Abstract
Even though plant proteins are more plentiful and affordable than animal proteins in comparison, direct usage of plant-based proteins (PBPs) is still limited because PBPs are fed to animals as feed to produce animal-based proteins. Thus, this work has comprehensively reviewed the effects of various factors such as pH, temperature, pressure, and ionic strength on PBP properties, as well as describes the protein interactions, and extraction methods to know the optimal conditions for preparing PBP-based products with high functional properties and health benefits. According to the cited studies in the current work, the environmental factors, particularly pH and ionic strength significantly affected on physicochemical and functional properties of PBPs, especially solubility was 76.0% to 83.9% at pH = 2, while at pH = 5.0 reduced from 5.3% to 9.6%, emulsifying ability was the lowest at pH = 5.8 and the highest at pH 8.0, and foaming capacity was lowest at pH 5.0 and the highest at pH = 7.0. Electrostatic interactions are the main way for protein interactions, which can be used to create protein/polysaccharide complexes for food industrial purposes. The extraction yield of proteins can be reached up to 86–95% with high functional properties using sustainable and efficient routes, including enzymatic, ultrasound-, microwave-, pulsed electric field-, and high-pressure-assisted extraction. Nondairy alternative products, especially yogurt, 3D food printing and meat analogs, synthesis of nanoparticles, and bioplastics and packaging films are the best available PBPs-based products. Moreover, PBPs particularly those that contain pigments and their products showed good bioactivities, especially antioxidants, antidiabetic, and antimicrobial.
GRAPHICAL ABSTRACT
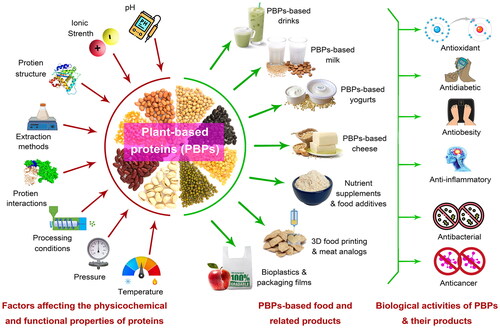
Introduction
Recently, the production of edible and non-edible products from plant sources is increasing day by day, owing to a rise in disease-related diets, an increase in global population that is forecast to reach around 9.7 billion by 2050, and, more critically, climate change (Yang et al. Citation2023; Mirón, Linares, and Díaz Citation2023; Rashwan et al. Citation2021). Therefore, scientists have paid more attention to fabricating sustainable and promising products from plant sources, especially plant-based proteins (PBPs). Due to concerns about health, the environment, and animal welfare, consumers are growing more interested in switching from meat to alternative proteins (Webb, Li, and Alavi Citation2023; Wang et al. Citation2023a; Kannan et al. Citation2008; Qamar et al. Citation2020; Banach et al. Citation2022). Proteins are the most versatile macromolecules in living systems, which are principally made up of amino acids () and play critical roles in nearly all biological processes. Proteins have several unique properties, such as serving as catalysts, transporting and storing other oxygen molecules, offering mechanical support and immunological protection, generating motion, and transmitting nerve impulses, as well as building the enzymes and governing growth and differentiation (Hadidi, Aghababaei, et al. Citation2023; Kumar et al. Citation2022a; Kannan et al. Citation2008).
Plant protein technology is a key area of biotechnology for addressing the issue of increasing protein demand by humans. Although PBPs are more abundant and less expensive than animal proteins, direct PBPs consumption is still restricted since PBPs are fed to animals as feed in order to generate animal-based proteins like milk, meat, and eggs. Nevertheless, only around 3% of plant proteins are converted into animal proteins, demonstrating the inefficiency of this process (Wang et al. Citation2023a; Kumar et al. Citation2022a). Consequently, overcoming the drawback of using PBPs as a source of protein for humans can be accomplished by the direct production of food products from protein-rich plants. There are many plants rich in protein, such as soybean, lentils, pinto beans, quinoa, wheat, sorghum, chia seeds, nuts, etc., which can be used to produce and prepare food-rich protein (Rashwan, Osman, and Chen Citation2023; Bian et al. Citation2023; Zhao et al. Citation2023a). Several recent studies investigated the possibility of using PBPs to produce food products directly or incorporating protein extracted from plants in various edible or non-edible products. These products such as nondairy milk products (e.g., beverages, yogurt, cheese, etc.), plant-based meat (artificial meat), nutrient supplements, bioplastics, and others (Kumar et al. Citation2022a; Rashwan, Osman, and Chen Citation2023; Wang et al. Citation2023b; Du, Wang, and Zheng Citation2023; da Silva, Velasco, and Fakhouri Citation2023; Aydar et al. Citation2023; Zhang et al. Citation2023).
Therefore, using PBPs in the development of new food products makes complete sense as they are rich in nutritional components such as phenolic compounds, anthocyanins, and catechins, in addition to fibers. As a result, several biological activities, including antioxidant, antidiabetic, anti-obesity, anticancer, and others, can be found in PBPs products. Besides, the unique structures and functional properties of proteins such as solubility water holding capacity (WHC), oil holding capacity (OHC), color, gelation, viscosity and texture, emulsification, foam formation, flavor-binding properties, curdling, and enzymatic browning can play important roles in the development of new functional food products (Chang et al. Citation2022; Webb, Li, and Alavi Citation2023; Zhao et al. Citation2023a; Rinaldoni et al. Citation2014). This review discussed the factors affecting the physicochemical and functional properties of PBPs. Besides, the present review summarized the protein-rich plants, the potential interactions between protein and other molecules, as well as the potential application of plant proteins for the development of food and related products. In this context, the health benefits of protein-rich plants and their products have also been reported. Therefore, this review could be a useful piece of work on protein-rich plants and their products that may attract the attention of not only food researchers but also industrial individuals.
Protein-rich plant sources
Proteins are the primary elements of agricultural raw materials, serving two distinct (but complementary) functions: bio- and techno-function. Protein bio-functionality refers to their nutritional and physiological properties, whereas protein techno-functionality refers to their physicochemical properties that affect the appearance, texture, and stability of food products (for example, solubility, viscosity, foaming, emulsifying and gelling ability, fat absorption capacity) (Pojić, Mišan, and Tiwari Citation2018; Qamar et al. Citation2020). As shown in , there are many PBPs including soybean (Glycine max), faba bean (Vicia faba), lupin (Lupinus angustifolius), rapeseed (Brassica rapa/napus subsp. Oleifera), flaxseed (Linum usitatissimum), oil hemp (Cannabis sativa), buckwheat (Fagopyrum esculentum), and quinoa (Chenopodium quinoa), maize (Zea mays), and others, which considered the promising sources for protein under current climate changes. These legumes, cereals, oil crops, and pseudo cereals may provide good alternatives to animal protein while also contributing to the diversity, environmental, and economic sustainability of local agricultural output. They are also high in energy, fiber, high-quality protein, and macro- and micronutrients (Zhou et al. Citation2022a; Kalman Citation2014; Al-Gaby Citation1998; Elsohaimy, Refaay, and Zaytoun Citation2015; Abugoch et al. Citation2008).
Table 1. Protein content and amino acids profile of some protein-rich plant.
For instance, soybean seeds contain approximately 42.95 to 46.32% of the protein (Zhou et al. Citation2022a), which includes several amino acids (g/100 g of protein) such as alanine (3.59), arginine (6.67), aspartic acid (10.2), cystine (1.46), glutamic acid (17.45), glycine (3.6), histidine (2.3), isoleucine (4.25), leucine (6.78), lysine (5.33), methionine (1.13), phenylalanine (4.59), proline (4.96), serine (4.59), threonine (3.14), tryptophan (1.12), tyrosine (3.22), and valine (4.1) (Kalman Citation2014). Another study showed that the protein content in different varieties of brown rice ranges from 6.8 to 11.2% (as N × 5.95) (Juliano, Antonio, and Esmama Citation1973). The abundant amino acids in brown rice, as reported by Kalman (Citation2014), including glutamic acid, aspartic acid, leucine, arginine, valine, alanine, phenylalanine, and tyrosine (13.9, 6.94, 6.41, 6.32, 4.56, 4.47, 4.41, 4.26 g/100g of oryzatein 90 silk isolate, respectively). Moreover, quinoa seeds are considered a promising protein source, where the previous research found that the protein content of quinoa seeds ranged from 12% to 23% on average. Quinoa seed flour has a greater overall protein content than cereal grains such as barley (11%), rice (7.5%), and corn (13.4%). In addition, the protein content of quinoa is higher than the protein content of groundnut (8.8–11.6%) and cowpea (8.8–12.1%). (Elsohaimy, Refaay, and Zaytoun Citation2015; Abugoch et al. Citation2008; Abdelshafy, El-Naggar, and Kenawi Citation2022a). The amino acids composition of quinoa protein isolate was histidine (2.76), leucine (4.60), isoleucine (1.30), lysine (17.13), methionine with cystine (1.70), phenylalanine with tyrosine (9.34), threonine (1.47), and valine (2.03) as essential amino acids, while the non-essential amino acids were alanine (5.34), glycine (9.60), proline (0.10), serine (2.57), tyrosine (2.88), glutamic (12.80), aspartic (8.54), and arginine (0.03) (g/100 g protein) (Elsohaimy, Refaay, and Zaytoun Citation2015).
However, the protein amount that exists in plants can be affected by several factors, including the plant cultivar, soil type, agricultural transactions, and environmental conditions. For example, four different cultivars of peanut seeds cultivated in Georgia, Oklahoma, Virginia, and Texas during the 1987 and 1988 seasons were chosen to study the effects of location and season on protein, polypeptides, and free amino acids in peanut seeds. According to the findings, Oklahoma, Texas, and Georgia peanuts had the highest concentration of free amino acids during the 1987 growing season, followed by Virginia peanuts. Additionally, analysis of the protein profiles of the peanuts produced in various regions revealed that the season and the location had a significant impact on the seed’s Arachne monomer and polymer levels (Basha Citation1992). Moreover, the protein content in 7 Egyptian cultivars was as follows: Sakha 3 (26.77%), Sakha 2 (26.12%), Sakha 4 (25.61%), Missr 1 (25.56%), Giza 843 (24.17%), Nubaria 1 (24.11%), and Giza 3 (23.04%) (Hendawey and Younes Citation2013). Besides, the authors evaluated 17 commercial cultivars of faba bean for protein content at four locations in Denmark and Finland during 2016 – 2018. They found that cultivar and environment had limited effects on protein content, where the minimum protein content was observed in Lynx (26.6%) and the maximum in Mistral (34.9%) (Skovbjerg et al. Citation2020).
Physicochemical and functional properties of plant proteins
The physicochemical and functional properties of dietary proteins have been categorized into three major types based on their mechanism of action as follows; (a) hydration-related qualities (including water and oil absorption, solubility, thickening, and wettability), (b) features linked to protein structure and rheological properties (such as viscosity, elasticity, adhesiveness, aggregation, and gelification), and (c) properties associated to protein surface characteristics (e.g., emulsifying and foaming, generation of protein-lipid films, and whippability). Besides, the most crucial functional aspects of protein in food are its solubility, water and fat-binding capacities, gel-forming and rheological behaviors, emulsifying abilities, foaming, and whipping abilities (Shevkani et al. Citation2015; Tang and Sun Citation2010; Li et al. Citation2020a). These properties can play important roles in the development of new functional food products, which can affect the biological activities of proteins and their products (Chang et al. Citation2022; Webb, Li, and Alavi Citation2023; Zhao et al. Citation2023a; Rinaldoni et al. Citation2014). Therefore, knowing and studying the factors that can affect these properties either positively or negatively can lead to finding the optimal conditions to extract and prepare suitable protein-based products.
