiti, Y. X. Bai, T. Pijning, S. S. Van Leeuwen, and L. Dijkhuizen. 2016. Structure-function relationships of family GH70 glucansucrase and 4,6-alpha-glucanotransferase enzymes, and their evolutionary relationships with family GH13 enzymes. Cellular and Molecular Life Sciences 73 (14):2681–706. doi: 10.1007/s00018-016-2245-7.
- Meng, X., J. M. Dobruchowska, G. J. Gerwig, J. P. Kamerling, and L. Dijkhuizen. 2015. Synthesis of oligo- and polysaccharides by Lactobacillus reuteri 121 reuteransucrase at high concentrations of sucrose. Carbohydrate Research 414 :85–92. doi: 10.1016/j.carres.2015.07.011.
- Meng, X., J. M. Dobruchowska, T. Pijning, G. J. Gerwig, and L. Dijkhuizen. 2016. Synthesis of new hyperbranched alpha-glucans from sucrose by Lactobacillus reuteri 180 glucansucrase mutants. Journal of Agricultural and Food Chemistry 64(2):433–42. doi: 10.1021/acs.jafc.5b05161.
- Meng, X., T. Pijning, J. M. Dobruchowska, G. J. Gerwig, and L. Dijkhuizen. 2015. Characterization of the functional roles of amino acid residues in acceptor-binding subsite +1 in the active site of the glucansucrase gtf180 from Lactobacillus reuteri 180. Journal of Biological Chemistry 290(50):30131–41. doi: 10.1074/jbc.M115.687558.
- Meng, X., T. Pijning, J. M. Dobruchowska, H. Yin, G. J. Gerwig, and L. Dijkhuizen. 2016. Structural determinants of alternating (alpha1 -> 4) and (alpha1 -> 6) linkage specificity in reuteransucrase of Lactobacillus reuteri. Scientific Reports 6(1):35261. doi: 10.1038/srep35261.
- Meng, X., T. Pijning, M. Tietema, J. M. Dobruchowska, H. Yin, G. J. Gerwig, S. Kralj, and L. Dijkhuizen. 2017. Characterization of the glucansucrase Gtf180 W1065 mutant enzymes producing polysaccharides and oligosaccharides with altered linkage composition. Food Chemistry 217 :81–90. doi: 10.1016/j.foodchem.2016.08.087.
- Mirza, O., L. K. Skov, M. Remaud-Simeon, G. Potocki de Montalk, C. Albenne, P. Monsan, and M. Gajhede. 2001. Crystal structures of amylosucrase from Neisseria polysaccharea in complex with D-glucose and the active site mutant Glu328Gln in complex with the natural substrate sucrose. Biochemistry 40(30):9032–9.
- Mitchell, H., M. H. Auerbach, and F. K. Moppet. 2001. Polydextrose. New York: L. O. Nabors. Marcel Dekker, 499–518.
- Monchois, V., M. Remaud-Simeon, R. R. Russell, P. Monsan, and R. M. Willemot. 1997. Characterization of Lactobacillus reuteri NRRL B-512f dextransucrase (DSRS) and identification of amino-acid residues playing a key role in enzyme activity. Applied Microbiology and Biotechnology 48(4):465–72.
- Monchois, V., R. M. Willemot, and P. Monsan. 1999. Glucansucrases: Mechanism of action and structure-function relationships. FEMS Microbiology Reviews 23 (2):131–51. doi:S0168-6445(98)00041-2 [pii].
- Monnier, L., E. Mas, C. Ginet, F. Michel, L. Villon, J. P. Cristol, and C. Colette. 2006. Activation of oxidative stress by acute glucose fluctuations compared with sustained chronic hyperglycemia in patients with type 2 diabetes. JAMA 295 (14):1681–7. doi: 10.1001/jama.295.14.1681.
- Monsan, P., Remaud, S. Andre. M., and I. 2010. Transglucosidases as efficient tools for oligosaccharide and glucoconjugate synthesis. Current Opinion in Microbiology 13 (3):293–300. doi: 10.1016/j.mib.2010.03.002.
