Abstract
Milk fat globule membrane (MFGM) is a complex trilayer structure present in mammalian milk and is mainly composed of phospholipids and proteins (>90%). Many studies revealed MFGM has positive effects on the immune system, brain development, and cognitive function of infants. Probiotics are live microorganisms that have been found to improve mental health and insulin sensitivity, regulate immunity, and prevent allergies. Probiotics are unstable and prone to degradation by environmental, processing, and storage conditions. In this review, the processes used for encapsulation of probiotics particularly the potential of MFGM and its constituents as encapsulating materials for probiotics are described. This study analyzes the importance of MFGM in encapsulating bioactive substances and emphasizes the interaction with probiotics and the gut as well as its resistance to adverse environmental factors in the digestive system when used as a probiotic embedding material. MFGM can enhance the gastric acid resistance and bile resistance of probiotics, mainly manifested in the survival rate of probiotics. Due to the role of digestion, MFGM-coated probiotics can be released in the intestine, and due to the biocompatibility of the membrane, it can promote the binding of probiotics to intestinal epithelial cells, and promote the colonization of some probiotics in the intestine.
Introduction
Milk fat globule membrane (MFGM) is a complex trilayer structure uniquely present in mammalian milk. Many studies have shown that MFGM and its components (particularly phospholipids, sphingomyelin, and specific proteins) play a significant role in infant brain development, cognitive, intestinal, and immune functions (Brink and Lönnerdal Citation2018).
The Food and Agriculture Organization of the United Nations (FAO) and World Health Organization (WHO) defines probiotic as live microorganisms which when administered in adequate amounts confer a health benefit on the host (Hill et al. Citation2014). They are naturally sensitive to heat and moisture which can render them ineffective during processing and prolonged storage. In addition, probiotics are sensitive to acidic gastric environment which might decrease their healthful properties. Processes have been developed to encapsulate probiotics to improve their viability against external environmental conditions and maintain their bioactivity.
At present, research on the use of MFGM for the encapsulation of probiotics is quite limited.
In this review, we summarized the structure, composition, healthful properties, and preparation methods of MFGM. We also discussed about probiotics’ common encapsulation materials and techniques. In addition, we reviewed the properties of MFGM which made it a good candidate as potential encapsulants for probiotics.
Structure, composition, and healthful properties of milk fat globule membrane
Milk is a nutritious food that consists of macronutrients [protein (3.47–4.06 g/100 g of milk), fat (4.33–6.21 g/100g of milk), lactose (4.39–4.83 g/100g of milk)], and micronutrients (vitamins, minerals, inorganic salts and etc.) that are beneficial for human health (Priyashantha et al. Citation2021). In addition, milk also contains high amounts of immunoglobulins and immune factors that play an important role in promoting the development of nervous system, enhancing immunity, and resisting pathogenic microorganisms (Allen and Hampel Citation2020; Brink and Lönnerdal Citation2020; Superti Citation2020).
In milk, lipids are present in the form of milk fat globules (MFG, diameter of 0.2–15 μm with an average diameter of 4 μm) surrounded by a complex trilayer membrane structure with a thickness of 10–50 nm (inner lipid monolayer and an outer lipid bi-layer), called MFGM (Lopez, Cauty, and Guyomarc’h Citation2019). The trilayer MFGM membrane structure is derived from mammary epithelial cells and is synthesized on the endoplasmic reticulum to obtain a polar lipid and protein monolayer vesicles surrounding inner lipid. The vesicles continue to grow as they move toward the cytoplasmic membrane and are squeezed into the alveolar cavity. During the extrusion process, the unilamellar vesicles are coated by cytoplasmic membrane and an additional phospholipid bilayer is obtained, forming a MFGM with trilayer structure vesicle (). MFGM are composed of mainly lipids (20–80%) (Smoczyński, Staniewski, and Kiełczewska Citation2012), proteins (25–60%) (Danthine et al. Citation2000; Singh Citation2006; Walstra et al. Citation2005), and carbohydrates (10%) (Singh Citation2006). Its compositions varies depending on the source of milk, animal feed, lactation period, extraction, and analysis method (Brink and Lönnerdal Citation2020). provides a summary of the health properties of MFGM lipids, proteins, and carbohydrates.
Figure 1. The structure of the milk fat globule membrane (MFGM) (A). molecular structure of MFGM phospholipids (B).
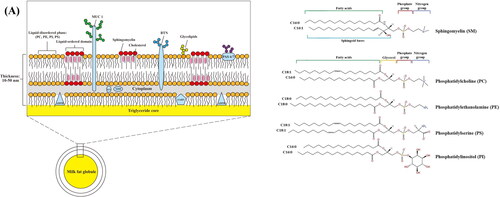
Table 1. Healthful properties of MFGM lipids, proteins, and carbohydrates.
Lipids in MFGM
Polar lipids such as glycerophospholipids [, phosphatidylcholine (PC)], phosphatidylethanolamine (PE), phosphatidylserine (PS) and phosphatidylinositol (PI)], sphingolipids [sphingomyelin (SM)] and glycosphingolipids are the major lipids in MFGM. SM (27.14–34.2% of milk polar lipids), PC (27.35–36.1% of milk polar lipids) and PE (18.9–20.24% of milk polar lipids) are the most abundant polar lipids in MFGM; meanwhile, PS (4.1–8.82% of milk polar lipids) and PI (6.04–6.6% of milk polar lipids) are the least abundant (Bourlieu et al. Citation2020; Wu et al. Citation2020). SM usually combines with cholesterol forming lipid-ordered domains on the surface of the MFGM also known as lipid rafts. Due to its tightly packed structure and higher melting temperature, SM plays a vital role in maintaining stability of the lipid rafts and membrane structure (Murata et al. Citation2022).
provides a comprehensive summary of the composition and healthful properties of MFGM. Lipids in MFGM contribute to development of nervous system and cognitive function. For example, SM helps development of infants language function (Schneider et al. Citation2019), prevents memory loss and treats Alzheimer’s disease (Hashioka et al. Citation2004). Besides that, polar lipids have also been shown to positively associated with cardiometabolic health. SM reduces lipids absorption particularly intestinal cholesterol (Vors et al. Citation2020). Meanwhile, PI effectively inhibits synthesis and storage of cholesteryl esters in blood vessels by altering vascular and cellular cholesterol homeostasis, and promoting cholesterol excretion (Burgess et al. Citation2003). In addition, lipids in MFGM also contributes to intestinal health. SM and PC play a positive role in promoting neonatal intestinal maturation, preventing, and inhibiting intestinal inflammation (Carlson et al. Citation1998; Hertervig et al. Citation1997; Motouri et al. Citation2003).
Proteins in MFGM
MFGM proteins are composed of more than 100 types of proteins of 13–240 kDa (Guerin et al. Citation2019). These proteins are distributed asymmetrically in MFGM. Proteins embedded in or on both sides of MFGM are called integral proteins; proteins bound outside the cell membrane are called peripheral proteins. There are also proteins that are loosely bound to the MFGM. These proteins play a crucial role in exerting physiological function of MFGM.
The main proteins of MFGM are shown in . Mucin 1 (MUC 1) penetrate the outer bilayer membrane, are heavily glycosylated. It is an important component of mucosal defense, and has been shown to inhibit invasion of SARS-CoV-2 (Lai et al. Citation2021). In addition, MUC 1 form a barrier between microorganisms and tissues to protect the digestive tract of newborns from harmful microorganisms (Parker et al. Citation2010). As a transmembrane protein, MUC 1 can participate in intercellular communication (Guo et al. Citation2020).
Xanthine dehydrogenase/oxidase (XDH/XO) are located on the surface of the inner phospholipid monolayer. They have the ability to inhibit bacterial (Escherichia coli, Salmonella enteritidis, and etc.) growth through the formation of reactive oxygen species (e.g., superoxide, hydrogen peroxides, nitric oxide, and peroxynitrite) (Harrison Citation2004; Stevens et al. Citation2000). Butyrophilin (BTN), another glycosylated proteins on MFGM, are the member of the immunoglobulin superfamily (Lu et al. Citation2016). XDH/XO can combine with BTN to inhibit adherence of F4ac-positive E. coli to porcine enterocytes in vitro (Brink and Lönnerdal Citation2020).
Other important proteins located in the inner phospholipid layer are adipophilin (ADPH) and fatty acid binding protein (FABP) which have strong binding affinity to triglycerides. Both ADPH and FABP are not glycosylated. FABP can bind to fatty acids and participate in the dissolution, storage, and transfer of fatty acids between cell membranes and organelles. Similar to FABP, ADPH regulates fat accumulation or hydrolysis processes by modulating lipase affinity to lipid droplets (Bickel, Tansey, and Welte Citation2009). In addition, ADPH and FABP has been shown to have antibacterial effects. ADPH inhibits infection of rotavirus in vitro and FABP have inhibits adherence of bacteria to enterocytes in vitro (Kvistgaard et al. Citation2004; Novakovic et al. Citation2015).
Periodic acid-Schiff base 6 and 7 (PAS 6/7) are located at the surface of the outermost membrane. PAS 6/7 prevents bacterial infection by binding to phospholipids and also participates in formation of mammary gland secretory cells, maintains intestinal epithelial homeostasis, and promotes mucosal healing (Bu et al. Citation2007; Lee et al. Citation2018).