Factors affecting plant protein properties
The physicochemical and functional properties of dietary proteins can be influenced by several environmental factors (such as pH, temperature, pressure, and ionic strength), and processing conditions (e.g., sonication, irradiation, cooking, germination, etc.) (). Besides, protein structure-function relationships govern how proteins interact with one another and with other substances in complex food systems (Ma et al. Citation2018; Tang and Sun Citation2011; Zhou et al. Citation2015; Zhou et al. Citation2016; Ngui et al. Citation2021). For instance, soybean protein isolate showed the lowest emulsifying activity at pH 5.8 and the highest emulsifying activity at pH 8.0. The results indicate that the emulsifying activity could increase upon the pH values being increased, and this phenomenon could be due to the greater solubility of proteins when the pH value increased (Liu et al. Citation2008). Additionally, at pH 5.0 (around the isoelectric point), the pea protein had the least ability to emulsify, and consequently, the emulsions that resulted were the most prone to coalescence and creaming. The ability of pea protein to emulsify at pH 3.0 was superior to that at neutral (7.0) or alkali (9.0) pH values (Liang and Tang Citation2013). As well, the surface hydrophobicity of protein is an important physicochemical parameter affecting the surface-related functional properties of protein. In which, surface hydrophobicity of protein is an important physicochemical parameter affecting the surface-related functional properties of the protein, where surface hydrophobicity of purified legumin at pH 7.0 was almost two times that of purified vicilin (2075 vs 1005), indicating much higher exposure of hydrophobic clusters (Liang and Tang Citation2013).
Figure 2. The potential environmental factors affecting the physicochemical and functional properties of proteins.
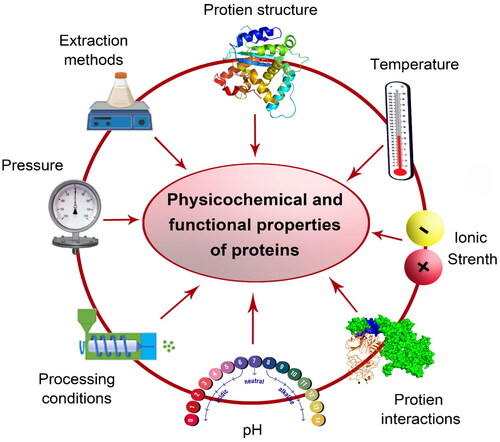
Moreover, amaranth protein isolates showed a range of solubilities, with the lowest solubility at pH 5.0 and the highest solubility in both acidic and alkaline environments. At pH = 2.0, the solubility of amaranth protein isolates ranged from 76.0–83.9%, while it significantly reduced at pH = 5.0 to 5.3–9.6%. Besides, the lowest emulsifying activity index (4.6–17.01 m2 g−1) of amaranth protein isolates was observed around the isoelectric region (pH 5.0), while the highest (20.7–52.7 m2 g−1) was found at pH = 9. Amaranth protein isolates had the lowest foaming capacity at pH 5.0 and the highest foaming capacity at pH 7.0 (Shevkani et al. Citation2014). The lower solubility, emulsifying activity index, and foaming capacity at pH 5.0 could be due to the isoelectric point of amaranth proteins. At their isoelectric point, proteins had no net charge; therefore, there were no repulsive interactions, and protein-protein interactions were unfavorable to solubility (Shevkani et al. Citation2014).
Moreover, the solubility of soy glycinin at ionic strength (I) = 0.2 (1.8 mM NaH2PO4, 3.1 mM Na2HPO4, and 0.089 M NaCl) was lower than that of glycinin at ionic strength = 0.06 (2.4 mM NaH2PO4, 2.5 mM Na2HPO4, and 4.9 mM NaCl), and ionic strength = 0.6 (1.6 mM NaH2PO4, 3.3 mM Na2HPO4, and 0.289 M NaCl. Na2HPO4) at pH 7.0. These results could be because the I = 0.2 led to disrupting the intermolecular aggregation of glycinin and exposing some of the hydrophobic regions of glycinin to the surface, which decreased the solubility of the glycinin (Zhou et al. Citation2016). Moreover, the solubility protein isolates from Amygdalus pedunculata Pall seeds increased first and then decreased with the increase in the sodium chloride concentration, which reached 24.7% (as maximum) when the concentration of sodium chloride was 0.4 mol/L. This effect may be because the solubility of the protein can be increased due to the increase of ionic strength of the salt dissolving effect in the low concentration of the salt solution, while when the concentration reaches a certain level, the repulsive electronic interactions occur between charged protein molecules are lowered by increasing ionic strength (Li et al. Citation2020a).
Furthermore, the treatment of protein using some processes, including high pressure (HP) and high-intensity ultrasound, can improve the functional properties of the protein (Zhou et al. Citation2016; Molina, Papadopoulou, and Ledward Citation2001). Despite soy protein isolate (SPI) showing a low surface hydrophobicity, it displayed the highest emulsifying activity index value after 400 MPa treatment. According to speculation, pressure at 400 MPa split the SPI's 7S into partially or completely denatured monomers that increased surface activity. However, at the same time, the unfolding of the 11S polypeptides within the hexamer caused aggregation, which harmed the surface hydrophobicity of the SPI (Molina, Papadopoulou, and Ledward Citation2001). In addition, treatment of soybean glycinin by high-intensity ultrasound (HIU; 20 kHz at 80 W cm−2 from 0 to 40 min) in three ionic strengths (I = 0.06, 0.2, and 0.6) at pH 7.0 increased emulsion stability and decreased the turbidity. Besides, HIU treatment increased the surface hydrophobicity of soybean glycinin at I = 0.06 and 0.2, while it remained unchanged at I = 0.6 (Zhou et al. Citation2016). The particle size of glycinin was gradually reduced from 224.3 nm to 65.2 nm after 40 min of HIU treatment at I = 0.06, while the particle size dramatically from 780.1 nm to 105.0 nm at I = 0.2 (Zhou et al. Citation2016). After 40 min of HIU treatment, the protein solubility of glycinin rose from 88% and 53% to 94% and 77%, respectively, at I = 0.06 and I = 0.2. The solubility of glycinin rose from 87% to 99% after 5 min of HIU at I = 0.6. However, the protein solubility was lowered to 88% by a longer HIU period (40 min) at I = 0.6 (Zhou et al. Citation2016).
Moreover, cowpea protein isolates (CPIs), which were extracted at pHs of 8.0 (A8) and 10.0 (A10), were exposed to thermal (70 and 90 °C) and high hydrostatic pressure (HHPTs, 200, 400, and 600 MPa) treatments. The result showed that the surface hydrophobicity value of CPIs that were extracted at pH 10 was higher (twice) than that were extracted at pH 8. This might be the result of irreversible modifications made to the proteins during the extraction process, like a distinct occurrence of aggregation and/or dissociation (Peyrano, Speroni, and Avanza Citation2016). Besides, the Bambara bean protein’s hydrophobicity was significantly higher at pH 4 and lower at pH 9. At pH 4, the protein’s net charge was close to zero, and hydrophobic protein-protein interactions predominated, but at pH 9, the protein was negatively charged, and protein-water interactions predominated. At pH 4, hydrophobicity decreased with increasing temperature up to 70 °C, as well as the heat treatment caused a decrease in protein hydrophobicity at pH 9 (Ngui et al. Citation2021). The decrease in hydrophobicity was most likely caused by protein aggregation via hydrophobic contact, whereas the increase was caused by the partial unfolding and exposing of regions previously buried by the bulky protein structure’s steric hindrance (Ngui et al. Citation2021). The emulsifying capacity of protein isolate was highest at 80 °C and pH 9, while emulsion stability was highest at pH 4. At pH 4 and 7 protein solubility was decreased when temperature was increased, while an increase in the protein solubility was observed at pH 9 and 100 °C (Ngui et al. Citation2021). Besides, the germination treatment improved the physical and functional properties of faba bean isolated protein, such as the water absorption index (2.97 g/g) and the foaming stability (140.13 mL/100 mL). Pressure-cooked faba beans had higher functional properties than other control beans, such as a greater water solubility index (2.12 g/100 g) and water absorption capacity (2.02 g/g) (Kumar, Sadiq, and Anal Citation2022b). Another study also showed that higher functional properties were observed in germinated amaranth flour than in raw amaranth flour (Chauhan, Saxena, and Singh Citation2015). The enhancement of protein functional properties during germination is due to the activity of proteases that can form lower molecular weight compounds (protein) (Kumar, Sadiq, and Anal Citation2022b; Chauhan, Saxena, and Singh Citation2015).
Additionally, Yao et al. (Citation2022) investigated the effects of γ-irradiation treatment on the physicochemical properties and functional properties of rice protein. They showed that when the rice protein explored to γ-irradiation at a dose of 2 kGy, the particle size distribution of protein has a main peak at 100–1000 nm and an impurity peak at 10–100 nm, where the average particle size is the smallest. In contrast, the particle size distribution of rice protein showed miscellaneous peaks on the right side of 10–100 nm and 1000 nm when the γ-irradiation treatment dose is greater than 2 kGy, indicating that the average particle size also increased (Yao et al. Citation2022). This could be because the γ-irradiation treatment destroys intermolecular connections, resulting in smaller rice protein particles. When the dose of γ-irradiation is too high, cross-linking, electrostatic interaction, hydrophobic interaction, and disulfide bond formation between proteins occur, causing them to aggregate into larger molecular weight proteins and increase particle size (Li et al. Citation2020b; Yong Sik and Bin Citation2000). Rice protein also showed the highest value of surface hydrophobicity (160.45) when the irradiation dose was 2 kGy, while when the γ-irradiation dose was too large (5 kGy), surface hydrophobicity decreased (123.3) (Yao et al. Citation2022). This could be because the radiation treatment exposes the hydrophobic residues within the protein, increases the number of hydrophobic sites, and improves the capacity to touch the external environment, hence boosting surface hydrophobicity (Wang et al. Citation2017b). On the other hand, when the γ-irradiation dose is excessively high, the hydrophobic sites of the protein are reduced due to a rise in β-sheets in the secondary structure of the protein, hence lowering surface hydrophobicity (Yuan et al. Citation2009).
Furthermore, the solubility of rice protein rose dramatically after γ-irradiation treatment; as the irradiation dose increased, the solubility of rice protein increased and eventually declined. The solubility of rice protein is 69.18% when the γ-irradiation dose is 2 kGy (Yao et al. Citation2022). This could be a result of γ-irradiation treatment, which decreases β-sheets in the protein’s secondary structure, opens the protein structure, exposes the internal hydrophilic groups, and makes it easier for water molecules to bind, increasing the protein’s solubility (Nazari et al. Citation2018). While, when the γ-irradiation dose increased to 5 kGy, the protein molecules either cluster or the protein residues are oxidized to form disulfide bonds, which decreases the protein’s hydration and rice protein’s solubility (Yao et al. Citation2022; Amagliani et al. Citation2017). Moreover, the greatest water and oil-retaining capabilities of rice protein are 5.89 and 3.45 g/g, respectively, with a γ-irradiation dose of 2 kGy (Yao et al. Citation2022). This might be because γ-irradiation treatment exposes internal groups and opens the protein structure. The exposure of hydrophilic groups, which enhances the likelihood of contact with water molecules and consequently improves the water-holding capacity, is intimately tied to the protein’s ability to hold water. The increase in surface hydrophobicity of the protein, which encourages more efficient adsorption and diffusion between the protein and the oil droplets, may be the cause of the increase in oil holding capacity (Yao et al. Citation2022; Li et al. Citation2019). On the contrary, a high γ-irradiation dose causes the protein secondary structure β-sheets to increase, the structure to become tighter and more orderly, and the proteins to be more susceptible to cross-linking. This reduces the protein’s contact with water and oil droplets, which leads to a decrease in water and oil holding capacity (Yao et al. Citation2022; Wang et al. Citation2017a).