- Moulis, C., I. Andre, and M. Remaud-Simeon. 2016. GH13 amylosucrases and GH70 branching sucrases, atypical enzymes in their respective families. Cellular and Molecular Life Sciences 73 (14):2661–79. doi: 10.1007/s00018-016-2244-8.
- Mountzouris, K. C., S. G. Gilmour, and R. A. Rastall. 2002. Continuous production of oligodextrans via controlled hydrolysis of dextran in an enzyme membrane reactor. Journal of Food Science 67 (5):1767–71. doi:DOI 10.1111/j.1365-2621.2002.tb08720.x.
- Na, S., M. Park, I. Jo, J. Cha, and N. C. Ha. 2017. Structural basis for the transglycosylase activity of a GH57-type glycogen branching enzyme from Pyrococcus horikoshii. Biochemical and Biophysical Research Communications 484(4):850–6. doi: 10.1016/j.bbrc.2017.02.002.
- Naessens, M., A. Cerdobbel, W. Soetaert, and E. J. Vandamme. 2005. Dextran dextrinase and dextran of Gluconobacter oxydans. Journal of Industrial Microbiology & Biotechnology 32(8):323–34. doi: 10.1007/s10295-005-0259-5.
- Nguyen, T. T. H., S.-H. Jung, S. Lee, H.-J. Ryu, H.-K. Kang, Y.-H. Moon, Y.-M. Kim, A. Kimura, and D. Kim. 2012. Inhibitory effects of epigallocatechin gallate and its glucoside on the human intestinal maltase inhibition. Biotechnology and Bioprocess Engineering 17 (5):966–71. doi: 10.1007/s12257-012-0242-8.
- Nichols, B. L., S. Avery, P. Sen, D. M. Swallow, D. Hahn, and E. Sterchi. 2003. The maltase-glucoamylase gene: Common ancestry to sucrase-isomaltase with complementary starch digestion activities. Proceedings of the National Academy of Sciences of the United States of America 100 (3):1432–7. doi: 10.1073/pnas.0237170100.
- Nishikawa, T., D. Edelstein, X. L. Du, S. Yamagishi, T. Matsumura, Y. Kaneda, M. A. Yorek, D. Beebe, P. J. Oates, H. P. Hammes., et al. 2000. Normalizing mitochondrial superoxide production blocks three pathways of hyperglycaemic damage. Nature 404 (6779):787–90. doi: 10.1038/35008121.
- Niu, D. D., J. Qiao, P. J. Li, K. M. Tian, X. G. Liu, S. Singh, and F. Lu. 2017. Highly efficient enzymatic preparation of isomalto-oligosaccharides from starch using an enzyme cocktail. Electronic Journal of Biotechnology 26 :46–51. doi: 10.1016/j.ejbt.2016.12.002.
- Norman, B., Pedersen, S., Stanley, K., Stanley, E., and Richmond, P. (2007). Production of crystalline short chain amylase. US Patent 2007059432.
- Nugent, A. P. 2005. Health properties of resistant starch. Nutrition Bulletin 30(1):27–54.
- Oates, C. G. 1997. Towards an understanding of starch granule structure and hydrolysis. Trends in Food Science & Technology 8 (11):375–82. doi:Doi 10.1016/S0924-2244(97)01090-X.
- Office of Food Additive Safety (HFS-200) Center for Food Safety and Applied Nutrition (CFSAN) Food and Drug Administration (FDA). 2010. GRAS Notice 000358: Cyclic dextrin, highly branched https://fradowner.com/d/db352e5e.
- Ohkuma, K., and S. Wakabayashi. 2000. Fibersol-2: A soluble, non-digestible, starch-derived dietary fibre. In: Advanced dietary fibre technology, 509–523. Hoboken, NJ: Blackwell Science Ltd.
- Pal, K., S. Kumar, S. Sharma, S. K. Garg, M. S. Alam, H. E. Xu, P. Agrawal, and K. Swaminathan. 2010. Crystal structure of full-length Mycobacterium tuberculosis H37RV glycogen branching enzyme: Insights of N-terminal beta-sandwich in substrate specificity and enzymatic activity. Journal of Biological Chemistry 285(27):20897–903. doi: 10.1074/jbc.M110.121707.