Carbohydrates in MFGM
Carbohydrates in MFGM are present mostly in the form of glycoconjugates such as glycoproteins and glycolipids. One such glycoconjugates is gangliosides which are composed of hydrophilic oligosaccharide chains (monosaccharides of oligosaccharide chains include D-glucose, D-galactose, N-acetylgalactosamine, Fucose, and sialic acid) and lipophilic ceramide which contain a fatty acid chain and a sphingosine. The main gangliosides present in milk are GD3, GM3, and GT3 (63–83% of total gangliosides) (Martín, Martín‐Sosa, and Hueso Citation2001). The function of gangliosides depends greatly on the sialic acid of glycosyl. Sialic acid is extended outside MFGM as receptors that regulate important physiological functions of the body including neurotransmission and leukocyte secretion (Stencel-Baerenwald et al. Citation2014). Gangliosides have been found to promote normal development of the brain specifically within the hippocampus and corpus callosum (Harris et al. Citation2020; Tang et al. Citation2021) and improve learning ability and memory process (Sukhov et al. Citation2020).
Enrichment, purification, and production of MFGM
Due to the healthful properties of MFGM, processes have been developed to either enrich and/or purify MFGM from various dairy sources. shows some of the currently commercially available MFGM materials and the content of the components. HilmarTM 7500, LIPAMINE M 20, LACPRODAN® MFGM-10, SureStartTM Lipid 100 and SureStartTM Lipid 70 are derived from sweet whey, fully natural milk, whey, cream products and cheese whey, respectively. LACPRODAN® MFGM-10 and HilmarTM 7500 are whey protein concentrate with high protein (72–73%) content and approximately 8% of phospholipids. LACPRODAN® MFGM-10 also contains 2% sialic acid. SureStartTM Lipid 100 have low protein (31%) and high carbohydrate (39.8%) content. SureStartTM Lipid 100 contains high levels of phospholipids (7.6%) and gangliosides (0.35%). MFGM products have good emulsification property and high digestibility, which can be applied in a variety of food and nutritional supplements such as infant formula, sports nutrition, and clinical nutrition products.
Table 2. MFGM Brands and components on the market.
To our knowledge, MFGM is mostly by-products of cheese and butter processing. As shown in , centrifugation is one of the most common methods to produce MFGM, which includes centrifugal separation and washing of cream to rupture MFG and collection of MFGM. First, cream is separated by high-speed or ultra-centrifugation (Haddadian et al. Citation2018). The obtained cream is collected and washed with deionized water, sucrose saline solution, isotonic phosphate buffer solution and phosphate saline buffer to remove whey protein, casein and ions (Ye et al. Citation2004). Generally, a maximum of three washing steps is sufficient as MFGM proteins which are loosely bound to the surface of MFGM can be lost easily during washing (Ye et al. Citation2004). Following washing, the obtained MFG is stirred or freeze-dried to release the internal triglycerides. Finally, the MFGM powder is obtained by centrifugation and freeze-drying. This method is simple to operate without the need for sophisticated equipment. However, integrity and purity of the MFGM are dependent on washing solutions, centrifugal force, stirring pH, times, and temperature (Holzmüller and Kulozik Citation2016). Haddadian et al. (Citation2018) elucidated the effects of temperature and pH during cream stirring on the yield and composition of MFGM. Results showed high enrichment of phospholipids (5.86 mg/g fat) can be obtained at a stirring temperature of 10 °C and pH of 5.5.
Membrane separation technology is a new technology for the isolation and purification of MFGM. The commonly used membrane separation methods of MFGM isolation are microfiltration (0.1–1 μm), ultrafiltration (0.001–0.1 μm) and diafiltration. As whey protein (3–6 nm) which is much smaller than MFGM fragments (100–400 nm), it can be directly removed through filtration. Compared with traditional methods, membrane separation technology can effectively increase the relative abundance of MFGM fraction and MFGM protein (e.g., FABP, CD36, MUC 1, MUC 15, XDH/XO, PAS 6/7, and ADPH) and other low molecular weight biological products, and reducing the relative abundance of non MFGM protein (casein: β-CN, α-S2-CN, κ-CN; whey protein: β-Lg, α-La) (Wang et al. Citation2021). However, β-lactoglobulin (small whey protein) binds to MFGM protein through disulfide bonds; hence cannot be easily filtered and retained in the MFGM fragments (Holzmüller and Kulozik Citation2016). Casein has similar particle size (50–600 nm) as MFGM fragments (Barry, Dinan, and Kelly Citation2017). Thus, washing solutions and sodium citrate are often used to separate or coagulate casein molecules prior to ultrafiltration (). Hansen et al. (Citation2020) combined membrane separation technology with traditional centrifugal processing method to obtain MFGM (yield: 2.6 g/L milk, MFGM protein: 58%). The authors also demonstrated gentle pasteurization (72 °C for 15 s) did not change the composition of MFGM materials (Hansen et al. Citation2020).
Enzyme technology is a green production method to further enrich MFGM phospholipids. Pepsin and trypsin hydrolyze proteins (casein, whey protein, and MFGM proteins) into smaller molecular substances which can be separated by membrane filtration technology to enrich the phospholipid fraction (Konrad, Kleinschmidt, and Lorenz Citation2013). Costa et al. (Citation2010) adopted a combination of enzyme and membrane filtration technology (10 kDa molecular mass cutoff, 11.33 m2 total surface area) to produce dairy powder with high MFGM phospholipids (67%) and proteins (74%).
The structure and composition of MFGM is dependent on its enrichment, purification, and isolation technique. Zheng, Jiménez-Flores, and Everett (Citation2014) investigated the structural damage and component loss of MFGM under three separation conditions (M1: 3000 × g for 5 min and 3 washing; M2: 3750 × g for 15 min and 1 washing; M3: 15,000 × g for 20 min and 3 washing, and washing solution was simulated milk ultrafiltrate (pH 6.5)). They found the milder M1 separation technique did not damage the outer phospholipid bilayer of the MFGM, whereas the M3 separation technique was found to be destructive to the outer phospholipid bilayer. In addition, they reported a significant decreased in total polar lipids content of MFGM (especially PE and PC) after the initial centrifugation. The contents of polar lipids following separation were as follows: PE: 26.45–30.52%; PI: 8–8.43%; PS: 13.81–16.78%; PC: 22.46–25.19; SM: 22.89–25.16%; cholesterol: 1.85–3.35 mg/g of fat (Zheng, Jiménez-Flores, and Everett Citation2014).
MFGM phospholipids as encapsulants
Microencapsulation is a technique used to encapsulate bioactive substances into micron or nanoscale particles, which mask any unpleasant odor, provide necessary protection for unstable compounds and control their release in the intestine (Bah, Bilal, and Wang Citation2020). MFGM phospholipids is an excellent material for encapsulating bioactive substances. MFGM phospholipids have higher degree of saturation as compared to plant-based phospholipids; and thus, have lower membrane permeability and higher phase-transition temperature to provide higher resistant against degradation and hydrolysis during storage, and transportation (Abdellatif et al. Citation2020; Yw et al. Citation2021).
MFGM phospholipids can be used as encapsulant for bioactive substances by forming liposomal structures for protection and delivery of sensitive bioactive compounds (). Hydrophilic bioactive substances can be entrapped in the aqueous core; and hydrophobic molecules can be entrapped within the bilayer membrane (Arranz and Corredig Citation2017). summarizes the methods used to form liposomal structures for encapsulation of bioactive substances.
Figure 3. Liposomal structures for encapsulation of bioactive substances (A). TEM analysis of strains grown in AOAC medium (B). (a) L. rhamnosus GG wild-type (LGG WT); (b) welE mutant CMPG5351 (Lebeer et al. Citation2009). TEM analysis of LGG grown after 3 h of incubation (C). (a) in MRS broth; (b) MRS broth with 5 g/L MFGM-10; (c) 0.5% bile in MRS broth; (d) 0.5% bile in MRS broth with 5 g/L of MFGM-10 (Zhang et al. Citation2020). TEM analysis of the effect of MFGM phospholipids in the cell morphology (D). (b) and (d) have MFGM phospholipids adhered to L. delbrueckii and L. plantarum obviously (the arrows indicate accumulation of MFGM phospholipids on the cell wall) (Ortega-Anaya, Marciniak, and Jiménez-Flores Citation2021).
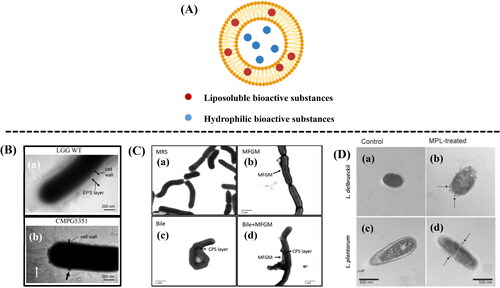
Table 3. Use of MFGM for encapsulation of bioactive substances.
MFGM liposomes have been used to encapsulate vitamins. Jash, Ubeyitogullari, and Rizvi (Citation2020) encapsulated vitamins E (encapsulation efficiency: 77%) and C (encapsulation efficiency: 65%) within MFGM liposomes produced using SC-CO2 assisted Vent-RESS system. They found liposomes produced from MFGM present in the form of ULV; meanwhile, liposomes produced from synthetic sunflower phosphatidylcholine (SFPC) present in a mixture of ULV, MLV, and MVV. Although SFPC liposomes (vitamin E: 88%, vitamin C: 72%) showed higher encapsulation efficiency (EE) than MFGM liposomes, MFGM liposomes exhibited higher thermal stability. MFGM which contains higher saturated fatty acids was less sensitive to oxygen, and was able to retain its morphology after high temperature treatment (Jash, Ubeyitogullari, and Rizvi Citation2020).
Tea polyphenol is a naturally bioactive substance with antioxidant, anti-tumor, bacteriostatic, and lipid-lowering properties. However, the biological activity of tea polyphenols is easily reduced by light, heat, and alkali. Gülseren and Corredig (Citation2013) encapsulated tea polyphenols within MFGM nanoliposomes with an EE of 58%. In addition, the MFGM nanoliposomes demonstrated enhanced physical stability than that of soybean phospholipids liposomes. The authors speculated that the presence of liquid-ordered domains with thicker film-forming characteristics of MFGM materials resulted in the formation of more effective and stable delivery vehicles.