Protein interactions
Proteins are the key molecules we need to comprehend because they are the main mediators of bio-functions in various biological systems, and their activity is determined by their interactions with other molecules, including polysaccharides, lipids, polyphenols, and most notably, proteins. The protein-protein interaction can occur with other copies of the same protein (homo-oligomers), distinct proteins (hetero-oligomers), or both, as in complicated molecular machinery. The interactome is the network of all protein-protein interactions. Because proteins are gregarious and associate in a variety of ways, only a few of these interactions are biologically significant, and the interactome is much less than the sum of all conceivable interactions. However, forming protein complex systems with other food components (polysaccharides, lipids, polyphenols, etc.) can affect the behavior of protein in food systems during processing, manufacturing, storage, and preparation, e.g., sorption, solubility, gelation, surfactant, ligand-binding, and film formation (Gentile Citation2020; Doublier et al. Citation2000; Soleymani et al. Citation2022; Chen, Jones, and Campanella Citation2023; Rashwan et al. Citation2024). Many foods naturally contain both proteins and polyphenol compounds such as phenolics, flavonoids, tannins, etc. (Rashwan, Osman, and Chen Citation2023). In normal conditions, these polyphenols are not in contact with other macronutrients in plant cell vacuoles. In contrast, when the integrity of plant tissue is disrupted during food processing and production, the phenolic compounds can probably interact with intracellular or extracellular molecules () (Günal-Köroğlu, Lorenzo, and Capanoglu Citation2023; Rashwan et al. Citation2022). In which phenolic compounds are quickly oxidized and can interact simultaneously with protein side chains (bound phenolics) during conventional protein extraction from sources of PBPs because of the alkali conditions. As a result, the characteristics of proteins may be dramatically altered. For instance, their digestibility may decrease, and their organoleptic properties may change, causing undesired browning reactions and haze formation in addition to altering their functional characteristics (Yan et al. Citation2020). For example, the authors found that 56–62% of phenolics were bound in flaxseed protein isolate, and 59–85% of phenolics were free in soybean protein isolate (Alu’datt et al. Citation2016). In comparison with dephenolized sunflower protein isolate, the phenolic-rich sunflower protein isolate showed a decrease in protein band intensity, enthalpy, free/total sulfhydryl groups, surface hydrophobicity, and an increase in denaturation temperature (Malik, Sharma, and Saini Citation2016). Moreover, the formation of protein-phenolic complex (protein isolate from Cinnamomum camphora seed kernel) resulted in a decrease of free sulfhydryl groups content from 17.13 (μmol/g) for protein isolate (PI) to 12.54 (μmol/g) for protein-phenolic complex (PPC). In addition, surface hydrophobicity reduced from 194.44 for PI to 133.81 for PPC, particle size increased from 64.30 (nm) for PI to 86.30 (nm) for PPC, and zeta potential for PI was −28.10 (mV) and increased to −35.00 (mV) for PPC. Furthermore, the protein-phenolic complex exhibited higher solubility, thermal stability, and better foaming and emulsifying properties than those of protein isolate only (Yan et al. Citation2020).
Figure 3. The potential interactions between (A) protein-polyphenol and (B) protein-polysaccharides.
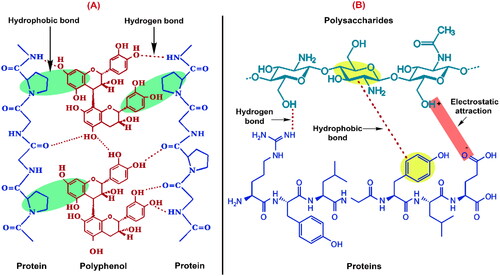
Electrostatic interactions are considered the main interaction route to create protein/polysaccharide complexes, which occur between oppositely charged macromolecules of protein and polysaccharide due to the presence of hydroxyl groups in these components (). Besides, the hydroxyl groups of protein and polysaccharide are involved in hydrogen bonding whereas the aromatic rings could be involved in hydrophobic interaction (Gentile Citation2020). In which these soluble complexes continue to congregate to decrease the free energy of the system, and eventually, their size and surface characteristics cause insolubilization (Schmitt and Turgeon Citation2011). However, there are several factors, such as pH, ionic strength, medium temperature, and the biopolymer molecular weight, charge, and nature, that can influence the formation of polysaccharide-protein complexes and their solubility (Warnakulasuriya and Nickerson Citation2018; Aryee and Nickerson Citation2014; Yildiz et al. Citation2018). For instance, the presence of monovalent ions in lentil protein isolate-anionic polysaccharides complexes (e.g., κ-carrageenan and gellan gum) reduced their optical density, and larger reductions were observed upon the addition of divalent ions. This phenomenon could be due to the presence of ions that can reduce the thickness of the electric double layer (diffuse plus stern layers) on the surfaces of protein and polysaccharides, decreasing the amount of attractive forces between their driving complexation (Aryee and Nickerson Citation2014). Furthermore, the highest protein solubility was observed in the pea protein isolate (PPI)-modified starch complexes followed by SPI-modified starch complexes and PPI-gum Arabic (72.50, 66.40, and 65.20%, respectively), while the lower solubility was observed in SPI-gum Arabic (62.02%), PPI-pectin complexes (59.16%), and SPI-pectin complexes (58.32%) (Yildiz et al. Citation2018).
Extraction technologies of protein
One of the most important processes that requires innovation is protein extraction techniques. To maximize protein extraction yield without compromising environmental sustainability or the nutritional, technological, and functional properties of proteins, eco-innovative methods are desperately needed. As far as we are aware, the primary structural connections between proteins and other macromolecules in plants include carbohydrates, lipids, fiber, and polyphenols. In order to release proteins effectively, the extraction method needs to be able to break structural bonds and dissolve cell walls and structural membranes. Numerous investigations investigated the use of solvents, salt, and alkali as traditional extraction techniques in order to accomplish this purpose (Kumar et al. Citation2021; Liu et al. Citation2019; Bou et al. Citation2022). However, the difficulty with these conventional extraction techniques is that they can only effectively disrupt structural molecules, removing only approximately half of the proteins that are available in a plant (Zhao et al. Citation2023b; Amagliani et al. Citation2017; Venkateswara Rao et al. Citation2023). According to the examination of amino acid composition, hempseed meal contains 54.5 mg essential amino acids/g total amino acids, and when compared with control experiments, extraction with NaOH, KOH, NaHCO3, or NaCl did not enhance the selective extraction of essential amino acids (Cabral et al. Citation2022).
Therefore, several pretreatment techniques are now being used to overcome this difficulty and facilitate protein extraction with high functional properties in a sustainable and efficient route, including enzymatic and physical approaches (such as pulsed electric field, ultrasound, microwave, high-voltage electrical, and high-pressure), as well as some novel extraction methods including ultrafiltration membrane, pressurized liquids, deep eutectic solvents, and others () (Zhao et al. Citation2023b; Bedin et al. Citation2020; Liu et al. Citation2019; Mala, Sadiq, and Anal Citation2021; Tsubaki et al. Citation2009; Hernández-Corroto et al. Citation2020). For instance, Wahlström et al. (Citation2017) used novel carboxylate salt-urea aqueous deep eutectic solvents to study the yield of protein recovered from Brewer’s wasted grain. They discovered that the protein yield obtained from 90-weight percent NaAcO: urea (molar ratio 1:2) was greater than that obtained from choline chloride: urea (1:2), which produced protein extractants with up to 79% extraction yields. The dissolving of naturally insoluble proteins or proteins that had become denatured during the brewing process was the cause of the high extraction yield from brewer’s spent grain at 90 weight percent NaAcO: urea (1:2) (Wahlström et al. Citation2017). Besides, deep eutectic solvents (DESs) (including choline chloride-glycerol, choline chloride-oxalic acid, and choline chloride-urea) were developed for extracting protein from seabuckthorn seed meal and compared with alkaline. The results indicated that using deep eutectic solvents produced protein with less β-sheet, more β-turn, more essential amino acids, and higher total amino acid content, especially choline chloride-urea, where this protein also showed the highest digestibility in vitro (by pepsin) (54.2%) (Hernández-Corroto et al. Citation2020).
Table 2. Description, advantages, and limitations of protein extraction methods.
Moreover, multi-enzymatic pretreatment was applied to extract the protein from okara (a by-product of tofu and soy drink production), where the okara cell walls were hydrolyzed using multi-enzyme complex Viscozyme (contains various carbohydrases) to make protein extraction easier. The findings demonstrated that 53 °C, pH = 6.2, and 4% enzyme concentration were the ideal conditions for maximizing protein content and recovery in protein concentrate. A protein content of 56% (dry weight basis) and a recovery of 28% were obtained under these circumstances, which is an increase of 17 and 86%, respectively, compared to the sample without enzymatic pretreatment (de Figueiredo et al. Citation2018). Besides, the enzyme-assisted extraction showed the highest protein yield extracted from sesame seed meal, which was above 45% protein yield. On the other hand, the E-Precipitate section of the enzyme extraction had the lowest protein yield, which was a result that was expected given that it was the final step in the enzyme extraction (Koysuren, Oztop, and Mazi Citation2021). However, because of the high expense, complexity, and potential racemization of amino acids associated with enzymatic pretreatment, physical technologies are attracting the interest of researchers (Ampofo and Ngadi Citation2022).
Furthermore, the effect of wampee seed protein (WSP) extraction using the ultrasonic and alkaline solution with the application of the response surface methodology on WSP protein yield as well as the functional properties of the protein, such as protein solubility, water and oil holding capacity, and emulsifying and foaming properties as compared with SPI, were studied by (Liu et al. Citation2019). They found that the best extraction conditions were attained when the solid-solvent ratio, ultrasonic time, and pH were 1:29 g/mL, 64 min, and 12, respectively. Under these conditions, the protein yield was 15.06%. Moreover, they showed that ultrasonic time and pH had a substantial impact on the yield. Additionally, WSP had a much better OHC, emulsion activity index, and emulsion stability index than SPI, as well as a solubility that was higher than SPI's and an isoelectric point that was close to 3.0. Because the protein molecules were partially unfolded and the ultrasonic treatment decreased the protein particle size, the solubility of WSP was higher than that of SPI (Zou et al. Citation2017). While the WHC, foaming capacity, and foam stability of WSP were noticeably inferior to those of the SPI (Liu et al. Citation2019). Due to the fewer hydrophilic groups on WSP molecules to connect with water and the more hydrophobic groups to absorb oil, WSP may have a lower WHC and greater OHC than SPI. Additionally, the ultrasonic treatment may make it easier for the protein particles to partially dissolve in water when the WHC is measured, resulting in a lower WHC; in contrast, the smaller protein particles were more likely to bind with oil due to their larger specific surface area, resulting in a higher OHC (Liu et al. Citation2019). Using an ultrasound-assisted extraction technique for isolating the proteins from cowpea pulse crop increased the protein yield from 31.78 to 58.96%, solubility from 57.26 to 68.85%, water-holding capacity from 3.06 to 3.68 g/g, foaming capacity and stability 70.64 to 83.74% and 30.76 to 60.01%, respectively, emulsion activity and stability 47.48 to 64.26% and 56.59% to 87.71%, respectively, in-vitro protein digestibility from 88.27 to 89.99%, and zeta-potential from −32.9 mV to −44.2 mV, as well as particle size dropped from 763 nm to 559 nm in comparison to control (Loushigam and Shanmugam Citation2023).