- Palomo, M., S. Kralj, M. J. van der Maarel, and L. Dijkhuizen. 2009. The unique branching patterns of deinococcus glycogen branching enzymes are determined by their N-terminal domains. Applied and Environmental Microbiology 75 (5):1355–62. doi: 10.1128/AEM.02141-08.
- Palomo, M., T. Pijning, T. Booiman, J. M. Dobruchowska, J. van der Vlist, S. Kralj, A. Planas, K. Loos, J. P. Kamerling, B. W. Dijkstra., et al. 2011. Thermus thermophilus glycoside hydrolase family 57 branching enzyme: Crystal structure, mechanism of action, and products formed. Journal of Biological Chemistry 286(5):3520–30. doi: 10.1074/jbc.M110.179515.
- Panasevich, M. R., K. R. Kerr, M. C. Serao, M. R. de Godoy, L. Guerin-Deremaux, G. L. Lynch, D. Wils, S. E. Dowd, G. C. Fahey, K. S. Swanson, and R. N. Dilger. 2015. Evaluation of soluble corn fiber on chemical composition and nitrogen-corrected true metabolizable energy and its effects on in vitro fermentation and in vivo responses in dogs. Journal of Animal Science 93(5):2191–200. doi: 10.2527/jas.2014-8425.
- Park, J. K. and T. Khan. 2009. Other microbial polysaccharides: Pullulan, scleroglucan, elsinan, levan, alternant, dextran. In: Handbook of Hydrocolloids, 592–614 Cambridge, UK:Woodhead Publishing Limited. doi: 10.1533/9781845695873.592.
- Passerini, D., M. Vuillemin, L. Ufarte, S. Morel, V. Loux, C. Fontagne-Faucher, P. Monsan, M. Remaud-Simeon, and C. Moulis. 2015. Inventory of the GH70 enzymes encoded by Leuconostoc citreum NRRL B-1299 - identification of three novel alpha-transglucosylases. FEBS Journal 282(11):2115–30. doi: 10.1111/febs.13261.
- Paul, F., Lopez-Munguia, A., Remaud, M., Pelenc, V., and Monsan, P. (1992). Method for the production of α-1,2 oligodextrans using Leuconostoc mesenteroides NRRL B-1299. US Patent 5141858 A.
- Pelzer, S., Zurek, C., Rose, T., Eek, J. I., Wach, W., Klingeberg, M., and Harms, K. (2012). Microorganisms having enhanced sucrose mutase activity. US Patent 8790900 B2.
- Peters, S., T. Rose, and M. Moser. 2010. Sucrose: a prospering and sustainable organic raw material. Topics in Current Chemistry 294 :1–23.
- Pijning, T., A. Vujicic-Zagar, S. Kralj, L. Dijkhuizen, and B. W. Dijkstra. 2012. Structure of the alpha-1,6/alpha-1,4-specific glucansucrase GtfA from Lactobacillus reuteri 121. Acta Crystallographica Section F Structural Biology and Crystallization Communications 68(12):1448–54. doi: 10.1107/S1744309112044168.
- Playne, M. J., and R. G. Crittenden. 2004. Prebiotics from lactose, sucrose, starch and plant polysaccharides. Bioprocesses and Biotechnology for Functional Foods and Nutraceuticals In. F. Shahidi, eds. J. R. Neeser and J. B. German. New York: Marcel Dekker.
- Plijter, J., Jurgens, A., Kats, M. P., Noort, M. W. J., Heddes, C. E. A., and Van, G. S. (2009). Bread improver. US Patent 20090297663 A1.
- Potocki-Veronese, G., J. L. Putaux, D. Dupeyre, C. Albenne, M. Remaud-Simeon, P. Monsan, and A. Buleon. 2005. Amylose synthesized in vitro by amylosucrase: Morphology, structure, and properties. Biomacromolecules 6(2):1000–11. doi: 10.1021/bm049326g.