Yw et al. (Citation2020) has synthesized bovine milk phospholipids nanoliposomes for encapsulation of curcumin with an EE of 76%. The curcumin encapsulation rate can be further enhanced (EE: 85%) with addition of cholesterol in the nanoliposomes formulation (Yw et al. Citation2021). We found that curcumin nanoliposomes prepared from bovine milk phospholipids have better stability (pH, oxygen, temperature, metalions, and relative humidity) in comparison to those prepared from krill phospholipids (Yw et al. Citation2020). In addition, the curcumin nanoliposomes can effectively target infectious bacteria that secrete pore-forming toxins such as Staphylococcus aureus by causing the bacterial cell wall to lysis. Transmission electron microscopy shows that the S. aureus treated with curcumin nanoliposomes had irregular edges and obvious cell membrane damage. The curcumin liposomes can be used as preservative to improve storage stability of food such as meat products (Yw et al. Citation2021).
Besides enhancing storage stability, MFGM liposomes can ensure delivery of bioactive substances to intestine for improved bioavailability. Liu et al. (Citation2013) found lactoferrin encapsulated within MFGM phospholipids remained quite stable during in vitro gastric digestion as compared to non-encapsulated lactoferrin. The lactoferrin nanoliposomes was rapidly hydrolyzed by trypsin during in vitro intestinal digestion and the liposome membrane structure was degraded. This indicated that MFGM liposomes can improve the stability of bioactive substances enroute intestine to prevent their degradation in gastric and ensure rapid release under intestinal conditions. Gülseren and Corredig (Citation2013) have evaluated the in-vitro bioavailability of EGCG encapsulated within MFGM liposomes using Caco-2/HT29-MTX co-culture. They found that EGCG encapsulated within MFGM liposomes had higher absorption rate (46 ng/mL) than that encapsulated within soybean phospholipid liposomes (29 ng/mL) (Gülseren and Corredig Citation2013).
Probiotics and encapsulation of probiotics
Probiotics are live microorganisms that have been found to improve mental health, lower blood cholesterol, improve insulin sensitivity, regulate immunity, and prevent allergies of host by regulating human’s intestinal flora (Bermúdez-Humarán et al. Citation2019; Kozakova et al. Citation2016). Due to its healthful properties, probiotics have been incorporated in many food products and supplements (Panghal et al. Citation2018). Some of the major probiotics in human gastrointestinal tract include Lactobacillus and Bifidobacterium species (Yadav, Srikanth, and Yadav Citation2018). In order to provide health promoting properties, ingested probiotics should survive during gastrointestinal transit, attached and colonize in the gastrointestinal epithelium, especially in the colon. Adherence of probiotics to gastrointestinal tract resulting in greater production of mucus (Misra, Mohanty, and Mohapatra Citation2019) to protect gastrointestinal epithelial cells from pathogens (Galdeano et al. Citation2019). In addition, probiotics also compete with harmful pathogenic bacteria for binding to intestinal sites, and prevent adhesion and colonization of harmful bacteria to the intestinal mucosa. Some probiotics also secrete antibacterial substances (i.e. bacteriocin (Ghorani et al. Citation2022), organic acid (Thomas, Mathew, and Rishad Citation2018), and hydrogen peroxide (Abdellatif et al. Citation2020)) to inhibit the growth of harmful bacteria (Abdellatif et al. Citation2020). Besides that, probiotics are able to stimulate inflammatory response and phagocytize harmful bacteria, thereby enhancing human immunity.
Probiotic are unstable and prone to easy degradation by environmental, processing and storage conditions (temperature, pH, oxygen, and moisture) resulting in reduced or loss of their viability in providing healthful properties up on consumption. Their viability can be enhanced by encapsulation technologies such as spray-drying (Akman et al. Citation2021), freeze-drying, extrusion and emulsification (Xu et al. Citation2022). The use of encapsulation can help the bioactive substances to be directly transferred to the target site, such as the intestine, and protect the probiotics from adverse environments (gastric acid and bile, etc.) to improve their bioavailability. In addition, encapsulation have good heat resistance and are friendly to production, transportation, and storage (Kwak Citation2014). Some of the more commonly used encapsulants for probiotic encapsulation include polysaccharides (Akman et al. Citation2021), resins (Akanny et al. Citation2020), and proteins (Ahmad, Mudgil, and Maqsood Citation2019).
Sodium alginate is a commonly used polysaccharide probiotic embedding material. Ji et al. (Citation2019) cross-linked sodium alginate by emulsification to form a gel material for encapsulating Bifidobacterium longum. The prepared alginate microcapsules (Alg-BL) have the advantages of high EE (95 ± 2.5%) and storage stability (25 °C, 180 min). As sodium alginate is a porous material, it is usually used in combination with other materials to enhance the stability of the probiotics. Akman et al. (Citation2021) used sodium alginate and maltodextrin to prepare L. plantarum microcapsules which can be stored at 25 °C for 60 days with a probiotic survival rate of 1.2–2 log CFU/g. Chitosan and agarose have good adhesive properties and can be used for encapsulating probiotics. The NH3 of chitosan will be protonated under acidic conditions which can combine with positively charged substances to form microcapsules. However, the repulsion of NH3 will reduce the firmness of chitosan microcapsules in the stomach. Sodium alginate or gelatin can be used to enhance the strength of chitosan microcapsules.
Eudragit (Eudragit L100 and Eudragit S100) is a compound polymerized by methacrylate monomer and methacrylic acid monomer, which forms insoluble film under acidic conditions of the stomach, effectively protecting the encapsulated probiotics from gastric acid, but it can be dissolved in the pH environment of the intestine. Although Eudragit® S100 has a great capacity of targeted colonic delivery, but it is mainly used as enteric coating material for drugs, and less used for probiotics encapsulation. The reason is that the dissolution characteristics of Eudragit® S100 require the use of organic solvents in the process of microparticles, making it unsuitable for embedding viable probiotics. By adopting the spray-drying operation, Akanny et al. (Citation2020) makes the encapsulation of probiotic is possible by using Eudragit®.
Soybean and milk proteins can also be used for probiotic encapsulation due to its good emulsification, molding, gelling, and water solubility properties (Gharibzahedi and Smith Citation2021). Premjit and Mitra (Citation2021) encapsulated probiotics (Leuconostoc lactis NCDC 200) in the composite matrix of soybean protein isolate (12–15% w/v) and sunflower oil (0–5% w/v) by optimizing the electrospraying operations. The EE was as high as 92.93%, and the viability loss was only 0.663 log CFU/g (Premjit and Mitra Citation2021). Milk proteins (casein, whey protein and MFGM protein) are considered as safe, efficient and nutritious materials to encapsulate probiotics because of their good binding ability to small molecules and pH-responsive gel swelling behavior (Ahmad, Mudgil, and Maqsood Citation2019; Devarajan et al. Citation2022). Ssa et al. (Citation2020) used whey protein isolate (WPI) and gum Arabic as the basic materials and supplemented with phytosterols powder emulsion (LACTEM (10%, emulsifier), phytosterols (10%), oil (11.61%) and hot water (68.39%)) to prepare materials. The encapsulated process of L. plantarum ATCC 8014 is carried out at 25 °C, formed liquid microcapsules by adjusting the pH of the system to 3.75 to stimulate electrostatic combination between WPI and gum Arabic. It is revealed that the microcapsules prepared with (EE: 95.80%) or without (EE: 93.86%) phytosterols powder emulsion had no significant difference in the EE of probiotics (p > 0.05), and the survival rate of L. plantarum encapsulated with two different materials had no significant difference between 31 and 61 days during storage at 4 °C, but there was a significant difference between them (8 log CFU/g) and the free L. plantarum, It shows that WPI and gum Arabic play a vital role in improving the encapsulation rate and bacterial survival rate (Ssa et al. Citation2020).
Most of the probiotic microcapsules are produced using spray-drying (Akman et al. Citation2021), freeze-drying and extrusion technology. However, microcapsules prepared using above methods are prone to reduce survival rate due to the high heat and pressure (spray drying), extreme low temperature (freeze-drying) and the size of microcapsules is too large (extrusion/emulsification (25 μm–2 mm). Electrospinning (Jas et al. Citation2021; Zupančič et al. Citation2019) and self-assembly (Feng et al. Citation2020; Liu et al. Citation2021) approach which enhance the probiotics survival rate during encapsulation is becoming increasingly popular in recent years, the new probiotic embedding technologies, are popular nano-microcapsule preparation technologies in recent years.
MFGM as potential encapsulation agents for probiotics
MFGM which are naturally rich in proteins and phospholipids has good potential for encapsulating probiotics. Research has shown MFGM can resist stomach digestion and enhance bioactivity of the encapsulated bioactive compounds enroute to intestine (Singh Citation2006). Alshehab et al. found that 73% of the curcumin encapsulated within bovine MFG was released in the intestine (Acevedo-Fani, Dave, and Singh Citation2020). Although MFGM materials have properties that make it suitable for encapsulation of probiotics, to our knowledge, no or little research have been conducted to apply MFGM as potential encapsulation agents for probiotics. Herein, we discuss the desirable properties of MFGM as potential encapsulation agent for probiotics.