Furthermore, the effect of microwave-assisted extraction (MAE) on the yield and protein characteristics of soymilk was investigated. The results showed that important criteria for the extraction process included the microwave power level and target temperature, where a power level of 675 W, a target temperature of 80 °C, and a stirring speed of 160 RPM were the ideal MAE conditions. When compared to normal soymilk, the MAE of soymilk significantly increased the extraction yield (24%) and protein content (44.4%) (Varghese and Pare Citation2019). These results may have occurred due to the solubilization of soybean cell walls by microwave heating (Tsubaki et al. Citation2009). Moreover, the protein properties of extracted soymilk, such as protein solubility (16.16%) and protein digestibility (82.69%), were significantly higher than those of steam-heated soymilk (11% and 79.2%, respectively) (Varghese and Pare Citation2019). Protein structures were exposed to lower temperatures during microwave heating, which reduced protein denaturation and enhanced protein solubility, thus increasing the amount of soluble protein in the soymilk sample. In which denaturation of protein at increasing temperatures can lead to the formation of more hydrophobic amino acids in the soymilk, thus reducing protein solubility (Varghese and Pare Citation2019; Cuq et al. Citation2000).
Additionally, the study on ultrafiltration of wastewater from isolated soy protein production using three ultrafiltration membranes (5, 20, and 50 kDa) indicates that the use of these membranes is very promising regarding organic load and protein content reduction. The 5-kDa membrane presented the best results, which showed the lowest permeate flux reduction and highest recovery percentages of protein (52%) from isolated soy protein production wastewater (Cassini et al. Citation2010). The ultrafiltration process for amino acid concentration from quinoa was efficient at both pH 7.0 and pH 9.5, with percentages of approximately 90% obtained at the end of the ultrafiltration, where nearly all amino acids were collected in the concentrate (Navarro-Lisboa et al. Citation2017). Enzymatic treatments by pepsin hydrolysis (15 min) and ultrafiltration improved the solubility and functional properties of faba bean protein, where the solubility increased from 24.4% to 88.8% at pH = 7 and 81.0% at pH = 5. Besides, the foaming capacity of protein increased from 31.2% to 122.2% at pH = 5 and 66.7% to 131.2% at pH = 7, and the oil holding capacity increased from 6.12 to 8.21 g/g (Eckert et al. Citation2019).
Moreover, subcritical and supercritical water technologies can be used to convert various protein-rich biomasses into amino acids that can be used in different foods, cosmetics, and pharmaceutical industries. Zhu et al. (Citation2010) recovered amino acids from soybean dregs using subcritical water hydrolysis, producing total amino acids yield of 22% at subcritical water conditions (330 °C and 20 min) with significant production of lysine, arginine, and alanine. Besides, Park, Jeong, and Chun (Citation2019b) extracted taurine, alanine, and glutamic acid from laver (Porphyra yezoensis) using subcritical water hydrolysis with no significant yield variation between 120 and 180 °C. However, this technology still needs a techno-economic assessment to facilitate the selection of the appropriate operational conditions for execution because it requires high pressure and, thus, high energy demand (Di Domenico Ziero et al. Citation2020).
To summarize, scientists are trying, as the previous section presented, to find suitable processing and extraction technologies for enhancing the physicochemical and functional properties of extracted protein to produce protein-based products with good properties, as presented in the next section.
Potential applications of plant-based proteins
Worldwide, animal-based proteins such as meat and its products, egg and its products, and milk and its products are considered the main sources of consumed proteins (as protein food), especially in the European Union and the United States. In contrast, a larger number of legumes (e.g., soy, beans, etc.) and cereals (e.g., maize, quinoa, sorghum, etc.) are consumed in these countries as animal feed (Rashwan, Osman, and Chen Citation2023; Rashwan et al. Citation2021). Despite livestock being an important source of proteins, it creates substantial environmental impacts that may increase climate change (Mirón, Linares, and Díaz Citation2023). The food and feed system are strongly related to the planetary and health boundaries, therefore changing to a healthy diet will necessitate significant dietary changes in favor of PBPs foods, including nuts, cereals, and legumes (Kumar et al. Citation2022a; Bian et al. Citation2023; Zhao et al. Citation2023a). Furthermore, due to the occurrence of lactose intolerance, celiac, and gluten intolerance-related health problems as well as an aversion to animal cruelty, there is a rising interest in gluten-free and lactose-free products. Therefore, many previous studies have focused on investigating formulation and preparing methods to produce high-protein vegetarian foods, lactose-, and gluten-free products from plants-based proteins as promising foods for increasing health benefits, consumer acceptability, and commercial value () (Hadidi, Aghababaei, et al. Citation2023; Rashwan et al. Citation2021).
Figure 4. Potential applications of plant-based proteins in food and related products and their biological activities.
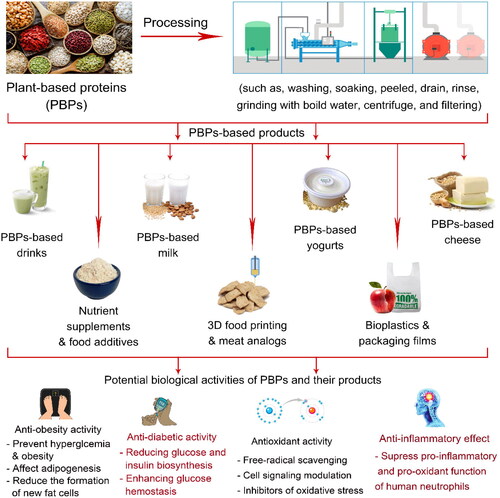
Nondairy alternative products
Due to the prevalence of some emergencies associated with the consumption of animal proteins (dairy milk products) by many people, such as the diet of vegans, lactose intolerance, high cholesterol, milk allergies, anemia, and coronary health diseases, nondairy milk products (plant-based milk products) such as soybean milk, mung bean milk, almond milk, nondairy yogurts, and others have garnered significant attention from both consumers and food scientists (Rashwan et al. Citation2021; Rashwan, Osman, and Chen Citation2023; Wang et al. Citation2023c). Furthermore, the products made from nondairy milk have a high nutritional value because they contain non-allergic proteins, dietary fiber, essential fatty acids, minerals, and vitamins, as well as a variety of bioactive compounds such as phenolics, flavonoids, and procyanidins (Shi et al. Citation2023a; Liu et al. Citation2023; Olabiran, Awolu, and Ayo-Omogie Citation2023). For example, milk alternatives from kidney beans had 1.92–2.32% protein, which was slightly greater than other commercial plant-based milk alternatives, and overall acceptance varied between 2.9 and 4.1 out of 10 (Aydar et al. Citation2023). Besides, soy-based yogurt-enriched essential oil microcapsules of Ocimum gratissimum (OGEOM) showed an increase in water holding capacity by almost 2%. Moreover, throughout five days of storage at room temperature, the pH of soy yogurt declined from 4.75 to 4.15, the titratable acidity climbed from 0.71 to 0.99%, and the syneresis degree decreased from 26.44 to 23.03%. In addition, the bacteria count in soy yogurt without OGEOM rose from zero at day 0 to 4.3.33 × 104 colony forming units (cfu)/100 ml by day five, while it was zero by day three and 3.62 × 103 cfu/100 ml by day five for soy yogurt with OGEOM (Olabiran, Awolu, and Ayo-Omogie Citation2023). The rheological analysis showed that soy-based yogurt owned high G′ and G'’ values and high stability against external stress, demonstrating that more and stronger interactions between soy proteins were built during fermentation. (Wang et al. Citation2023c). Additionally, yogurt made from quinoa and fermented using a modified commercial starter and Weissella confusa demonstrated a better fermentation performance of quinoa utilization. W. confusa accelerated the fermentation process of quinoa yogurt, enhancing its rheological characteristics, texture, and storage stability. The modified starter considerably improved the quinoa yogurt’s nutritional attributes, such as its polyphenol content, antioxidant activity, ability to block digestive enzymes, and ability to lower postprandial blood glucose levels. In addition, the modified starter made the fermented quinoa yogurt’s polyphenols, protein, and fat more digestible and bio-accessible (Liu et al. Citation2023). Therefore, these results can be supplied on a theoretical basis and an improvement direction for the better development of healthier plant-based yogurt like dairy yogurt.
Furthermore, PBP milk can be used as an alternative to produce lactose-free cheese. For instance, soft cheese-like product development enriched with soy protein concentrates showed an increase in protein and fat contents by 6.8–17 and 22–32 (g/100 g), respectively, also the yield increased from 19.0 to 22.5 (Ya). The sensory assessment also showed that cheese-like products containing soy protein concentrates and control products were almost similar in color and overall acceptability (Rinaldoni et al. Citation2014). Moreover, cheese prepared from corn protein (zein), which contains 30% zein displayed a highly analogous behavior to cheddar cheese (as sample standard), where it softened and exhibited increased viscous properties with increasing temperatures. These samples were examined by texture analysis affixed with a specifically designed stretching rig and showed comparable textural characteristics and stretchability (Mattice and Marangoni Citation2020). Another study also investigated the possibility of producing a cheese-like product from the partial substitution of soy milk with almond milk. The results showed that the total titratable acidity of the produced cheese was between 0.23% and 0.33%, representing a significant increase in total titratable acidity from 0.23% to 0.33%. With an increase in the percentage of almond milk in the cheese, the pH increased (6.45 and 6.90). Furthermore, the protein content ranged from 25.67–29.97%, moisture from 22.79–26.45%, fat from 30.58–36.99%, carbohydrate from 7.02–14.61%, ash from 1.20–1.79%, fiber and 0.90–2.03% for fiber. Protein and fat contents increased with an increase in almond milk substitution, while carbohydrate and ash content decreased with an increase in almond milk substitution (Arise et al. Citation2020). Moreover, the effect of different concentrations of coconut milk and soy milk (80:20, 70:30, and 60:40) on the physical and chemical properties of coconut milk cheese, as well as product acceptability, was investigated. The results reported that the coconut milk cheese yield at concentrations 80:20, 70:30, and 60:40 was 37.96%, 34.44%, and 31.48% respectively. The spreadability of coconut milk cheese at the following concentrations, 80:20, 70:30, and 60:40, was 9.83, 9.65, and 9.53 cm, respectively (Halim, Wangrimen, and Fitriani Citation2022). The plant-based model cheeses made from the combination of zein, and PPI showed a total protein content of 30% and a good potential to mimic the melting of conventional cheese (Sutter, Assad-Bustillos, and Windhab Citation2023). To summarize, the previous studies showed the potential of PBPs as an alternative source of milk in beverages, yogurt, and cheese making with improved nutritional value and increased acceptability for the consumer.
3D food printing and meat analogs
Three-dimensional (3D) printing is a revolutionary manufacturing process that constructs products using computer-designed digital model files and various raw materials. 3D food printing (3DFP) technology is a technique that can combine 3D printing and digital cooking technologies to shape food products. This technology offers a user-friendly and adaptable architecture that employs reasonably quick processing with excellent precision and quality at cheap manufacturing costs (Chen, Mu, et al. Citation2019; Shi et al. Citation2023b; Qiu et al. Citation2023). 3D food printing technology has the potential to be used in personalized food design, small-scale food production, and customized nutrition for certain consumer groups such as the elderly (especially with swallowing disorders), pregnant women, children, sports, and other (Chen et al. Citation2022a; Carranza et al. Citation2023). PBPs including SPI, PPI, wheat gluten (WG), and other materials, are rich in essential and non-essential amino acids, have good physicochemical and functional properties, and can be used successfully to print the porous scaffold forms, plant-based meat analogs, and so on (Carranza et al. Citation2023; Shi et al. Citation2023b; Banach et al. Citation2022). For instance, the food matrix containing the mixture of SPI, sodium alginate, and gelatin has favorable 3D food printing characteristics, and the SPI combinations with 2, 6, and 10% gelatin produce outstanding geometries. The SPI and its blends demonstrated shear-thinning behavior and made an excellent 3D printing material. When compared to SPI, the SPI combination with gelatin had lower viscosity and elastic modulus at 35 °C, while the rheological index increased rapidly as the temperature dropped to 25 °C, supporting the deposited layers and maintaining the intended shape. While enhancing the hardness and chewiness of 3D printed geometries, adding sodium alginate and gelatin to SPI did not result in chemical cross-linking between protein subunits during mixing and 3D printing at 35 °C (Chen, Mu, et al. Citation2019).