- Przylas, I., Y. Terada, K. Fujii, T. Takaha, W. Saenger, and N. Strater. 2000. X-ray structure of acarbose bound to amylomaltase from Thermus aquaticus. Implications for the synthesis of large cyclic glucans. European Journal of Biochemistry 267(23):6903–13. doi:ejb1790 [pii].
- Putaala, H. (2013). Polydextrose in Lipid Metabolism, Lipid Metabolism, Prof. Rodrigo Valenzuela Baez (Ed.), InTech, DOI: 10.5772/51791. https://www.intechopen.com/books/lipid-metabolism/polydextrose-in-lipid-metabolism
- Quezada-Calvillo, R., C. C. Robayo-Torres, Z. Ao, B. R. Hamaker, A. Quaroni, G. D. Brayer, E. E. Sterchi, S. S. Baker, and B. L. Nichols. 2007. Luminal substrate " brake" on mucosal maltase- glucoamylase activity regulates total rate of starch digestion to glucose. Journal of Pediatric Gastroenterology and Nutrition 45 (1):32–43.
- Quezada-Calvillo, R., L. Sim, Z. H. Ao, B. R. Hamaker, A. Quaroni, G. D. Brayer, E. E. Sterchi, C. C. Robayo-Torres, D. R. Rose, and B. L. Nichols. 2008. Luminal starch substrate "brake" on maltase-glucoamylase activity is located within the glucoamylase subunit. The Journal of Nutrition 138 (4):685–92.
- Remaud-Simeon, M., A. Lopez-Munguia, V. Pelenc, F. Paul, and P. Monsan. 1994. Production and use of glucosyltransferases from Leuconostoc mesenteroides NRRL B-1299 for the synthesis of oligosaccharides containing α-(1-2) linkages. Applied Biochemistry and Biotechnology 44(2):101–17.
- Rennhard, H. H. (1973). Polysaccharides and their preparation. US Patent 3766165 A.
- Robyt, J. F., S. H. Yoon, and R. Mukerjea. 2008. Dextransucrase and the mechanism for dextran biosynthesis. Carbohydrate Research 343 (18):3039–48. doi: 10.1016/j.carres.2008.09.012.
- Rolland-Sabaté, A., P. Colonna, G. Potocki-Veronese, P. Monsan, and V. Planchot. 2004. Elongation and insolubilisation of α-glucans by the action of Neisseria polysaccharea amylosucrase. Journal of Cereal Science 40(1):17–30.
- Roper, H., and H. Koch. 1988. New carbohydrate-derivatives from biotechnical and chemical processes. Starch 40 (12):453–64. doi:DOI 10.1002/star.19880401203.
- Roth, C., N. Weizenmann, N. Bexten, W. Saenger, W. Zimmermann, T. Maier, and N. Strater. 2017. Amylose recognition and ring-size determination of amylomaltase. Science Advances 3 (1):e1601386. doi: 10.1126/sciadv.1601386.
- Roujeinikova, A., C. Raasch, J. Burke, P. J. Baker, W. Liebl, and D. W. Rice. 2001. The crystal structure of thermotoga maritima maltosyltransferase and its implications for the molecular basis of the novel transfer specificity. Journal of Molecular Biology 312(1):119–31. doi: 10.1006/jmbi.2001.4944.
- Roujeinikova, A., C. Raasch, S. Sedelnikova, W. Liebl, and D. W. Rice. 2002. Crystal structure of Thermotoga maritima 4-alpha-glucanotransferase and its acarbose complex: Implications for substrate specificity and catalysis. Journal of Molecular Biology321(1):149–62. doi:S0022283602005703 [pii].
- Roussel, X., C. Lancelon-Pin, A. Vikso-Nielsen, A. Rolland-Sabate, F. Grimaud, G. Potocki-Veronese, A. Buleon, J. L. Putaux, and C. D'Hulst. 2013. Characterization of substrate and product specificity of the purified recombinant glycogen branching enzyme of Rhodothermus obamensis. Biochimica et Biophysica Acta 1830(1):2167–77. doi: 10.1016/j.bbagen.2012.09.022.