Adhesion properties of probiotics to MFGM
MFGM has been reported to increase the survival rate of probiotics by forming biofilm that either physically or chemically adhere to the surface of the probiotics. Physical adherence refers to intermolecular forces (e.g., electrostatic interactions, van der Waals, and Lewis acid/base interactions), surface free energy and hydrophobic/hydrophilic interaction between the MFGM proteins and the bacteria’s surface (Brisson et al. Citation2010). MFG is hydrophobic, and thus more hydrophobic probiotics are inclined to show greater affinity toward the MFGM (Brisson et al. Citation2010). Meanwhile, chemical adherence includes MUC 1, phospholipids, proteins, glycospholipids, and glycosides in MFGM to the bacterial cell surface (Burgain et al. Citation2014; Guerin et al. Citation2019). Lopez et al. (Citation2006) reported that lactic acid bacteria (LAB) are more likely to bind with fat-protein interfaces (or MFGM). Over time, bacteria cells may be embedded in MFGM and become part of the phospholipid bilayer. In addition, S-layer proteins of LAB play an important role in the adhesion with MFGM: (1) S-layer proteins are cationic at neutral conditions, allowing them to bind with anionic MFGM-phospholipid head groups (Smit et al. Citation2001). (2) Gene regulation of S-layer protein components of LAB is also one of the factors for the binding of LAB to dairy products containing MFGM (such as raw and pasteurized creams, buttermilk, and buttermilk powder) (Skinner, et al.).
Cleveland (Citation2011) found probiotics have different interactions with MFGM. They found Lactobacillus reuteri, Lacticaseibacillus casei and Lactobacillus acidophilus can effectively bind to MFGM phospholipids. They stained Lactobacillus with acridine orange fluorescence staining and found that Lactobacillus bind to the phospholipid coated polyvinylidene fluoride (PVDF) membrane spots indicating Lactobacillus has selective binding affinity to phospholipids (Cleveland Citation2011). MFGM phospholipids can induce the overexpression of MapA and Cnb genes which produce adhesion factors in probiotics (L. rochei OSU-PECh-37A and OSU-PECh-48), thereby promoting the adhesion of bacteria to MFGM phospholipids (Miyoshi et al. Citation2006). Rocha-Mendoza et al. (Citation2020) also found that Lactobacillus combined with MFGM phospholipids partially up-regulated the relative expression of genes (CmbA, Cnb, Mnb, MapA, MapB, MuB, and Slp) that have been proved to enhance the adhesion of probiotics to the surface of intestinal epithelial cells, which may improve their ability to colonize in the intestine.
Burgain et al. (Citation2014) evaluated the adhesion properties between MFGM and probiotics by using atomic force microscopy (AFM). MFGM were adsorbed on a functionalized mica and an AFM probe to investigate the stress changes of MFGM-functionalized AFM probe on the contact with Lactobacillus rhamnosus. The results have shown that AFM probe coated with MFGM was affected by adhesion during the retraction process, indicating strong adhesion between MFGM and L. rhamnosus GG (LGG) (Burgain et al. Citation2014).
MFGM enhanced acid and bile resistance of probiotics
The pH of human gastric acid is about 1–2 and the concentration of bile is about 0.3%–0.4% (Shori Citation2017; Smith Citation2003). The viability of probiotics in such harsh environments enroute to intestine where they colonize, adhere and grow is related to the bacterial species and surface properties. Suzuki et al. (Citation2014) found extracellular polymeric substance (EPS) produced by bacteria (Lactobacillus brevis and Lactobacillus paracasei strains) helps to improve bile tolerance and viability of the bacteria in host gastrointestinal tract (), increased adhesion to intestinal epithelial cells (IEC) and immunomodulatory capacity (Bengoa et al. Citation2018; Suzuki et al. Citation2014).
Zhang et al. (Citation2020) explored the effects of MFGM on improving the survival rate of LGG in the presence of 0.5% pig bile. They found bile stress upregulates expression of wzb gene which increases secretion of EPS and subsequently promotes adhesion of MFGM to probiotics (). This resulted in the formation of a thicker protective film on the surface of LGG. Hence, higher survival rate of LGG was observed in the cecal contents of mice following ingestion of LGG and MFGM (Zhang et al. Citation2020). In addition, MFGM glycoprotein has also been reported to improve viability of probiotics against stomach conditions enroute intestine (Guerin et al. Citation2019).
The explanation for MFGM improving the survival rate of probiotics in bile can be found in the research of Gallier et al. (Citation2014). Gallier et al. (Citation2014) added bile salts to phospholipids, phospholipids-β-casein or phospholipid-β-lactoglobulin (β-casein and β-lactoglobulin were used to replace the role of MFGM proteins in the MFGM structure). They found bile salts bind to the phospholipid-protein interface to form micelles. β-lactoglobulin has been proven to help maintain the microstructure of phospholipids-β-lactoglobulin membrane under the bile salts damage, avoiding the direct damage of probiotics from bile salts. Additionally, the surface pressure of the phospholipid and phospholipid-protein membrane was also a decisive factor to resist the penetration of bile salts before the addition of bile salts (Gallier et al. Citation2014).
Although the mechanism by which MFGM improves acid and bile resistance of probiotics is limited, the use of MFGM and its components as encapsulation agents has some beneficial values. illustrate our postulation on protection effects of MFGM on viability of probiotics. We postulate EPS will enhance the adhesion of MFGM to probiotics resulted in formation of a thicker protective film on the surface of probiotics and increased viability of the probiotics enroute to intestine.
Figure 4. Schematic diagram of proposed gastrointestinal digestion of MFGM-encapsulated probiotics. Encapsulation of probiotics (e.g., LGG-welE, LGG-spa CBA, L. reuteri, L. delbrueckii, and L. plantarum) by MFGM (e.g., glycoprotein and phospholipid) (A). proposed protection mechanism of probiotics against gastric acid (B). proposed gastrointestinal digestion of MFGM-encapsulated probiotics (C).
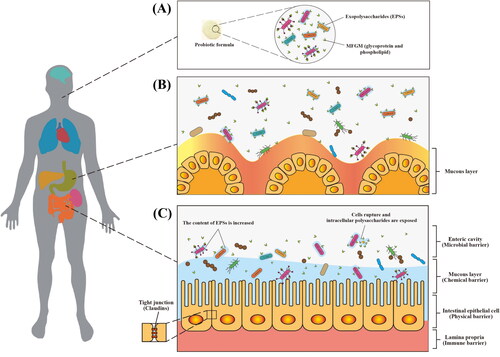
Effects of MFGM on probiotic intestinal adhesion and colonization
Guerin et al. (Citation2018) investigated adhesion and colonization of LGG WT (with pili and EPS), LGG spaCBA (pili-depleted) and LGG welE (EPS-deficient) to Caco-2 TC7 after attaching in vitro to MFGM. A combination of MFGM and LGG was found to reduce bacterial adhesion to Caco-2 TC7 cells and they proposed that MFGM competes with Caco-2 cells to bind wtih LGG. However, this study does not take into consideration of MFGM digestion in the gastrointestinal tract. It remains unclear whether the digested MFGM will cause release of probiotics in the intestinal tract and subsequently colonization of probiotics to the intestinal tract (Guerin et al. Citation2018). Surprisingly, LGG WT and LGG welE increased the number of probiotics adhering to intestinal epithelial cells by the interaction between pili and cells, indicating that the selection of probiotics with pili was also one of the factors that enabled MFGM to be successfully encapsulated. Ortega-Anaya, Marciniak, and Jiménez-Flores (Citation2021) investigated the effects of MFGM metabolism on Lactobacillus (L. acidophilus, L. casei, L. delbrueckii, and L. plantarum) adhesion and colonization to Caco-2/HT-29 co-culture. They found L. acidophilus and L. casei metabolized MFGM phospholipids, and MFGM phospholipids could not be seen attached to the bacterial surface under electron microscope, which improved their adhesion to hydrophilic surfaces such as gold (). On the other hand, L. delbrueckii and L. plantarum do not metabolize MFGM phospholipids, and the adhesion of MFGM phospholipids can be clearly seen under the electron microscope, which irreversibly binds phospholipids to the cell membrane surface, so as to reduce their adhesion to the gold surface (Ortega-Anaya, Marciniak, and Jiménez-Flores Citation2021). They also found Lactobacillus increased surface electronegativity by the metabolization or adhesion of MFGM phospholipids, and the electronegativity is proportional to the number of bacteria adhered to intestinal cells.
Probiotics intestinal adhesion and colonization following interaction with MFGM depends on type of probiotics and the mechanism of interaction between probiotics and MFGM. For example, MFGM administration to newborn piglets during the first week of life was found to promote colonization of bacteria (i.e. Ruminococcaceae) in the gut which can produce short chain fatty acids (SCFA) and promote intestinal barrier function by upregulating genes of tight junctions (E-cadherin, claudin-1, occludin and zonula occludin 1 (ZO-1)), mucins (mucin-13 and mucin-20) and interleukin (IL)-22 (Wu et al. Citation2021). Although the results of this study cannot explain the release of MFGM from the bacterial surface, it can indicate that MFGM has potential functional value in regulating the gut microbiota in early life and promoting the colonization of probiotics in the gut.
Conclusion
Current research has shown MFGM (MFGM phospholipids and glycoproteins) can enhanced viability of probiotics enroute to intestine and promote colonization of probiotics in the intestine. These properties indicate that MFGM has great potential to be used as encapsulant of probiotics. Probiotics encapsulated within the MFGM will demonstrate combined beneficial healthful properties of both function ingredients. They can be expected to have wide application in food industry.
Disclosure statement
No potential conflict of interest was reported by the authors.
Additional information
Funding
References
- Abdellatif, B., C. Mcveigh, G. Bendriss, and A. Chaari. 2020. The promising role of probiotics in managing the altered gut in autism spectrum disorders. International Journal of Molecular Sciences 21 (11):4159. doi: 10.3390/IJMS21114159.
- Acevedo-Fani, A., A. Dave, and H. Singh. 2020. Nature-assembled structures for delivery of bioactive compounds and their potential in functional foods. Frontiers in Chemistry 8: 564021. doi: 10.3389/fchem.2020.564021.