Furthermore, the authors combined powders of SPI, wheat gluten (WG), and rice protein (RP) to create a high-protein edible ink (25% total dry matter content) that can be used to create 3D-printed meat analogs, and they investigated the rheological properties and printing performance of edible inks made from these three powders. They discovered that these protein-enriched inks have pseudoplastic behavior as well as viscoelastic features. With increasing rice protein proportion, the apparent viscosity and storage modulus of these pastes reduced, which improved their 3D printing performance, such as hardness, support force, and plasticization (Qiu et al. Citation2023). Since the viscosity reduced as the shear rate increased, the doughs containing 25% (w/v) of SPI with 10, 20, and 30 wt% red cabbage (RC) had a shear thinning characteristic, an appropriate rheological behavior for 3D printing. Additionally, the texture profile study revealed that the addition of RC had a negligible opposing influence on the hardness, gumminess, and chewiness of the hydrated samples as infill increased (Carranza et al. Citation2023).
Additionally, to produce the prefabricated mold-based and 3D-printed scaffolds for the cultivation of bovine satellite cells (BSC), Ianovici et al. (Citation2022) evaluated mixtures of PPI and SPI with RGD-modified alginate (RGD-alginate) as potential bio-inks for cellular printing. They demonstrated that scaffolds composed of mold-based proteins exhibited increased stability and stiffness in comparison to those made only of RGD-alginate while permitting unrestricted BSC propagation and maturation. The two formulations were then combined to create extrusion-based 3D printing with an edible, removably attached agar support bath. In two cultivation configurations, cell recovery post-printing reached ∼80–90% viability over time (Ianovici et al. Citation2022). Moreover, fish analogs were produced using SPI, where fish analogs with a similar texture to real fish were obtained by regulating structure (15.86% porosity in 200 filaments) using 20 wt% of SPI, 4 wt% of xanthan gum, and 15 wt% rice starch (Shi et al. Citation2023b). In addition, the physicochemical and textural characteristics of hybrid meat analogs made from pea protein and chicken that were 3D printed were investigated. The outcomes demonstrated that both the hybrid and PPI-only cooked meat analogs had a moisture level of about 70%, which was comparable to that of chicken mince. However, the amount of chicken in the hybrid paste that underwent 3D printing and cooking considerably boosted the protein content. The hardness values of the 3D-printed cooked pastes and their non-printed cooked counterparts were significantly different, indicating that the 3D printing process reduces the hardness of the samples and is an effective way to produce a soft meal with significant applications in the care of the elderly (Wang et al. Citation2023d).
Nutrient supplements and food additives
Proteins are a crucial dietary supplement in the human diet, where dietary supplements provide extra amounts of nutrients to food to make it more nutrient-dense. Proteins serve a variety of functional purposes, including bone health maintenance, aging-related muscle gain, weight management, and nutrient supplementation (Alu’datt et al. Citation2016; Liu et al. Citation2023; Kumar et al. Citation2022a; Kritikos et al. Citation2021). Proteins are bioactive substances that serve as the foundation of the immune system. Proteins are crucial in the management of cardiovascular health and the prevention of many illnesses. Plant proteins help lower cholesterol, maintain bone health, increase muscle mass in older adults, and meet the protein needs of athletes. As a result, proteins can be employed as a vital supplement in the human diet to preserve the health of people of all ages. Legumes (chickpea, cowpea, soybean, pea, lupin), cereals (wheat, rice, sorghum, minor millets, maize, barley), pseudocereals (amaranth, buckwheat, and quinoa), seeds (sunflower, pumpkin, sesame, flaxseed), and various dry fruits have all been studied for use as protein supplements (Audu and Aremu Citation2011; Sengupta et al. Citation2016; Hadidi, Aghababaei, et al. Citation2023; Rashwan, Osman, and Chen Citation2023). For instance, sunflower meal protein isolate (SMPI) with low levels of caffeic acid and chlorogenic acid was used to make functional wheat bread (at 8–12% of the total wheat flour). In comparison to the control bread, the enriched bread had a higher mass volume and nutritious quality, where 10% was found to be the ideal amount of SMPI to add to the dough formula without significantly changing the color of the finished loaf (Shchekoldina and Aider Citation2014). Additionally, PPI was used to develop eggless cakes suitable for Lacto-vegetarians, where isolated pea protein (PPI), xanthan gum (XN), and emulsifier mixtures were used to prepare eggless cakes. The results demonstrated that the eggless cake formulation that contained PPI, 0.1% XN, and 1% soy lecithin (SL) was found to be close to the control traditional cakes in terms of specific gravity (1.01 vs. 1.03), crumb color (a* at 0.09 vs. −0.04), and crumb pore properties (average pore area both 0.20 mm2) (Lin et al. Citation2017). A randomized trial study by Lynch et al. (Citation2020) found no significant differences in muscle growth and strength development in men and women who consumed soy and whey protein supplements matched for leucine after a 12-week resistance training program. Moreover, consuming soy protein supplements to boost daily protein intake to 1.5 g/kg prevents field performance declines during subsequent speed-endurance training sessions without affecting exercise-induced muscle damage or redox status markers in professional male soccer players (Kritikos et al. Citation2021).
Synthesis of nanoparticles
With the unique properties of PBPs, including amphiphilic properties, inherent abundance, biodegradability, biocompatibility, and balanced amino acid profile that promotes the protein-nutraceutical interaction, proteins are considered a suitable choice for preparing various encapsulation systems (nanoparticles). In which proteins can interact effectively with both the solvent and the encapsulated compounds. Several isolated proteins such as soy protein, wheat protein (gluten and gliadin), pea protein, corn protein (zein), and sorghum protein (kafirin) have been used to produce and/or improve the nano-carriers, including Pickering emulsions, nano-gel, nanofibrils, complex coacervates, nanocrystal composite, and others (Liu et al. Citation2021; Pu et al. Citation2020; Wei et al. Citation2020; Wang et al. Citation2020; Fu et al. Citation2019; Li et al. Citation2023). For example, biopolymer particles formed by wheat gluten nanoparticles (WGN), or wheat gluten nanoparticle-xanthan gum (WGN-XG) complexes were used to stabilize Pickering emulsions for encapsulating β-carotene. The initial mean particle diameters of the WGN-XG emulsions were higher (23.9 μm) than those of the WGN ones (9.4 μm), but they were still resistant to aggregation over a wide range of pH (4–8) and salt concentrations (0–1000 mM NaCl). Additionally, they were successful in preventing the chemical deterioration of β-carotene during storage, with roughly 94.3% and 70.1% of the carotenoids remaining after one-month storage at 25 and 37 °C, respectively. Both the WGN-XG and WGN emulsions could be thermally sterilized. The bioaccessibility of β-carotene was higher in the WGN-XG emulsions than the WGN ones, according to an in vitro digestion experiment (Fu et al. Citation2019). The authors prepared hollow kafirin nanoparticles via gallic acid crosslinking to encapsulate resveratrol, and they showed that the nanoparticles formed were anionic spheres with an average diameter of <100 nm when loading amounts of resveratrol were less than 20%. After lyophilization, the hollow nanoparticles maintained their homogeneity and stable colloidal dispersion. The hollow nanoparticles crosslinked with gallic acid demonstrated stability against pancreatin and delayed release in stimulated digestion (Pu et al. Citation2020). Soy protein isolate (SPI) and cellulose nanocrystals (CNC) were employed as polymer matrices to create unique complex nanoparticles that served as carriers for the delivery of curcumin. At a mass ratio of 6:1, SPI-CNC complex nanoparticles had a relatively small size (197.7 ± 0.2 nm) and low polydispersity index (0.14). The nano-system was largely stable under a range of pH (3–9), temperature (30–90 °C), and salt concentrations (0–40 mmol/L) conditions. In addition, the SPI-CNC complex nanoparticles displayed a high encapsulation efficiency (88.3%) and sustained release during simulated gastrointestinal digestion (Wang et al. Citation2020). Moreover, core-shell pea protein-carboxymethylated corn fiber gum composite nanoparticles were used as delivery vehicles for curcumin. The results noted that the nanocomposite exhibited an excellent encapsulation performance for curcumin with high curcumin loading efficiency, good water dispersibility, and high chemical and thermal stability. Besides, the curcumin-loaded core-shell nanocomposite also showed higher antioxidant and radical scavenging activities than the free-curcumin (Wei et al. Citation2020). Additionally, Liu et al. (Citation2021) successfully prepared zein nanoparticles by stabilizing them using whey protein nanofibrils, where the hydrodynamic diameter and zeta potential of the nanoparticles were 413 nm and − 25 mV, respectively. In summary, these findings may provide new insight into the development of food-grade nanoparticles from PBPs suitable for the formulation of functional foods.
Bioplastics and packaging films
PBPs and plant waste protein, including soy protein, corn protein (zein), wheat gluten, cottonseed protein, sorghum protein (kafirin), and so on have emerged as a new class of green and eco-friendly materials, which can be used to produce bioplastics that also known as second-generation bioplastics (Patnode et al. Citation2021; Taylor and Taylor Citation2023). These second-generation bioplastics are potential substitutes for petroleum-based plastics because they are inexpensive, easy to produce, easily adaptable, and abundant in natural resources. These factors have led to their consideration in a variety of environmentally sensitive industries, such as agriculture (such as mulch films, greenhouse films, flowerpots, planting pots, etc.), packaging (one-time use before disposal), and the biomedical sector (Yamada et al. Citation2020; Jiménez-Rosado et al. Citation2019). For example, the authors successfully prepared films experimentally based on both modified soy protein and zein protein, which exhibit promising physical and mechanical behaviors. They added natural additives to plant proteins of varying chemical structures to obtain protein-based films with different properties. They found that adding glycerol and sorbitol as natural plasticizers, as well as micro-fibrillated cellulose as a reinforcement agent into protein systems, improved the film flexibility (elongation 2–120%) with Young’s modulus of 99–400 MPa, and the produced films had a higher surface hydrophobicity (Patnode et al. Citation2021). After buffer immersion, the films made from quality protein maize zein cast from glacial acetic acid were less transparent, while they absorbed the least liquid (≈16%) and swelled less (≈11%) compared to other zein films and aqueous ethanol-cast films. Besides, these films became highly flexible after ambient storage, whereas the other zein films remained brittle. The cross-linking process involving the cysteine-rich γ-zein polypeptides is thought to be the cause of the poor buffer uptake and swelling of quality protein maize zein films.
Additionally, the flexibility of these films is due to the zein’s improved solubilization in glacial acetic acid and the plasticizing effects of water molecules attached to the γ-zein polypeptides (Baloyi, Taylor, and Taylor Citation2023). Furthermore, Taylor and Taylor (Citation2023) reported that the total kafirin films (α-, β-, and γ-kafirins) were stronger and took up less moisture than commercial zein films (essentially α-zein), while both total kafirin- and total zein films showed a similar moisture uptake. Moreover, total kafirin films showed little difference in oxygen barrier properties with commercial zein films. Total kafirin elastomers also exhibited similar elastic recovery to gluten and better elastic recovery than that from commercial- and total zein elastomers. The better functional properties of bioplastics made from kafirin compared to bioplastics made from commercial zein could be owing to the greater disulfide bonded polymerization of kafirin polypeptides, involving the cysteine-rich β- and γ-kafirin classes (Taylor and Taylor Citation2023).