- Rycroft, C. E., M. R. Jones, G. R. Gibson, and R. A. Rastall. 2001. A comparative in vitro evaluation of the fermentation properties of prebiotic oligosaccharides. Journal of Applied Microbiology 91(5):878–87.
- Sadahiro, J., H. Mori, W. Saburi, M. Okuyama, and A. Kimura. 2015. Extracellular and cell-associated forms of Gluconobacter oxydans dextran dextrinase change their localization depending on the cell growth. Biochemical and Biophysical Research Communications 456(1):500–5. doi: 10.1016/j.bbrc.2014.11.115.
- Sawada, T., Y. Nakamura, T. Ohdan, A. Saitoh, P. B. Francisco, Jr., E. Suzuki, N. Fujita, T. Shimonaga, S. Fujiwara, M. Tsuzuki., et al. 2014. Diversity of reaction characteristics of glucan branching enzymes and the fine structure of alpha-glucan from various sources. Archives of Biochemistry and Biophysics 562 :9–21. doi: 10.1016/j.abb.2014.07.032.
- Schneider, J., C. Fricke, H. Overwin, B. Hofmann, and B. Hofer. 2009. Generation of amylosucrase variants that terminate catalysis of acceptor elongation at the di- or trisaccharide stage. Applied and Environmental Microbiology 75 (23):7453–60. doi: 10.1128/AEM.01194-09.
- SCO. 2000. Opinion of the scientific committee on food on a dextran preparation, produced using Leuconostoc mesenteroides, Saccharomyces cerevisae and lactobacillus spp, as a novel food ingredient in bakery products. Brussels, Belgium.
- Shin, H. J., S. J. Choi, C. S. Park, and T. W. P. Moon. 2010. Preparation of starches with low glycaemic response using amylosucrase and their physicochemical properties. Carbohydrate Polymers 82(2):489–97.
- Shinohara, M. L., M. Ihara, M. Abo, M. Hashida, S. Takagi, and T. C. Beck. 2001. A novel thermostable branching enzyme from an extremely thermophilic bacterial species, Rhodothermus obamensis. Applied Microbiology and Biotechnology 57(5-6):653–9.
- Shiroza, T., S. Ueda, and H. K. Kuramitsu. 1987. Sequence analysis of the GTFB gene from Streptococcus mutans. Journal of Bacteriology 169(9):4263–70.
- Siddiqui, I. R., and B. Furgala. 1967. Isolation and characterization of oligosaccharides from honey. Part i disaccharides. Journal of Apicultural Research 6(3):139–45.
- Sim, L., C. Willemsma, S. Mohan, H. Y. Naim, B. M. Pinto, and D. R. Rose. 2010. Structural basis for substrate selectivity in human maltase-glucoamylase and sucrase-isomaltase N-terminal domains. Journal of Biological Chemistry 285 (23):17763–70. doi: 10.1074/jbc.M109.078980.
- Simpson, D. T. (2011). Printing ink base material. Google Patents.
- Simsek, M., R. Quezada-Calvillo, M. G. Ferruzzi, B. L. Nichols, and B. R. Hamaker. 2015. Dietary phenolic compounds selectively inhibit the individual subunits of maltase-glucoamylase and sucrase-isomaltase with the potential of modulating glucose release. Journal of Agricultural and Food Chemistry 63 (15):3873–9. doi: 10.1021/jf505425d.
- Skov, L. K., S. Pizzut-Serin, M. Remaud-Simeon, H. A. Ernst, M. Gajhede, and O. Mirza. 2013. The structure of amylosucrase from Deinococcus radiodurans has an unusual open active-site topology. Acta Crystallographica Section F Structural Biology and Crystallization Communications 69(9):973–8. doi: 10.1107/S1744309113021714.
- Srisuvor, N., N. Chinprahast, C. Prakitchaiwattana, and S. Subhimaros. 2013. Effects of inulin and polydextrose on physicochemical and sensory properties of low-fat set yoghurt with probiotic-cultured banana purée. LWT - Food Sci Technol 51 (1):30–6. doi: 10.1016/j.lwt.2012.10.018.