- Ahmad, M., P. Mudgil, and S. Maqsood. 2019. Camel whey protein microparticles for safe and efficient delivery of novel camel milk derived probiotics. LWT 108:81–8. doi: 10.1016/j.lwt.2019.03.008.
- Akanny, E., S. Bourgeois, A. Bonhommé, C. Commun, A. Doleans-Jordheim, F. Bessueille, and C. Bordes. 2020. Development of enteric polymer-based microspheres by spray-drying for colonic delivery of Lactobacillus rhamnosus GG. International Journal of Pharmaceutics 584:119414. doi: 10.1016/j.ijpharm.2020.119414.
- Akman, P. K., F. Bozkurt, K. Dogan, F. Tornuk, and F. Tamturk. 2021. Fabrication and characterization of probiotic Lactobacillus plantarum loaded sodium alginate edible films. Journal of Food Measurement and Characterization 15 (1):84–92. doi: 10.1007/s11694-020-00619-6.
- Allen, L. H., and D. Hampel. 2020. Human milk as the first source of micronutrients. Global Landscape of Nutrition Challenges in Infants and Children 93:67–76. doi: 10.1159/000503359.
- Al-Shehri, S. S., J. A. Duley, and N. Bansal. 2020. Xanthine oxidase-lactoperoxidase system and innate immunity: Biochemical actions and physiological roles. Redox Biology 34:101524. doi: 10.1016/j.redox.2020.101524.
- Anand, B. S., J. J. Romero, S. K. Sanduja, and L. M. Lichtenberger. 1999. Phospholipid association reduces the gastric mucosal toxicity of aspirin in human subjects. The American Journal of Gastroenterology 94 (7):1818–22. doi: 10.1016/S0002-9270(99)00267-1.
- Arranz, E., and M. Corredig. 2017. Invited review: Milk phospholipid vesicles, their colloidal properties, and potential as delivery vehicles for bioactive molecules. Journal of Dairy Science 100 (6):4213–22. doi: 10.3168/jds.2016-12236.
- Bah, M. G., H. M. Bilal, and J. Wang. 2020. Fabrication and application of complex microcapsules: A review. Soft Matter 16 (3):570–90. doi: 10.1039/C9SM01634A.
- Barry, K. M., T. G. Dinan, and P. M. Kelly. 2017. Selective enrichment of dairy phospholipids in a buttermilk substrate through investigation of enzymatic hydrolysis of milk proteins in conjunction with ultrafiltration. International Dairy Journal 68:80–7. doi: 10.1016/j.idairyj.2016.12.016.
- Bengoa, A. A., M. G. Llamas, C. Iraporda, M. T. Dueñas, A. G. Abraham, and G. L. Garrote. 2018. Impact of growth temperature on exopolysaccharide production and probiotic properties of Lactobacillus paracasei strains isolated from kefir grains. Food Microbiology 69:212–8. doi: 10.1016/j.fm.2017.08.012.
- Bermúdez-Humarán, L. G., E. Salinas, G. G. Ortiz, L. J. Ramirez-Jirano, J. A. Morales, and O. K. Bitzer-Quintero. 2019. From probiotics to psychobiotics: Live beneficial bacteria which act on the brain-gut axis. Nutrients 11 (4):890. doi: 10.3390/nu11040890.
- Bickel, P. E., J. T. Tansey, and M. A. Welte. 2009. PAT proteins, an ancient family of lipid droplet proteins that regulate cellular lipid stores. Biochimica et Biophysica Acta 1791 (6):419–40. doi: 10.1016/j.bbalip.2009.04.002.
- Bint E Naser, S. F., H. Su, H.-Y. Liu, Z. A. Manzer, Z. Chao, A. Roy, A.-M. Pappa, A. Salleo, R. M. Owens, and S. Daniel. 2021. Detection of ganglioside-specific toxin binding with biomembrane-based bioelectronic sensors. ACS Applied Bio Materials 4 (11):7942–50. doi: 10.1021/acsabm.1c00878.
- Bourlieu, C., W. Mahdoueni, G. Paboeuf, E. Gicquel, O. Ménard, S. Pezennec, S. Bouhallab, A. Deglaire, D. Dupont, F. Carrière, et al. 2020. Physico-chemical behaviors of human and bovine milk membrane extracts and their influence on gastric lipase adsorption. Biochimie 169:95–105. doi: 10.1016/j.biochi.2019.12.003.
- Brink, L. R., and B. Lönnerdal. 2018. The role of milk fat globule membranes in behavior and cognitive function using a suckling rat pup supplementation model. The Journal of Nutritional Biochemistry 58:131–7. doi: 10.1016/j.jnutbio.2018.05.004.
- Brink, L. R., and B. Lönnerdal. 2020. Milk fat globule membrane: The role of its various components in infant health and development. The Journal of Nutritional Biochemistry 85:108465. doi: 10.1016/j.jnutbio.2020.108465.
- Brisson, G., H. F. Payken, J. P. Sharpe, and R. Jime?Nez-Flores. 2010. Characterization of Lactobacillus reuteri interaction with milk fat globule membrane components in dairy products. Journal of Agricultural and Food Chemistry 58 (9):5612–9. doi: 10.1021/jf904381s.
- Bu, H. F., X. L. Zuo, X. Wang, M. A. Ensslin, V. Koti, W. Hsueh, A. S. Raymond, B. D. Shur, and X. D. Tan. 2007. Milk fat globule-EGF factor 8/lactadherin plays a crucial role in maintenance and repair of murine intestinal epithelium. The Journal of Clinical Investigation 117 (12):3673–83. doi: 10.1172/JCI31841.
- Burgain, J., J. Scher, G. Francius, F. Borges, M. Corgneau, A. M. Revol-Junelles, C. Cailliez-Grimal, and C. Gaiani. 2014. Lactic acid bacteria in dairy food: Surface characterization and interactions with food matrix components. Advances in Colloid and Interface Science 213:21–35. doi: 10.1016/j.cis.2014.09.005.
- Burgess, J. W., J. Boucher, T. A. Neville, P. Rouillard, C. Stamler, S. Zachariah, and D. L. Sparks. 2003. Phosphatidylinositol promotes cholesterol transport and excretion. Journal of Lipid Research 44 (7):1355–63. doi: 10.1194/jlr.M300062-JLR200.
- Carlson, S. E., M. B. Montalto, D. L. Ponder, S. H. Werkman, and S. B. Korones. 1998. Lower Incidence of necrotizing enterocolitis in infants fed a preterm formula with egg phospholipids. Pediatric Research 44 (4):491–8. doi: 10.1203/00006450-199810000-00005.
- Cleveland, M. A. 2011. The development of a novel technique to evaluate binding between probiotic bacteria and phospholipids, and the creation of a dairy-based food product rich in milk bioactives. doi: 10.15368/theses.2011.27.
- Cohen, B.-C., C. Raz, A. Shamay, and N. Argov-Argaman. 2017. Lipid droplet fusion in mammary epithelial cells is regulated by phosphatidylethanolamine metabolism. Journal of Mammary Gland Biology and Neoplasia 22 (4):235–49. doi: 10.1007/s10911-017-9386-7.
- Costa, M. R., X. E. Elias-Argote, R. Jiménez-Flores, and M. L. Gigante. 2010. Use of ultrafiltration and supercritical fluid extraction to obtain a whey buttermilk powder enriched in milk fat globule membrane phospholipids. International Dairy Journal 20 (9):598–602. doi: 10.1016/j.idairyj.2010.03.006.
- Danthine, S., C. Blecker, M. Paquot, N. Innocente, and C. Deroanne. 2000. Évolution des connaissances sur la membrane du globule gras du lait: Synthèse bibliographique. Le Lait 80 (2):209–22. doi: 10.1051/lait:2000120.
- Devarajan, A., P. Mudgil, F. Aldhaheri, F. Hamed, S. Dhital, and S. Maqsood. 2022. Camel milk-derived probiotic strains encapsulated in camel casein and gelatin complex microcapsules: Stability against thermal challenge and simulated gastrointestinal digestion conditions. Journal of Dairy Science 105 (3):1862–77. doi: 10.3168/jds.2021-20745.
- Dewettinck, K., R. Rombaut, N. Thienpont, T. T. Le, K. Messens, and J. V. Camp. 2008. Nutritional and technological aspects of milk fat globule membrane material. International Dairy Journal 18 (5):436–57. doi: 10.1016/j.idairyj.2007.10.014.
- Dhanisha, S. S., C. Guruvayoorappan, S. Drishya, and P. Abeesh. 2018. Mucins: Structural diversity, biosynthesis, its role in pathogenesis and as possible therapeutic targets. Critical Reviews in Oncology/Hematology 122:98–122. doi: 10.1016/j.critrevonc.2017.12.006.
- Feng, P., Z. Cao, X. Wang, J. Li, and J. Liu. 2020. On-demand bacterial reactivation by restraining within a triggerable nanocoating. Advanced Materials (Deerfield Beach, Fla.) 32 (34): E 2002406. doi: 10.1002/adma.202002406.
- Fernández-Arroyo, S., A. Hernández-Aguilera, M. A. de Vries, B. Burggraaf, E. van der Zwan, N. Pouw, J. Joven, and M. Castro Cabezas. 2019. Effect of Vitamin D3 on the postprandial lipid profile in obese patients: A non-targeted lipidomics study. Nutrients 11 (5):1194. doi: 10.3390/nu11051194.
- Fong, B. Y., C. S. Norris, and A. Macgibbon. 2007. Protein and lipid composition of bovine milk-fat-globule membrane. International Dairy Journal 17 (4):275–88. doi: 10.1016/j.idairyj.2006.05.004.