Fruits with varied respiratory metabolisms can be preserved for a longer period using lignocellulose nanofibers (LCNF)-wheat gluten (WG) hybrid coatings on their surfaces and hybrid packaging films. The multifunctional LCNF-WG coating and packaging demonstrated excellent barrier properties to oxygen ((15.9 cm3 μm m−2 day−1 kPa−1)) and water vapor (10.3 g mm−1 m−2 day−1) as well as excellent UV blocking, water-resistant, reusable, and recoverability performance (Chen et al. Citation2022b). The LCNF-WG hybrid film displayed only 4.3% water solubility. Interestingly, the LCNF-WG film degraded after five days of being buried in soil, showing high degradability and less negative impact on the environment (Chen et al. Citation2022b). Additionally, a film-forming solution (FFS) based on soy protein isolate (SPI) was studied by Jiang et al. (Citation2023) to determine how cysteine concentration affected the viscosity and physicochemical characteristics of SPI films. The results revealed that after adding 1 mmol/L cysteine, the apparent viscosity of FFS decreased; however, adding 2–8 mmol/L cysteine had little effect. The film solubility dropped from 70.40% to 57.60% following treatment with 1 mmol/L cysteine, but the other physical parameters remained unchanged. As cysteine content rose from 4 mmol/L to 8 mmol/L, the water vapor permeability and contact angle of SPI films increased while the film elongation at break decreased. According to studies from X-ray diffraction and scanning electron microscopy, cysteine crystallization may gather on the surface of SPI films treated with 4 or 8 mmol/L cysteine (Jiang et al. Citation2023). In conclusion, previous studies confirmed that PBPs showed excellent properties, making it a promising economic alternative in food preservation with the natural-derived material.
Potential health effects of plant-based protein and its products
Protein malnutrition has a strong negative impact on the body’s metabolism, composition, functioning, and clinical outcomes due to a lack or imbalance in dietary proteins. Although it is uncommon in developed countries, protein malnutrition is still the main cause of childhood mortality and morbidity worldwide. Many studies on PBPs products have demonstrated that they contain antioxidant compounds, act as tyrosine kinase protein inhibitors, regulate cell life and death, reduce lipid and bile acid absorption from the gastrointestinal system, and improve anti-neoplastic enzyme activity. These interactions are what give plant proteins their beneficial effects on human health. Following that, research on plant proteins sparked other studies in medicine and dietetics to show health benefits like antioxidant, antidiabetic, antimicrobial, anticancer, and others (). Scientific advancements have now led to the creation of food products that mix PBPs into normal meals to enhance the quality of life (Rashwan et al. Citation2021; Rashwan, Osman, and Chen Citation2023; Wang et al. Citation2023c; Aydar et al. Citation2023; Liu et al. Citation2023; Fawzi et al. Citation2022; Shi et al. Citation2023a; Vilcacundo et al. Citation2018).
Antioxidant and anti-inflammatory activities
DPPH (2,2-diphenyl-1-picrylhydrazyl) radical scavenging activity assay showed that gluten-free cookies prepared from raw and germinated amaranth grain flour exhibited the highest antioxidant activity (21.43 g/100 g) (Chauhan, Saxena, and Singh Citation2015). One of the several metabolic changes that occur because of seed sprouting is an increase in antioxidant activity. This is primarily caused by an increase in the activity of the endogenous hydrolytic enzymes (Chauhan, Saxena, and Singh Citation2015). Analysis of residues obtained after protein isolation revealed high phenolic contents of the bound form in flaxseed (56–62%) and free form in soybean (59–85%), indicating that the extracted bound phenolics from residue remaining after protein extraction had antioxidant activities ranging from 27 to 34% for full-fat soybean residue and 18–24% for full-fat flaxseed residue (Alu’datt et al. Citation2016). Additionally, the antioxidant activity analysis of pea protein irradiated by electron beam showed that hydrolysates pre-irradiated at 50 kGy have the strongest scavenging effect, with the DPPH radical and ABTS+ radical scavenging activity levels increasing by 32.73% and 52.80%, respectively (Wang et al. Citation2017b). This increase in antioxidant activity of pea proteins after pretreatment with electron beam irradiation may be due to the influence of electron beam irradiation on protein structure, where the energy generated by an electron beam accelerator induces protein unfolding and scission, exposing more peptide bonds to proteolytic enzymes and hydrolyzing polypeptide chains, thereby increasing pea protein antioxidant activity (Wang et al. Citation2017b).
Moreover, Caco-2 cells were used to assess the antioxidant activity of navy bean and light red kidney bean milk or yogurt after in vitro gastrointestinal digestion. For both navy and light red kidney beans, as well as milk and yogurts, fractions with MW <10 kDa demonstrated considerably higher antioxidant activity than fractions with MW 10–50 kDa. Furthermore, the cellular antioxidant activity of both NB and LKB digestates (MW < 10 kDa) was high (Chen, Zhang, et al. Citation2019). When zein nanoparticles were stabilized by the formation of whey protein nanofibrils between whey protein nanofibrils and zein, the antioxidant activity of whey protein nanofibrils was further increased (DPPH• scavenging rate improved by 39.82%, and the reducing power improved 144.38% compared to when zein: whey protein nanofibrils ratio was 1:0.6). However, the addition of more whey protein nanofibrils somewhat increased the antioxidant activity of the stabilized zein nanoparticles and was associated by a decrease in reducing power (Liu et al. Citation2021). According to the hypothesis, the whey protein nanofibril shell played a major role in the antioxidant activity of the zein nanoparticles that whey protein nanofibrils stabilized, and increasing the amount of whey protein nanofibrils will increase the antioxidant activity of the nanoparticles. Consequently, the increasing particle size may be the cause of the antioxidant activity’s stalling. A colloid system’s specific surface area may decrease because of the increased particle size of nanoparticles, which in turn lowers the amount of active groups on the shell’s surface that can interact with free radicals (Liu et al. Citation2021). In addition, the total antioxidant capacity bio-accessibility of kidney bean (Phaseolus vulgaris L.) milk substitute as a novel plant-based drink was 154%–295% by cupric reducing antioxidant capacity (CUPRAC) and 50%–186% by DPPH• method while the total phenolic content bio-accessibility varied between 207%–266% (Aydar et al. Citation2023). Functional soy-based yogurt incorporated with scent leaf essential oil microcapsules showed a high content of flavonoids (0.35 mg/100 g) and phenolics (5.94mgGAE/g), and thus high antioxidant activity, including FRAP (27.51 mg/100 g), and DPPH (56.88%) (Olabiran, Awolu, and Ayo-Omogie Citation2023).
Probiotic common bean (Phaseolus vulga L.) yogurts showed strong anti-inflammatory activity as demonstrated in suppressed tumor necrosis factor-induced interleukin-8 secretion in Caco-2 and HT-29 cell lines compared to their corresponding milk (Chen, Zhang, et al. Citation2019). Feeding high-fat-diet and streptozotocin-induced type 2 diabetic mice on sprouted quinoa yogurt significantly decreased the level of pro-inflammatory cytokines (TNF-α, IL-6, and IL-1β) and increased the level of anti-inflammatory cytokine IL-10. Histopathological studies also showed that QY protected the tissue structure of the liver of T2DM mice. Immunohistochemistry showed that QY increased AKT-2 and AMPK-α2 expressions while it suppressed p85 (Obaroakpo et al. Citation2020). Additionally, TNF-α, IL-1β, and IL-6 are examples of pro-inflammatory cytokines that can be decreased by quinoa yogurt. It can also suppress endotoxemia and systemic inflammation. Furthermore, when animals on the HFD were given quinoa yogurt and quinoa, endotoxemia and systemic inflammation were returned to the levels seen in control mice (Shi et al. Citation2023a).
Antidiabetic and antiobesity activities
Superoxide dismutase and catalase levels in liver homogenate increased after 8 wk of administration of bio-fortified soy yogurt at a dose of 2.5 g/kg body weight in Swiss albino male mice with hypercholesterolemia, and the serum activities of alanine aminotransferase, aspartate aminotransferase, alkaline phosphatase, acid phosphatase, and gamma-glutamyl significantly decreased (Sengupta et al. Citation2016). In diabetic rats given alloxan, the authors investigated the potential antidiabetic effects of fermented soy milk and flaxseed milk together. Researchers found that oral administration of fermented soy and flaxseed milk (FSFM) at low and high doses dramatically reduced blood glucose levels in rats that were neither normoglycemic nor alloxan-induced hyperglycemic, while also increasing insulin secretion like metformin dosage. Hematological changes were not evident in any of the groups. After receiving FSFM medication for the duration of trial, the body weight and feed consumption of the diabetes-induced rats increased in comparison to the normal and diabetic control group (Veerichetty Citation2018). The antidiabetic activity of FSFM may be correlated to the growth of microorganisms (probiotics containing Bifidobacterium and Streptococcus thermophilus) which have a strong ability to hydrolyze isoflavone glycosides and produce an ample quantity of isoflavone aglycones that known by their strong activity against diabetic enzymes (Ruiz de la Bastida et al. Citation2023; Peng et al. Citation2022). The evaluation of the inhibitory effects of the fermented quinoa and soy-based yogurt on digestive enzymes, including α-amylase, α-glucosidase, and pancreatic lipase showed that the digestive enzyme inhibition activities of the fermented yogurt occurred at the substrate ratio of 2:8 in the following order: α-amylase > α-glucosidase > pancreatic lipase, and α-amylase > pancreatic lipase > α-glucosidase at the ratio of 8:2 (Huang et al. Citation2022). The discrepancies in lipase inhibitions that have been found could potentially be primarily due to different phenolic chemical types. It demonstrated that the maximum inhibition rate was displayed by the yogurt with a substrate ratio of 2:8, followed by 0:10, 6:4, and 2:8. Other fermented soy-based products were also shown to have lipase inhibitory actions that were greatly improved by fermentation, in addition to plain quinoa yogurt (Lee et al. Citation2018). Based on an in vitro investigation, quinoa yogurt (QY) possesses anti-diabetic qualities. This was confirmed by looking at how high-fat diet/streptozotocin-induced type 2 diabetes mellitus (T2DM) mice responded to QY's hypoglycemic and hypolipidemic effects. The outcomes demonstrated that QY enhanced the body weights of T2DM mice and decreased fasting blood glucose levels. The serum levels of total cholesterol, triglycerides, and low-density lipoprotein cholesterol were all markedly decreased by QY, whereas the level of high-density lipoprotein cholesterol was raised. The amount of hepatic glycogen was also much higher, as were the activities of superoxide dismutase, catalase, and glutathione peroxidase, while lipid peroxidation was noticeably decreased (Obaroakpo et al. Citation2020). In which the qRT-PCR analysis indicated that QY exerted its hypoglycemic and anti-hyperlipidemic effects through the AKT/AMPK/PI3K signaling pathway (Obaroakpo et al. Citation2020). Besides, yogurt made from quinoa has been shown to drastically lower body weight increase and fat tissue weight in obese rats fed a high-fat diet (HFD). Additionally, quinoa yogurt dramatically improved insulin sensitivity, glucose homeostasis, and hepatic steatosis (Shi et al. Citation2023a). In quinoa yogurt fermented with commercial starter (CS) and W. confusa (WC), which had the strongest inhibitory efficacy, both α-amylase and α-glucosidase were inhibited at a rate of more than 70%. When rats were given several types of quinoa yogurt, their blood sugar levels were at their highest 0.5 h later. When mice were fed CS + WC, unfermented sample, or CS, the blood glucose levels were only 5.8 mmol/L, 6.7 mmol/L, and 7.5 mmol/L, respectively (Liu et al. Citation2023).