- Su, D., and J. F. Robyt. 1993. Control of the synthesis of dextran and acceptor-products by Leuconostoc mesenteroides B-512FM dextransucrase. Carbohydrate Research 248 :339–48. doi:0008-6215(93)84139-W [pii].
- Suzuki, E., and R. Suzuki. 2016. Distribution of glucan-branching enzymes among prokaryotes. Cellular and Molecular Life Sciences 73 (14):2643–60. doi: 10.1007/s00018-016-2243-9.
- Takata, H., K. Ohdan, T. Takaha, T. Kuriki, and S. Okada. 2003. Properties of branching enzyme from hyperthermophilic bacterium, Aquifex aeolicus, and its potential for production of highly-branched cyclic dextrin. Journal of Applied Glycoscience 50(1):15–20.
- Takata, H., T. Takaha, S. Okada, S. Hizukuri, M. Takagi, and T. Imanaka. 1996. Structure of the cyclic glucan produced from amylopectin by Bacillus stearothermophilus branching enzyme. Carbohydrate Research 295 :91–101.
- Takazoe, I. 1985. New trends on sweeteners in Japan. International Dental Journal 35 (1):58–65.
- Takii, H., K. Ishihara, T. Kometani, S. Okada, and T. Fushiki. 1999. Enhancement of swimming endurance in mice by highly branched cyclic dextrin. Biosci Biotechnol Biochem 63 (12):2045–52.
- Terada, Y., K. Fujii, T. Takaha, and S. Okada. 1999. Thermus aquaticus ATCC 33923 amylomaltase gene cloning and expression and enzyme characterization: Production of cycloamylose. Applied and Environmental Microbiology 65(3):910–5.
- Thiemann, V., C. D?Nges, S. G. Prowe, R. Sterner, and G. Antranikian. 2004. Characterisation of a thermoalkali-stable cyclodextrin glycosyltransferase from the anaerobic thermoalkaliphilic bacterium Anaerobranca gottschalkii. Archives of Microbiology 182(2-3):226–35. doi: 10.1007/s00203-004-0717-x.
- Tsuji, Y., K. Yamada, N. Hosoya, and S. Moriuchi. 1986. Digestion and absorption of sugars and sugar substitutes in rat small intestine. Journal of Nutritional Science and Vitaminology 32 (1):93–100.
- Tsunehiro, J., K. Okamoto, Y. Furuyama, T. Yatake, and T. Kaneko. 1999. Digestibility of the hydrogenated derivative of an isomaltooligosaccharide mixture by rats. Bioscience, Biotechnology, and Biochemistry 63 (9):1515–21. doi: 10.1271/bbb.63.1515.
- Uitdehaag, J. C. M., G. J. W. M. van Alebeek, B. A. van der Veen, L. Dijkhuizen, and B. W. Dijkstra. 2000. Structures of maltohexaose and maltoheptaose bound at the donor sites of cyclodextrin glycosyltransferase give insight into the mechanisms of transglycosylation activity and cyclodextrin size specificity. Biochemistry 39 (26):7772–80. doi: 10.1021/bi000340x.
- Uitdehaag, J. C., R. Mosi, K. H. Kalk, B. A. van der Veen, L. Dijkhuizen, S. G. Withers, and B. W. Dijkstra. 1999. X-ray structures along the reaction pathway of cyclodextrin glycosyltransferase elucidate catalysis in the alpha-amylase family. Nature Structural & Molecular Biology 6 (5):432–6. doi: 10.1038/8235.
- Valette, P., V. Pelenc, Z. Djouzi, C. Andrieux, F. Paul, P. Monsan, and O. Szylit. 1993. Bioavailability of new synthesized glucooligosaccharides in the intestinal-tract of gnotobiotic-rats. Journal of the Science of Food and Agriculture 62 (2):121–7. doi: 10.1002/jsfa.2740620204.