- Galdeano, C. M., S. I. Cazorla, J. M. L. Dumit, E. Vélez, and G. Perdigón. 2019. Beneficial effects of probiotic consumption on the immune system. Annals of Nutrition & Metabolism 74 (2):115–24. doi: 10.1159/000496426.
- Gallier, S., E. Shaw, A. Laubscher, D. Gragson, H. Singh, and R. Jiménez-Flores. 2014. Adsorption of bile salts to milk phospholipid and phospholipid–protein monolayers. Journal of Agricultural and Food Chemistry 62 (6):1363–72. doi: 10.1021/jf404448d.
- Gharibzahedi, S. M. T., and B. Smith. 2021. Legume proteins are smart carriers to encapsulate hydrophilic and hydrophobic bioactive compounds and probiotic bacteria: A review. Comprehensive Reviews in Food Science and Food Safety 20 (2):1250–79. doi: 10.1111/1541-4337.12699.
- Ghorani, M. 2022. Antiviral Effects of Probiotic Metabolites. Iranian Journal of Medical Microbiology 16 (2):83–97. doi: 10.30699/ijmm.16.2.83.
- Guerin, J., J. Burgain, F. Gomand, J. Scher, and C. Gaiani. 2019. Milk fat globule membrane glycoproteins: Valuable ingredients for lactic acid bacteria encapsulation? Critical Reviews in Food Science and Nutrition 59 (4):639–51. doi: 10.1080/10408398.2017.1386158.
- Guerin, J., C. Soligot, J. Burgain, M. Huguet, G. Francius, S. El-Kirat-Chatel, F. Gomand, S. Lebeer, Y. Le Roux, F. Borges, et al. 2018. Adhesive interactions between milk fat globule membrane and Lactobacillus rhamnosus GG inhibit bacterial attachment to Caco-2 TC7 intestinal cell. Colloids and Surfaces. B, Biointerfaces 167:44–53. doi: 10.1016/j.colsurfb.2018.03.044.
- Gülseren, I., and M. Corredig. 2013. Storage stability and physical characteristics of tea-polyphenol-bearing nanoliposomes prepared with milk fat globule membrane phospholipids. Journal of Agricultural and Food Chemistry 61 (13):3242–51. doi: 10.1021/jf3045439.
- Guo, Y., J. Tao, Y. Li, Y. Feng, H. Ju, Z. Wang, and L. Ding. 2020. Quantitative localized analysis reveals distinct exosomal protein-specific glycosignatures: Implications in cancer cell subtyping, exosome biogenesis, and function. Journal of the American Chemical Society 142 (16):7404–12. doi: 10.1021/jacs.9b12182.
- Haddadian, Z., G. T. Eyres, P. Bremer, and D. W. Everett. 2018. Polar lipid composition of the milk fat globule membrane in buttermilk made using various cream churning conditions or isolated from commercial samples. International Dairy Journal 81:138–42. doi: 10.1016/j.idairyj.2018.01.012.
- Han, L., M. Zhang, Z. Xing, D. N. Coleman, Y. Liang, J. J. Loor, and G. Yang. 2020. Knockout of butyrophilin subfamily 1 member A1 (BTN1A1) alters lipid droplet formation and phospholipid composition in bovine mammary epithelial cells. Journal of Animal Science and Biotechnology 11 (1):72. doi: 10.1186/s40104-020-00479-6.
- Hansen, S. F., S. A. Hogan, J. Tobin, J. T. Rasmussen, L. B. Larsen, and L. Wiking. 2020. Microfiltration of raw milk for production of high-purity milk fat globule membrane material. Journal of Food Engineering 276:109887. doi: 10.1016/j.jfoodeng.2019.109887.
- Harris, A., A. Roseborough, R. Mor, K. K.-C. Yeung, and S. N. Whitehead. 2020. Ganglioside detection from formalin-fixed human brain tissue utilizing MALDI imaging mass spectrometry. Journal of the American Society for Mass Spectrometry 31 (3):479–87. doi: 10.1021/jasms.9b00110.
- Harrison, R. 2004. Physiological roles of xanthine oxidoreductase. Drug Metabolism Reviews 36 (2):363–75. doi: 10.1081/DMR-120037569.
- Hartmann, P., A. Szabó, G. Eros, D. Gurabi, G. Horváth, I. Németh, M. Ghyczy, and M. Boros. 2009. Anti-inflammatory effects of phosphatidylcholine in neutrophil leukocyte-dependent acute arthritis in rats. European Journal of Pharmacology 622 (1-3):58–64. doi: 10.1016/j.ejphar.2009.09.012.
- Hashioka, S., A. Monji, Y. Han, H. Nakanishi, and S. Kanba. 2004. Potentiality of phosphatidylserine (PS)-liposomes for treatment of Alzheimer’s disease. Journal of Neuroimmunology 154:119.
- Hertervig, E., A. Nilsson, L. Nyberg, and R. D. Duan. 1997. Alkaline sphingomyelinase activity is decreased in human colorectal carcinoma. Cancer 79 (3):448–53. doi: 10.1016/j.foodres.2018.11.040.
- Hill, C., F. Guarner, G. Reid, G. R. Gibson, D. J. Merenstein, B. Pot, L. Morelli, R. B. Canani, H. J. Flint, S. Salminen, et al. 2014. Expert consensus document: The International Scientific Association for Probiotics and Prebiotics consensus statement on the scope and appropriate use of the term probiotic. Nature Reviews. Gastroenterology & Hepatology 11 (8):506–14. doi: 10.1038/nrgastro.2014.66.
- Holzmüller, W., and U. Kulozik. 2016. Isolation of milk fat globule membrane (MFGM) material by coagulation and diafiltration of buttermilk. International Dairy Journal 63:88–91. doi: 10.1016/j.idairyj.2016.08.002.
- Hussain, G., J. Wang, A. Rasul, H. Anwar, A. Imran, M. Qasim, S. Zafar, S. K. S. Kamran, A. Razzaq, N. Aziz, et al. 2019. Role of cholesterol and sphingolipids in brain development and neurological diseases. Lipids in Health and Disease 18 (1):26. doi: 10.1186/s12944-019-0965-z.
- Jas, A., A. Prdg, B. Gr, B. Gaa, and A. Nm. 2021. Immobilization of vaginal Lactobacillus in polymeric nanofibers for its incorporation in vaginal probiotic products. European Journal of Pharmaceutical Sciences 156:105563. doi: 10.1016/j.ejps.2020.105563.
- Jash, A., A. Ubeyitogullari, and S. S. Rizvi. 2020. Synthesis of multivitamin-loaded heat stable liposomes from milk fat globule membrane phospholipids by using a supercritical-CO2 based system. Green Chemistry 22 (16):5345–56. doi: 10.1039/D0GC01674H.
- Ji, R., J. Wu, J. Zhang, T. Wang, X. Zhang, L. Shao, D. Chen, and J. Wang. 2019. Extending viability of Bifidobacterium longum in chitosan-coated alginate microcapsules using emulsification and internal gelation encapsulation technology. Frontiers in Microbiology 10:1389. doi: 10.3389/fmicb.2019.01389.
- Jin, H. H., Q. Lu, and J. G. Jiang. 2016. Curcumin liposomes prepared with milk fat globule membrane phospholipids and soybean lecithin. Journal of Dairy Science 99 (3):1780–90. doi: 10.3168/jds.2015-10391.
- Kidd, P. 2000. Dietary phospholipids as anti-aging nutraceuticals. Anti-Aging Medical Therapeutics 4:282–300. doi: 10.1007/0-387-28813-9_4.
- Kimura, A. K., and H.-Y. Kim. 2013. Phosphatidylserine synthase 2: High efficiency for synthesizing phosphatidylserine containing docosahexaenoic acid. Journal of Lipid Research 54 (1):214–22. doi: 10.1194/jlr.M031989.
- Kingsley, D. M. 2006. Effects of phosphatidylserine supplementation on exercising humans. Sports Medicine (Auckland, N.Z.) 36 (8):657–69. doi: 10.2165/00007256-200636080-00003.
- Koletzko, B. 2016. Human milk lipids. Annals of Nutrition & Metabolism 69 (Suppl. 2):28–40. doi: 10.1159/000452819.
- Konrad, G., T. Kleinschmidt, and C. Lorenz. 2013. Ultrafiltration of whey buttermilk to obtain a phospholipid concentrate. International Dairy Journal 30 (1):39–44. doi: 10.1016/j.idairyj.2012.11.007.
- Kozakova, H., M. Schwarzer, L. Tuckova, D. Srutkova, E. Czarnowska, I. Rosiak, T. Hudcovic, I. Schabussova, P. Hermanova, Z. Zakostelska, et al. 2016. Colonization of germ-free mice with a mixture of three lactobacillus strains enhances the integrity of gut mucosa and ameliorates allergic sensitization. Cellular & Molecular Immunology 13 (2):251–62. doi: 10.1038/cmi.2015.09.
- Kvistgaard, A., L. Pallesen, C. Arias, S. Lopez, T. Petersen, C. Heegaard, and J. Rasmussen. 2004. Inhibitory effects of human and bovine milk constituents on rotavirus infections. Journal of Dairy Science 87 (12):4088–96. doi: 10.3168/jds.S0022-0302(04)73551-1.
- Kwak, H. S. 2014. Overview of nano‐and microencapsulation for foods. Nano‐and microencapsulation for foods, 1–14. doi: 10.1002/9781118292327.ch1.
- Lai, X., Y. Yu, W. Xian, F. Ye, X. Ju, Y. Luo, H. Dong, Y. Zhou, W. Tan, and H. Zhuang. 2021. Inhibition of SAR S-CoV-2 infection and replication by lactoferrin, MUC1 and α-lactalbumin identified in human breastmilk. bioRxiv doi: 10.1101/2021.10.29.466402.