Antimicrobial and anticancer activities
Novel peptides were isolated from protein hydrolysates of RuBisCO purified from green juice alfalfa and showed antibacterial activity against Escherichia coli (E. coli), Salmonella enterica, Staphylococcus aureus, Enterococcus faecalis, Micrococcus luteus, and Bacillus subtilis, with distinct growth inhibition zones of higher than 10 mm (Kobbi et al. Citation2015). Banana flower protein’s antibacterial properties were examined utilizing the Mueller-Hinton agar medium and the well-diffusion method. The diameter of the inhibitory zone was used to interpret the findings. Significant antibacterial activity was demonstrated by the alkaline extract of banana flowers against both gram-positive and gram-negative bacteria. At lower tested doses (5 and 10 mg/ml), S. aureus was more responsive to extracted protein than Escherichia coli. At a dosage of 25 mg/ml with an inhibition zone of 14.30 mm, the extract was efficient against E. coli; however, at 5 and 10 mg/ml, there was no inhibition for E. coli. At doses of 25, 10, and 5 mg/ml, the banana flower protein extract inhibited Staphylococcus aureus with inhibition diameters of 12.70, 11.64, and 8.84 mm, respectively (Sitthiya et al. Citation2018). Additionally, the antimicrobial effect of LCNF-WG hybrid film was evaluated against the E. coli strain, and the results displayed that the bacteria titers dropped to zero after overnight incubation with LCNF-WG hybrid, LCNF, WG film, which suggested that the films effectively inhibited bacterial growth (Chen et al. Citation2022b).
The antimicrobial activity of proteins is basically due to the combination of the antimicrobial molecules (antimicrobial peptides (AMPs)) with the microorganisms’ membrane and/or cytoplasmic components, altering their cellular functions, which can lead to metabolism depletion or/and cellular death. This is caused by several properties of these molecules, which make it easier for them to insert into lipid bilayers and subsequently cause pore formation and cellular extravasation. These properties include their smaller size, electric charges, and composition of amino acids (Corrêa et al. Citation2019). Despite this general mechanism being relatively widespread, recent studies have countered this information, claiming that pore formation is not the only mechanism of action of AMPs. In which the increasing numbers of these observations suggest these peptides may also inhibit processes of the nucleic acids, cell wall, and protein synthesis, besides inhibiting several other enzymatic functions of the target cells (Sharma et al. Citation2022). Peptides have been reported to interact with cell membranes as part of their action against microbes, where the peptides have a tendency toward discrete channel formations that disturb lipid bilayers due to the occurrence of carpet-like peptide bindings and specific peptide-lipid interactions, which results in phase separation, membrane detergent-like solubilization, and even peptide penetration into the cell while leaving the membrane intact (Chen et al. Citation2022b). Moreover, the antibacterial effects of peptides have typically been related to several agents, such as the load, structural diversity, hydrophobicity, and particular amino acid composition, such as histidine, arginine, proline, cysteines, and glycine. About 50% of antimicrobial peptides have hydrophobic residues. Antimicrobial peptides have alternating hydrophobic and cationic regions. The cationic component enables binding to the negatively charged polar heads of microbial agents’ membrane phospholipids. The hydrophobic components are subsequently utilized for their entry into the agent’s cell membrane, which weakens the water inlet and induces cell lysis (Kobbi et al. Citation2015; Shevkani et al. Citation2022).
Furthermore, Kannan et al. (Citation2008) found the < 5 kDa fraction of rice bran peptide hydrolyzates to have higher anticancer activity against HepG2 and Caco-2 cells than both the 5–10 and > 10 kDa fractions. Besides, the antioxidant and colon cancer cell inhibitory activity of quinoa protein concentrate throughout simulated gastrointestinal digestion was evaluated. The highest effects were observed in the digests obtained during the intestinal phase, with fractions containing peptides < 5 kDa as the main ones responsible for the antioxidant activity and peptides > 5 kDa showing the greatest anti-cancer effects (Vilcacundo et al. Citation2018). Rice-based yogurt fermented by lactic acid bacteria demonstrated the maximum cytotoxicity activity against CaCo-2 colon cancer cells, with IC50 values ranging from 107.8 to 167.8 g/mL, whereas it was not toxic (safe) on normal cell lines, with IC50 values ranging from 445.9 to 537.9 g/mL. This activity could be because of organic acids and exopolysaccharide activities, specifically rEPS (released exopolysaccharides) and cbEPS (cell-bound exopolysaccharides) (Fawzi et al. Citation2022). Moreover, nanoencapsulation of resveratrol via pea protein nanofibrils (RES-PPI) exhibited higher antiproliferative activities than free-resveratrol, with cell viabilities of 52.6% and 38.5% for RES (free) and RES-PPI nanofibrils complex at 20 μg/mL (Yi et al. Citation2022). The anticancer activity of plant proteins may be because of the anticancer peptides (ACPs) which are released from proteins during digestion, where these peptides combine with tumor cells via electrostatic interaction. They can penetrate the membrane once there is sufficient concentration on its surface. The cell membrane then begins to disintegrate and micellize. As a result, pore structure develops and permeates the membrane, eventually leading to cell death. Conversely, peptides can disrupt the integrity of the mitochondrial membrane once they’ve entered the cell cytoplasm, which can lead to the release of various mitochondrial proteins like cytochrome c, a potent inducer of apoptosis. After that, the injured mitochondria’s released cytochrome c causes caspase 9 activation, apoptosis-protease activating factor 1 oligomerization, and pro-caspase 3 conversion to caspase 3, which oversees numerous apoptotic events (Norouzi, Mirmohammadi, and Houshdar Tehrani Citation2022; Lath et al. Citation2023; Fawzi et al. Citation2022).
Challenges and opportunities of using PBPs in food processing
Plant protein-based products are becoming more and more popular because of recent marketing campaigns and product development initiatives, particularly non-meat and nondairy alternatives. However, this initial momentum is faced with numerous technological and consumerism challenges, where the attempt to simulate the sensory characteristics (texture and flavor) of animal products (meat and milk, yogurt, and cheese) is confirmed to be difficult, especially concerning the construction of muscle-like tissue in the meat products or color and taste in milk products (Sha and Xiong Citation2020). According to numerous recent surveys, consumers often regard the appearance, texture, mouthfeel, and flavor of such food to be key variables impacting their acceptance and consumption. Innovative product formulations and processing technology advancements will keep enhancing the qualities of animal proteins-like products. To replicate the texture, juiciness, mouthfeel, and flavor of animal proteins, a variety of additives are used, which presents questions about consumer confidence, clean labels, nutrition, and food safety (Graça, Godinho, and Truninger Citation2019). To mimic the flavors of processed meat, a variety of spices and herbs are added, including several that are also used in meat processing. Even yet, a lot of plant-based substitutes still have an aftertaste. The distinct smell associated with beany, which is believed to be linked to derivatives or products of secondary lipid oxidation like hexanal and methanethiol, as well as the naturally occurring bitter-astringent tastes resulting from the presence of saponins and isoflavones, may hinder the use of soy protein as the building blocks for meat substitutes. Therefore, research is required to lessen the influence of these unpleasant smells during the production of soy protein isolate or concentrate (Bohrer Citation2019; Yuan et al. Citation2021; Marc et al. Citation2022). According to the analysis of the nutritional makeup and formulation of seven widely used commercial substitute goods (hams, nuggets, and burgers), each item list indicated the presence of 20 to 30 additives. The topic of whether some plant-based alternatives are better and more nutritious than meat is raised by the numerous additives, excessive salt content, and saturated fat in some of the products (Bohrer Citation2019).
Besides, the consumption of PBPs and their products as human food has another large challenge which is the presence of some anti-nutritional factors including tannins, phytic acid, saponins, alkaloids, oxalate, trypsin inhibitors, protease inhibitors, and protein cross-linkers, etc. that have unfavorable consequences. These antinutritional factors may contain indigestible components that hinder the capacity of digestive enzymes to access the proteins, or they may contain substances that limit protein digestion or absorption (Rashwan et al. Citation2021; Ding et al. Citation2023). For instance, approximately 38 to 41% of the total phytate discovered in whole quinoa seeds was found in the bran, which had a greater phytate level than the flour or peel. Phytic acid values in five different quinoa varieties ranged from 10.5 to 13.1 mg/g. Besides, the phytic acid content in barley, corn, wheat, and sorghum grains ranged from 9.7 to 11.6, 8.9 to 9.9, 6.2 to 13.5, and 1.61 to 2.16 mg/g, respectively (Filho et al. Citation2017; Sorour et al. Citation2017). Moreover, the contents of trypsin inhibitors in eight varieties of quinoa ranged from 1.36 to 5.04 unit trypsin inhibitor (UTI)/mg protein, in soybean ranged from 24.5 to 41.5 UTI/mg, in beans ranged from 12.9 to 42.8 UTI/mg), and in lentils 8 to 17.8 UTI/mg (Filho et al. Citation2017). Therefore, producers of plant-based foods should consider the bioavailability of proteins to make sure that their products provide the necessary vital nutrients. Therefore, PBPs can be treated to improve their nutritional availability and functional properties by reducing the negative effects of those antinutritional factors through careful design of the processing operations used to isolate and purify the proteins, or/and using pretreatment including soaking, germination, fermentation, thermal processes, etc. In contrast, the protein ingredients should still be able to provide the necessary functional qualities, such as the ability to hold water and oil, emulsify and foam, bind, be soluble, and form gels without negative effects on the sensory attributes of final products (Shaghaghian et al. Citation2022; Rashwan, Osman, and Chen Citation2023; Rashwan et al. Citation2024). For example, Abbas and Ahmad (Citation2018) reported that treatment of legume seeds using the autoclave at 121 °C reduced tannins and phytic acid contents by 33.1 to 45.7, and 28 to 51.6%, respectively. Additionally, boiling Chickpea seeds for 90 min at 100 °C decreased trypsin inhibitor activity by 82.27%, tannins by 48.04%, phytic acid by 28.93%, and hemagglutinin by 100%, while soaking Mung bean at 25 °C for 12 h reduced trypsin inhibitor activity, tannins, phytic acid, and hemagglutinin by 15.8, 39.4, 26.7, and 49.1%, respectively. Using an extrusion microwave to treat Chickpea at high temperatures for 15 min led to a reduction of its content from trypsin inhibitor activity (80.5%), tannins (48.45%), phytic acid (38.02%), and hemagglutinin (100%) (Abbas and Ahmad Citation2018). Besides, fermentation of quinoa using Lactobacillus plantarum as a starter reduced its anti-nutritional components such saponin, alkaloids, oxalate, phytic acid, and tannins from 2.84, 0.73, 0.21, 1.35, and 0.49% to 2.04, 0.56, 0.18, 1.31, and 0.35%, respectively (Ibrahem and Mohamed Citation2021). Besides, probiotic-fermentation of quinoa seeds flour by Lactobacillus plantaram ATCC 14917 and Lactobacillus delbrueckii spp. Bulgaricus EMCC 11102 resulted in a decrease in phytate levels from 94.38 to 67.06 and from 94.38 to 71.88 mg/100 g fermented quinoa, respectively (Abdelshafy, El-Naggar, and Kenawi Citation2022b). The phytate and saponin contents in quinoa decreased from 11.06 to 3.02 mg/g and from 11.20 to 0.15 mg/g, respectively, after the fermentation process for four days at 30 °C by Lactobacillus bulgaricus, phytate, and saponin contents can be appropriately reduced through fermenting while maintaining the acceptable sensory properties of quinoa beverages (Jan et al. Citation2023).