- Valk, V.,. W. Eeuwema, F. D. Sarian, R. M. van der Kaaij, and L. Dijkhuizen. 2015. Degradation of granular starch by the bacterium Microbacterium aurum strain B8.A involves a modular alpha-amylase enzyme system with FNiii and CBM25 domains. Applied and Environmental Microbiology 81(19):6610–20. doi: 10.1128/AEM.01029-15.
- van Can, J. G., L. J. van Loon, F. Brouns, and E. E. Blaak. 2012. Reduced glycaemic and insulinaemic responses following trehalose and isomaltulose ingestion: Implications for postprandial substrate use in impaired glucose-tolerant subjects. British Journal of Nutrition 108 (07):1210–7. doi: 10.1017/S0007114511006714.
- van der Maarel, M. J. E. C., B. van der Veen, J. C. M. Uitdehaag, H. Leemhuis, and L. Dijkhuizen. 2002. Properties and applications of starch-converting enzymes of the alpha-amylase family. Journal of Biotechnology 94 (2):137–55.
- Van der Maarel, M. J. E. C., I. Capron, G. J. W. Euverink, H. T. Bos, T. Kaper, D. J. Binnema, and P. A. M. Steeneken. 2005. A novel thermoreversible gelling product made by enzymatic modification of starch. Starch 57(10):465–72.
- van der Maarel, M. J., and H. Leemhuis. 2013. Starch modification with microbial alpha-glucanotransferase enzymes. Carbohydrate Polymers 93 (1):116–21. doi: 10.1016/j.carbpol.2012.01.065.
- van der Veen, B. A., G. J. W. M. van Alebeek, J. C. M. Uitdehaag, B. W. Dijkstra, and L. Dijkhuizen. 2000. The three transglycosylation reactions catalyzed by cyclodextrin glycosyltransferase from Bacillus circulans (strain 251) proceed via different kinetic mechanisms. European Journal of Biochemistry 267(3):658–65. doi:DOI 10.1046/j.1432-1327.2000.01031.x.
- van Leeuwen, S. S., S. Kralj, I. H. van Geel-Schutten, G. J. Gerwig, L. Dijkhuizen, and J. P. Kamerling. 2008. Structural analysis of the alpha-D-glucan (eps35-5) produced by the Lactobacillus reuteri strain 35-5 glucansucrase GtfA enzyme. Carbohydrate Research 343(7):1251–65. doi: 10.1016/j.carres.2008.01.044.
- Van Tiegham, P. 1878. Sur la gomme de sucrerie. Annales des sciences naturelles. Botanique. Botanique 6 (7):180.
- Vanschoonbeek, K., M. Lansink, K. M. van Laere, J. M. Senden, L. B. Verdijk, and L. J. van Loon. 2009. Slowly digestible carbohydrate sources can be used to attenuate the postprandial glycemic response to the ingestion of diabetes-specific enteral formulas. The Diabetes Educator 35 (4):631–40. doi: 10.1177/0145721709335466.
- Voragen, A. G. J. 1998. Technological aspects of functional food-related carbohydrates. Trends in Food Science and Technology 9 (8-9):328–35. doi:https://doi.org/10.1016/S0924-2244(98)00059-4.
- Vuillemin, M., Claverie, M. Brison, Y. Severac, E. Bondy, P. Morel, S. Monsan, P. Moulis, C. Remaud. S., and M. 2016. Characterization of the first alpha-(1->3) branching sucrases of the GH70 family. Journal of Biological Chemistry 291(14):7687–702. doi: 10.1074/jbc.M115.688044.
- Vujicic-Zagar, A., T. Pijning, S. Kralj, C. A. Lopez, W. Eeuwema, L. Dijkhuizen, and B. W. Dijkstra. 2010. Crystal structure of a 117 kda glucansucrase fragment provides insight into evolution and product specificity of GH70 enzymes. Proceedings of the National Academy of Sciences of the United States of America 107 (50):21406–11. doi: 10.1073/pnas.1007531107.