- Lastra, G., C. Manrique, G. Jia, A. R. Aroor, M. R. Hayden, B. J. Barron, B. Niles, J. Padilla, and J. R. Sowers. 2017. Xanthine oxidase inhibition protects against Western diet-induced aortic stiffness and impaired vasorelaxation in female mice. American Journal of Physiology. Regulatory, Integrative and Comparative Physiology 313 (2):R67–R77. doi: 10.1152/ajpregu.00483.2016.
- Lebeer, S., T. L. Verhoeven, G. Francius, G. Schoofs, I. Lambrichts, Y. F. Dufrêne, J. Vanderleyden, and S. D. Keersmaecker. 2009. Identification of a gene cluster for the biosynthesis of a long, galactose-rich exopolysaccharide in Lactobacillus rhamnosus GG and functional analysis of the priming glycosyltransferase. Applied and Environmental Microbiology 75 (11):3554–63. doi: 10.1128/AEM.02919-08.
- Lee, H., E. Padhi, Y. Hasegawa, J. Larke, M. Parenti, A. Wang, O. Hernell, B. Lönnerdal, and C. Slupsky. 2018. Compositional dynamics of the milk fat globule and its role in infant development. Frontiers in Pediatrics 6:313. doi: 10.3389/fped.2018.00313.
- Li, Y., E. Arranz, A. Guri, and M. Corredig. 2017. Mucus interactions with liposomes encapsulating bioactives: Interfacial tensiometry and cellular uptake on Caco-2 and cocultures of Caco-2/HT29-MTX. Food Research International (Ottawa, Ont.) 92:128–37. doi: 10.1016/j.foodres.2016.12.010.
- Li, T., M. Du, H. Wang, and X. Mao. 2020. Milk fat globule membrane and its component phosphatidylcholine induce adipose browning both in vivo and in vitro. The Journal of Nutritional Biochemistry 81:108372. doi: 10.1016/j.jnutbio.2020.108372.
- Li, Z., A. T. Paulson, and T. A. Gill. 2015. Encapsulation of bioactive salmon protein hydrolysates with chitosan-coated liposomes. Journal of Functional Foods 19:733–43. doi: 10.1016/j.jff.2015.09.058.
- Liu, Z., B. Cocks, A. Patel, A. Oglobline, G. Richardson, and S. Rochfort. 2016. Identification and quantification of phosphatidylinositol in infant formulas by liquid chromatography–mass spectrometry. Food Chemistry 205:178–86. doi: 10.1016/j.foodchem.2016.02.163.
- Liu, J., W. Li, Y. Wang, Y. Ding, A. Lee, and Q. Hu. 2021. Biomaterials coating for on-demand bacteria delivery: Selective release, adhesion, and detachment. Nano Today. 41:101291. doi: 10.1016/j.nantod.2021.101291.
- Liu, W., A. Ye, W. Liu, C. Liu, and H. Singh. 2013. Stability during in vitro digestion of lactoferrin-loaded liposomes prepared from milk fat globule membrane-derived phospholipids. Journal of Dairy Science 96 (4):2061–70. doi: 10.3168/jds.2012-6072.
- Lopez, C., C. Cauty, and F. Guyomarc’h. 2019. Unraveling the complexity of milk fat globules to tailor bioinspired emulsions providing health benefits: The key role played by the biological membrane. European Journal of Lipid Science and Technology 121 (1):1800201. doi: 10.1002/ejlt.201800201.
- Lopez, C., M. B. Maillard, V. Briard-Bion, B. Camier, and J. A. Hannon. 2006. Lipolysis during ripening of Emmental cheese considering organization of fat and preferential localization of bacteria. Journal of Agricultural and Food Chemistry 54 (16):5855–67. doi: 10.1021/jf060214l.
- Lu, J., X. Wang, W. Zhang, L. Liu, X. Pang, S. Zhang, and J. Lv. 2016. Comparative proteomics of milk fat globule membrane in different species reveals variations in lactation and nutrition. Food Chemistry 196:665–72. doi: 10.1016/j.foodchem.2015.10.005.
- Manoni, M., C. Di Lorenzo, M. Ottoboni, M. Tretola, and L. Pinotti. 2020. Comparative proteomics of milk fat globule membrane (MFGM) proteome across species and lactation stages and the potentials of MFGM fractions in infant formula preparation. Foods (Basel, Switzerland) 9 (9):1251. doi: 10.3390/foods9091251.
- Martin, H. M., K. P. Moore, E. Bosmans, S. Davies, A. K. Burroughs, A. P. Dhillon, D. Tosh, and R. Harrison. 2004. Xanthine oxidoreductase is present in bile ducts of normal and cirrhotic liver. Free Radical Biology & Medicine 37 (8):1214–23. doi: 10.1016/j.freeradbiomed.2004.06.045.
- Martín, M. J., S. Martín‐Sosa, and P. Hueso. 2001. Bovine milk gangliosides: Changes in ceramide moiety with stage of lactation. Lipids 36 (3):291–8. doi: 10.1007/s11745-001-0720-x.
- Misra, S., D. Mohanty, and S. Mohapatra. 2019. Applications of Probiotics as a Functional Ingredient in Food and Gut Health. Journal of Food and Nutrition Research 7 (3):213–23. doi: 10.12691/jfnr-7-3-6.
- Miyoshi, Y., S. Okada, U. Tai, and E. Satoh. 2006. A mucus adhesion promoting protein, MapA, mediates the adhesion of Lactobacillus reuteri to Caco-2 human intestinal epithelial cells. Bioscience, Biotechnology, and Biochemistry 70 (7):1622–8. doi: 10.1271/bbb.50688.
- Motouri, M., H. Matsuyama, J. I. Yamamura, M. Tanaka, S. Aoe, T. Iwanaga, and H. Kawakami. 2003. Milk sphingomyelin accelerates enzymatic and morphological maturation of the intestine in artificially reared rats. Journal of Pediatric Gastroenterology and Nutrition 36 (2):241–7. doi: 10.1097/00005176-200302000-00016.
- Moukarzel, S., R. A. Dyer, C. Garcia, A. M. Wiedeman, G. Boyce, J. Weinberg, B. O. Keller, R. Elango, and S. M. Innis. 2018. Milk fat globule membrane supplementation in formula-fed rat pups improves reflex development and may alter brain lipid composition. Scientific Reports 8 (1):15277. doi: 10.1038/s41598-018-33603-8.
- Murata, M., N. Matsumori, M. Kinoshita, and E. London. 2022. Molecular substructure of the liquid-ordered phase formed by sphingomyelin and cholesterol: Sphingomyelin clusters forming nano-subdomains are a characteristic feature. Biophysical Reviews 14 (3):655–78. doi: 10.1007/s12551-022-00967-1.
- Norris, G. H., C. M. Porter, C. Jiang, C. L. Millar, and C. N. Blesso. 2017. Dietary sphingomyelin attenuates hepatic steatosis and adipose tissue inflammation in high-fat-diet-induced obese mice. The Journal of Nutritional Biochemistry 40:36–43. doi: 10.1016/j.jnutbio.2016.09.017.
- Novakovic, P., Y. Y. Huang, B. Lockerbie, F. Shahriar, J. Kelly, J. R. Gordon, D. M. Middleton, M. E. Loewen, B. A. Kidney, and E. Simko. 2015. Identification of Escherichia coli F4ac-binding proteins in porcine milk fat globule membrane. Canadian Journal of Veterinary Research 79 (2):120–8.
- Ortega-Anaya, J., and R. Jiménez-Flores. 2019. Symposium review: The relevance of bovine milk phospholipids in human nutrition-Evidence of the effect on infant gut and brain development. Journal of Dairy Science 102 (3):2738–48. doi: 10.3168/jds.2018-15342.
- Ortega-Anaya, J., A. Marciniak, and R. Jiménez-Flores. 2021. Milk fat globule membrane phospholipids modify adhesion of Lactobacillus to mucus-producing Caco-2/Goblet cells by altering the cell envelope. Food Research International (Ottawa, Ont.) 146:110471. doi: 10.1016/j.foodres.2021.110471.
- Panghal, A., S. Janghu, K. Virkar, Y. Gat, V. Kumar, and N. Chhikara. 2018. Potential non-dairy probiotic products–A healthy approach. Food Bioscience 21:80–9. doi: 10.1016/j.fbio.2017.12.003.
- Parker, P., L. Sando, R. Pearson, K. Kongsuwan, R. L. Tellam, and S. Smith. 2010. Bovine Muc1 inhibits binding of enteric bacteria to Caco-2 cells. Glycoconjugate Journal 27 (1):89–97. doi: 10.1007/s10719-009-9269-2.
- Premjit, Y., and J. Mitra. 2021. Optimization of electrospray-assisted microencapsulation of probiotics (Leuconostoc lactis) in soy protein isolate-oil particles using Box-Behnken experimental design. Food and Bioprocess Technology 14 (9):1712–29. doi: 10.1007/s11947-021-02670-7.
- Priyashantha, H., Å. Lundh, A. Höjer, G. Bernes, D. Nilsson, M. Hetta, K. H. Saedén, A. H. Gustafsson, and M. Johansson. 2021. Composition and properties of bovine milk: A study from dairy farms in northern Sweden; Part I. Effect of dairy farming system. Journal of Dairy Science 104 (8):8582–94. doi: 10.3168/jds.2020-19650.
- Rocha-Mendoza, D., E. Kosmerl, G. Miyagusuku-Cruzado, M. M. Giusti, R. Jiménez-Flores, and I. García-Cano. 2020. Growth of lactic acid bacteria in milk phospholipids enhances their adhesion to Caco-2 cells. Journal of Dairy Science 103 (9):7707–18. doi: 10.3168/jds.2020-18271.