- Wallander, M., M. Bartnik, S. Efendic, A. Hamsten, K. Malmberg, J. Ohrvik, L. Ryden, A. Silveira, and A. Norhammar. 2005. Beta cell dysfunction in patients with acute myocardial infarction but without previously known type 2 diabetes: a report from the GAMI study. Diabetologia 48 (11):2229–35. doi: 10.1007/s00125-005-1931-z.
- Wattebled, F., J. P. Ral, D. Dauvillee, A. M. Myers, M. G. James, R. Schlichting, C. Giersch, S. G. Ball, and C. d'Hulst. 2003. Sta11, a Chlamydomonas reinhardtii locus required for normal starch granule biogenesis, encodes disproportionating enzyme. Further evidence for a function of alpha-1,4 glucanotransferases during starch granule biosynthesis in green algae. Plant Physiology 132(1):137–45. doi: 10.1104/pp.102.016527.
- Welkie, D. G., B. H. Lee, and L. A. Sherman. 2015. Altering the structure of carbohydrate storage granules in the Cyanobacterium Synechocystis sp. strain PCC 6803 through branching-enzyme truncations. Journal of Bacteriology 198(4):701–10. doi: 10.1128/JB.00830-15 [doi].
- Wolf, B. W., K. A. Garleb, Y. S. Choe, P. M. Humphrey, and K. C. Maki. 2003. Pullulan is a slowly digested carbohydrate in humans. The Journal of Nutrition 133 (4):1051–5.
- Wolf, B., J. Chow, and C.-S. Lai. 2006. Method for using gamma-cyclodextrin to control bood glucose and insulin secretion. WO 2009/004574A2.
- Yamamoto, K., K. Yoshikawa, and S. Okada. 1993. Structure of dextran synthesized by dextrin dextranase from acetobacter-capsulatus ATCC 11894. Bioscience, Biotechnology, and Biochemistry 57(9):1450–3.
- Yamamoto, T., T. Unno, Y. Watanabe, M. Yamamoto, M. Okuyama, H. Mori, S. Chiba, and A. Kimura. 2004. Purification and characterization of acremonium implicatum a-glucosidase having regioselectivity for α-(1,3)- glucosidic linkage. Biochimica et Biophysica Acta 1700(2):189–98.
- Yasuda, E., Takaku, H., and Matsumoto, H. 1986. Production of branched oligosaccharide syrup. JPS61212296 (A).
- Yoo, S. H., M. R. Kweon, M. J. Kim, J. H. Auh, D. S. Jung, J. R. Kim, C. Yook, J. W. Kim, and K. H. Park. 1995. Branched oligosaccharides concentrated by yeast fermentation and effectiveness as a low sweetness humectant. Journal of Food Science 60 (3):516–9. doi:DOI 10.1111/j.1365-2621.1995.tb09816.x.
- Yun, J., M. Lee, and S. Song. 1994. Continuous production of isomaltooligosaccharides from maltose syrup by immobilized cells of permeabilized aureobasidium pullulans. Biotechnology Letters 16(11):1145–50.
- Zhang, G., and B. R. Hamaker. 2009. Slowly digestible starch: Concept, mechanism, and proposed extended glycemic index. Critical Reviews in Food Science and Nutrition 49 (10):852–67. doi: 10.1080/10408390903372466.
- Zhang, G., Z. Ao, and B. R. Hamaker. 2006. Slow digestion property of native cereal starches. Biomacromolecules 7(11):3252–8.
- Zhang, G., Z. Ao, and B. R. Hamaker. 2008. Nutritional property of endosperm starches from maize mutants: a parabolic relationship between slowly digestible starch and amylopectin fine structure. Journal of Agricultural and Food Chemistry 56 (12):4686–94. doi: 10.1021/jf072822m.
- α-cyclodextrin. Csid392705. accessed October. http://www.chemspider.com/Chemical-Structure.392705.html.
- β-cyclodextrin. Csid:10469496. accessed October. http://www.chemspider.com/Chemical-Structure.10469496.html.
- γ-cyclodextrin. Csid:10469499. accessed October. http://www.chemspider.com/Chemical-Structure.10469499.html.