- Russell, A., A. Laubscher, R. Jiménez-Flores, and L. H. Laiho. 2010. Investigating the protective properties of milk phospholipids against ultraviolet light exposure in a skin equivalent model. Proceedings of SPIE – The International Society for Optical Engineering, 7569. doi: 10.1117/12.845803.
- Schneider, N., J. Hauser, M. Oliveira, E. Cazaubon, S. C. Mottaz, B. V. O’Neill, P. Steiner, and S. C. L. Deoni. 2019. Sphingomyelin in brain and cognitive development: Preliminary data. eNeuro 6 (4):ENEURO.0421-18.2019. doi: 10.1523/ENEURO.0421-18.2019.
- Shori, A. B. 2017. Microencapsulation improved probiotics survival during gastric transit. HAYATI Journal of Biosciences 24 (1):1–5. doi: 10.1016/j.hjb.2016.12.008.
- Singh, H. 2006. The milk fat globule membrane-A biophysical system for food applications. Current Opinion in Colloid & Interface Science 11 (2-3):154–63. doi: 10.1016/j.cocis.2005.11.002.
- Skinner, G., N. Reddy, and B. Parisi. Dairy foods: milk, dairy food chemistry and microbiology.
- Smit, E., F. Oling, R. Demel, B. Martinez, and P. H. Pouwels. 2001. The S-layer protein of Lactobacillus acidophilus ATCC 4356: Identification and characterisation of domains responsible for S-protein assembly and cell wall binding. Journal of Molecular Biology 305 (2):245–57. doi: 10.1006/jmbi.2000.4258.
- Smith, J. L. 2003. The role of gastric acid in preventing foodborne disease and how bacteria overcome acid conditions. Journal of Food Protection 66 (7):1292–303. doi: 10.4315/0362-028X-66.7.1292.
- Smoczyński, M., B. Staniewski, and K. Kiełczewska. 2012. Composition and structure of the bovine milk fat globule membrane—some nutritional and technological implications. Food Reviews International 28 (2):188–202. doi: 10.1080/87559129.2011.595024.
- Ssa, B., B. Rb, B. Ma, B. Ha, and B. Sa. 2020. Use of whey protein isolate and gum Arabic for the co-encapsulation of probiotic Lactobacillus plantarum and phytosterols by complex coacervation: Enhanced viability of probiotic in Iranian white cheese. Food Hydrocolloids. 113, 106496. doi: 10.1016/j.foodhyd.2020.106496.
- Stencel-Baerenwald, J. E., K. Reiss, D. M. Reiter, T. Stehle, and T. S. Dermody. 2014. The sweet spot: Defining virus–sialic acid interactions. Nature Reviews. Microbiology 12 (11):739–49. doi: 10.1038/nrmicro3346.
- Stevens, C. R., T. M. Millar, J. G. Clinch, J. M. Kanczler, T. Bodamyali, and D. R. Blake. 2000. Antibacterial properties of xanthine oxidase in human milk. Lancet (London, England) 356 (9232):829–30. doi: 10.1016/S0140-6736(00)02660-X.
- Sukhov, I., M. Lebedeva, I. Zakharova, K. Derkach, L. Bayunova, I. Zorina, N. Avrova, and A. Shpakov. 2020. Intranasal administration of insulin and gangliosides improves spatial memory in rats with neonatal type 2 diabetes mellitus. Bulletin of Experimental Biology and Medicine 168 (3):317–20. doi: 10.1007/s10517-020-04699-8.
- Superti, F. 2020. Lactoferrin from bovine milk: A protective companion for life. Nutrients 12 (9):2562. doi: 10.3390/nu12092562.
- Suzuki, S., H. Kimoto-Nira, H. Suganuma, C. Suzuki, T. Saito, and N. Yajima. 2014. Cellular fatty acid composition and exopolysaccharide contribute to bile tolerance in Lactobacillus brevis strains isolated from fermented Japanese pickles. Canadian Journal of Microbiology 60 (4):183–91. doi: 10.1139/cjm-2014-0043.
- Tang, F. L., J. Wang, Y. Itokazu, and R. K. Yu. 2021. Ganglioside GD3 regulates dendritic growth in newborn neurons in adult mouse hippocampus via modulation of mitochondrial dynamics. Journal of Neurochemistry 156 (6):819–33. doi: 10.1111/jnc.15137.
- Thomas, S., L. Mathew, and K. S. Rishad. 2018. Isolation and molecular identification of phosphate solubilizing bacteria, Bacillus licheniformis UBPSB-07 capable of enhancing seed germination in Vigna radiata L. Phytomorphology: An International Journal of Plant Morphology 68 (1-2):13–8.
- Thompson, A. K., A. Couchoud, and H. Singh. 2009. Comparison of hydrophobic and hydrophilic encapsulation using liposomes prepared from milk fat globule-derived phospholipids and soya phospholipids. Dairy Science and Technology 89 (1):99–113. doi: 10.1051/dst/2008036.
- Vance, J. E. 2008. Phosphatidylserine and phosphatidylethanolamine in mammalian cells: Two metabolically related aminophospholipids. Journal of Lipid Research 49 (7):1377–87. doi: 10.1194/jlr.R700020-JLR200.
- Vors, C., L. Joumard-Cubizolles, M. Lecomte, E. Combe, L. Ouchchane, J. Drai, K. Raynal, F. Joffre, L. Meiller, M. Le Barz, et al. 2020. Milk polar lipids reduce lipid cardiovascular risk factors in overweight postmenopausal women: Towards a gut sphingomyelin-cholesterol interplay. Gut 69 (3):487–501. doi: 10.1136/gutjnl-2018-318155.
- Walstra, P., P. Walstra, J. T. Wouters, and T. J. Geurts. 2005. Dairy science and technology. CRC Press.
- Wang, M., C. Cao, Y. Wang, H. Li, H. Li, and J. Yu. 2021. Comparison of bovine milk fat globule membrane protein retention by different ultrafiltration membranes using a label-free proteomic approach. LWT 144 (10):111219. doi: 10.1016/j.lwt.2021.111219.
- Weiland, A., A. Bub, S. W. Barth, J. Schrezenmeir, and M. Pfeuffer. 2016. Effects of dietary milk- and soya-phospholipids on lipid-parameters and other risk indicators for cardiovascular diseases in overweight or obese men – two double-blind, randomised, controlled, clinical trials. Journal of Nutritional Science 5: E 21. doi: 10.1017/jns.2016.9.
- Wu, Y., B. Mou, S. Song, C.-P. Tan, O.-M. Lai, C. Shen, and L.-Z. Cheong. 2020. Curcumin-loaded liposomes prepared from bovine milk and krill phospholipids: Effects of chemical composition on storage stability, in-vitro digestibility and anti-hyperglycemic properties. Food Research International (Ottawa, Ont.) 136:109301. doi: 10.1016/j.foodres.2020.109301.
- Wu, Y., X. Zhang, D. Han, Y. Pi, S. Tao, S. Zhang, S. Wang, J. Zhao, L. Chen, and J. Wang. 2021. Early life administration of milk fat globule membrane promoted SCFA-producing bacteria colonization, intestinal barriers and growth performance of neonatal piglets. Animal Nutrition (Zhongguo xu mu Shou yi Xue Hui) 7 (2):346–55. doi: 10.1016/j.aninu.2020.07.012.
- Xu, C., Q. Ban, W. Wang, J. Hou, and Z. Jiang. 2022. Novel nano-encapsulated probiotic agents: Encapsulate materials, delivery, and encapsulation systems. Journal of Controlled Release: Official Journal of the Controlled Release Society 349:184–205. doi: 10.1016/j.jconrel.2022.06.061.
- Yadav, D., K. Srikanth, and K. Yadav. 2018. Flavors in probiotics and prebiotics. In: Flavors for nutraceutical and functional foods, 51–74. CRC Press.
- Ye, A., H. Singh, M. W. Taylor, and S. Anema. 2004. Interactions of whey proteins with milk fat globule membrane proteins during heat treatment of whole milk. Le Lait 84 (3):269–83. doi: 10.1051/lait:2004004.
- Yw, A., A. Bm, S. B. Shuang, C. Cpt, D. Oml, S. E. Cai, and A. Lzc. 2020. Curcumin-loaded liposomes prepared from bovine milk and krill phospholipids: Effects of chemical composition on storage stability, in-vitro digestibility and anti-hyperglycemic properties. Food Research International 136:109301 doi: 10.1016/j.foodres.2020.109301.
- Yw, A., W. A. Ke, A. Ql, A. Xl, A. Bm, C. Omlb, D. Cpt, and A. Lzc. 2021. Selective antibacterial activities and storage stability of curcumin-loaded nanoliposomes prepared from bovine milk phospholipid and cholesterol. Food Chemistry 367:130700 doi: 10.1016/j.foodchem.2021.130700.
- Zhang, L., M. Chichlowski, G. Gross, M. J. Holle, L. A. Lbarra-Sánchez, S. Wang, and M. J. Miller. 2020. Milk fat globule membrane protects Lactobacillus rhamnosus GG from bile stress by regulating exopolysaccharide production and biofilm formation. Journal of Agricultural and Food Chemistry 68 (24):6646–55. doi: 10.1021/acs.jafc.0c02267.
- Zheng, H., R. Jiménez-Flores, and D. W. Everett. 2014. Lateral lipid organization of the bovine milk fat globule membrane is revealed by washing processes. Journal of Dairy Science 97 (10):5964–74. doi: 10.3168/jds.2014-7951.
- Zupančič, Š., K. Škrlec, P. Kocbek, J. Kristl, and A. Berlec. 2019. Effects of electrospinning on the viability of ten species of lactic acid bacteria in poly(ethylene oxide) nanofibers. Pharmaceutics 11 (9):483. doi: 10.3390/pharmaceutics11090483.