Furthermore, the allergy to PBPs (induced by the allergenic proteins) such as celiac disease (triggered by the gluten proteins) is one of the most important side effects associated with the consumption of PBPs (Tan, Nawaz, and Buckow Citation2023). An allergic reaction occurs when the immune system attacks food proteins with symptoms ranging from mild and transient to severe and life-threatening (Sampson et al. Citation2014). Additionally, isoflavones represent another limitation in PBPS products produced by soy proteins (Hertzler et al. Citation2020). Isoflavone compounds have some elements in their chemical structure like estrogen hormones, and can weakly bind with estrogen receptors, resulting in endocrine disrupting and harmful effects on reproductive hormones (Hertzler et al. Citation2020; Messina Citation2016). Additionally, some circles in the nephrology and nutrition community indicated that PBPs have been viewed as nutritionally inadequate and even hazardous for the management of patients with chronic kidney disease (CKD). These concerns regarding proteins and amino acid deficiency with PBPs have precluded their consumption by CKD patients. However, many of these concerns were debunked years ago, but recommendations persist regarding the use of animal-based proteins in CKD patients, which may contribute to the worsening of other parameters such as hyperphosphatemia and blood pressure. On the other hand, many investigations stated that PBPs are adequate and safe for patients with CKD including those with proteinuria (Joshi, Shah, and Kalantar-Zadeh Citation2019).
Conclusion and future directions
Plant protein technology is a key area of biotechnology for addressing the issue of increasing protein demand by humans. Even though plants (proteins) are more plentiful and affordable than animal proteins in comparison, direct usage of PBPs is still limited because PBPs are fed to animals as feed to produce animal-based proteins like meat, eggs, and milk. The physicochemical and functional properties of PBPs, especially solubility, emulsifying abilities, and foaming are significantly affected by environmental factors, particularly pH and ionic strength. Electrostatic interactions are the main way for protein interactions, which can be used to create protein/polysaccharide complexes for food industrial purposes. The extraction yield of proteins can be reached up to 86–95% with high functional properties and from a sustainable and efficient route using enzymatic, ultrasound-, microwave-, pulsed electric field-, and high-pressure-assisted extraction. Nondairy alternative products, especially yogurts and cheese successfully produced from PBPs, which can be used as gluten-free and lactose-free products, as well as 3D food printing and meat analogs produced from PBPs that can be consumed by older people (especially with swallowing disorders), pregnant women, children, sports, and so on. Additionally, the synthesis of nanoparticles, bioplastics, and packaging films can be created from PBPs as green materials. Moreover, PBPs particularly those that contain pigments and their products showed good bioactivities, especially antioxidants, antidiabetic, and antimicrobial. Therefore, this review could be a useful piece of work on protein-rich plants and their products that may attract the attention of not only food researchers but also industrial individuals.
Authors’ contributions
Ahmed K. Rashwan: Conceptualization, Methodology, Software, Writing-original draft, Writing-review & editing. Ahmed I. Osman: Resources, Conceptualization, Writing-original draft, Writing-editing. Asem M. Abdelshafy: Writing-review & editing. Jianling Mo: Review & editing. Wei Chen: Conceptualization, Methodology, Supervision, Resources, Writing - review & editing.
Acknowledgements
Dr. Ahmed K. Rashwan would like to thank Zhejiang University and Zhejiang Province for providing a postdoctoral Fellowship position for him and supporting his research. Dr. Ahmed I. Osman wishes to acknowledge the support of The Bryden Centre project (Project ID VA5048), which was awarded by The European Union’s INTERREG VA Programme, managed by the Special EU Programmes Body (SEUPB), with match funding provided by the Department for the Economy in Northern Ireland and the Department of Business, Enterprise and Innovation in the Republic of Ireland.
Disclosure statement
The authors declare that there are no conflicts of interest.
Additional information
Funding
References
- Abbas, Y., and A. Ahmad. 2018. Impact of processing on nutritional and antinutritional factors of legumes: A review. Annals: Food Science & Technology 19:199–215.
- Abdelshafy, A. M., E. A. El-Naggar, and M. N. Kenawi. 2022a. Moringa leaves for improving the health benefits of quinoa fermented by probiotics. Food Bioengineering 1 (3–4):264–75. doi: 10.1002/fbe2.12035.
- Abdelshafy, A. M., E. A. El-Naggar, and M. N. Kenawi. 2022b. Enhancing of nutritional properties of quinoa fermented by probiotics. Discover Food 2 (1):21. doi: 10.1007/s44187-022-00022-8.
- Abugoch, L. E., N. Romero, C. A. Tapia, J. Silva, and M. Rivera. 2008. Study of Some Physicochemical and Functional Properties of Quinoa (Chenopodium Quinoa Willd) Protein Isolates. Journal of Agricultural and Food Chemistry 56 (12):4745–50. doi: 10.1021/jf703689u.
- Al-Gaby, A. M. A. 1998. Amino acid composition and biological effects of supplementing broad bean and corn proteins with Nigella sativa(black cumin) cake protein. Nahrung / Food 42 (05):290–4. doi: 10.1002/(SICI)1521-3803(199810)42:05 < 290::AID-FOOD290 > 3.0.CO;2-Y.
- Al-Ruwaih, N., J. Ahmed, M. F. Mulla, and Y. A. Arfat. 2019. High-pressure assisted enzymatic proteolysis of kidney beans protein isolates and characterization of hydrolysates by functional, structural, rheological and antioxidant properties. Lwt 100:231–6. doi: 10.1016/j.lwt.2018.10.074.
- Alu’datt, M. H., T. Rababah, M. N. Alhamad, S. Gammoh, K. Ereifej, S. Kubow, and I. Alli. 2016. Characterization and antioxidant activities of phenolic interactions identified in byproducts of soybean and flaxseed protein isolation. Food Hydrocolloids. 61:119–27. doi: 10.1016/j.foodhyd.2016.05.002.
- Amagliani, L., J. O'Regan, A. L. Kelly, and J. A. O'Mahony. 2017. The composition, extraction, functionality and applications of rice proteins: A review. Trends in Food Science & Technology 64:1–12. doi: 10.1016/j.tifs.2017.01.008.
- Ampofo, J., and M. Ngadi. 2022. Ultrasound-assisted processing: Science, technology and challenges for the plant-based protein industry. Ultrasonics Sonochemistry 84:105955. doi: 10.1016/j.ultsonch.2022.105955.
- Andersen, P. C., K. Hill, D. W. Gorbet, and B. V. Brodbeck. 1998. Fatty Acid and Amino Acid Profiles of Selected Peanut Cultivars and Breeding Lines. Journal of Food Composition and Analysis 11 (2):100–11. doi: 10.1006/jfca.1998.0565.
- Arise, A. K., D. O. Opaleke, K. O. Salami, G. V. Awolola, and D. F. Akinboro. 2020. Physico-chemical and sensory properties of a cheese-like product from the blend of soymilk and almond milk. Agrosearch 19 (2):54–63. doi: 10.4314/agrosh.v19i2.5.
- Aryee, F. N. A., and M. T. Nickerson. 2014. Effect of pH, biopolymer mixing ratio and salts on the formation and stability of electrostatic complexes formed within mixtures of lentil protein isolate and anionic polysaccharides (κ-carrageenan and gellan gum. International Journal of Food Science & Technology 49 (1):65–71. doi: 10.1111/ijfs.12275.
- Audu, S. S., and M. O. Aremu. 2011. Nutritional composition of raw and processed pinto bean (Phaseolus vulgaris L.) grown in Nigeria. Journal of Food, Agriculture & Environment 9:72–80. doi: 10.1234/4.2011.2226.
- Aydar, E. F., Z. Mertdinç, E. Demircan, S. Koca Çetinkaya, and B. Özçelik. 2023. Kidney bean (Phaseolus vulgaris L.) milk substitute as a novel plant-based drink: Fatty acid profile, antioxidant activity, in-vitro phenolic bio-accessibility and sensory characteristics. Innovative Food Science & Emerging Technologies 83:103254. doi: 10.1016/j.ifset.2022.103254.
- Baloyi, J. T., J. Taylor, and J. R. N. Taylor. 2023. Bioplastic film making properties of quality protein maize (QPM) zein. Cereal Chemistry 100 (4):805–15. doi: 10.1002/cche.10665.
- Banach, J. L., J. P. van der Berg, G. Kleter, H. van Bokhorst-van de Veen, S. Bastiaan-Net, L. Pouvreau, and E. D. van Asselt. 2022. Alternative proteins for meat and dairy replacers: Food safety and future trends. Critical Reviews in Food Science and Nutrition 1–18. doi: 10.1080/10408398.2022.2089625.
- Basha, S. M. 1992. Effect of location and season on peanut seed protein and polypeptide composition. Journal of Agricultural and Food Chemistry 40 (10):1784–8. doi: 10.1021/jf00022a011.
- Bedin, S., F. M. Netto, N. Bragagnolo, and O. P. Taranto. 2020. Reduction of the process time in the achieve of rice bran protein through ultrasound-assisted extraction and microwave-assisted extraction. Separation Science and Technology 55 (2):300–12. doi: 10.1080/01496395.2019.1577449.
- Bian, X., T.-L. Xing, Y. Yang, J. Fan, C.-M. Ma, X.-F. Liu, Y. Wang, Y.-Y. He, L.-D. Wang, B. Wang, et al. 2023. Effect of soy protein isolate on physical properties of quinoa dough and gluten-free bread quality characteristics. Journal of the Science of Food and Agriculture 103 (1):118–24. doi: 10.1002/jsfa.12118.
- Bohrer, B. M. 2019. An investigation of the formulation and nutritional composition of modern meat analogue products. Food Science and Human Wellness 8 (4):320–9. doi: 10.1016/j.fshw.2019.11.006.
- Bou, R., P. Navarro-Vozmediano, R. Domínguez, M. López-Gómez, M. Pinent, A. Ribas-Agustí, J. J. Benedito, J. M. Lorenzo, X. Terra, J. V. García-Pérez, et al. 2022. Application of emerging technologies to obtain legume protein isolates with improved techno-functional properties and health effects. Comprehensive Reviews in Food Science and Food Safety 21 (3):2200–32. doi: 10.1111/1541-4337.12936.
- Cabral, E. M., M. M. Poojary, M. N. Lund, J. Curtin, M. Fenelon, and B. K. Tiwari. 2022. Effect of solvent composition on the extraction of proteins from hemp oil processing stream. Journal of the Science of Food and Agriculture 102 (14):6293–8. doi: 10.1002/jsfa.11979.
- Carranza, T., P. Guerrero, K. d l Caba, and A. Etxabide. 2023. Texture-modified soy protein foods: 3D printing design and red cabbage effect. Food Hydrocolloids. 145:109141. doi: 10.1016/j.foodhyd.2023.109141.
- Cassini, A. S., I. C. Tessaro, L. D. F. Marczak, and C. Pertile. 2010. Ultrafiltration of wastewater from isolated soy protein production: A comparison of three UF membranes. Journal of Cleaner Production 18 (3):260–5. doi: 10.1016/j.jclepro.2009.10.016.
- Chang, L., Y. Lan, N. Bandillo, J.-B. Ohm, B. Chen, and J. Rao. 2022. Plant proteins from green pea and chickpea: Extraction, fractionation, structural characterization and functional properties. Food Hydrocolloids. 123:107165. doi: 10.1016/j.foodhyd.2021.107165.
- Chauhan, A., D. C. Saxena, and S. Singh. 2015. Total dietary fibre and antioxidant activity of gluten free cookies made from raw and germinated amaranth (Amaranthus spp.) flour. LWT - Food Science and Technology 63 (2):939–45. doi: 10.1016/j.lwt.2015.03.115.
- Chen, D., O. G. Jones, and O. H. Campanella. 2023. Plant protein-based fibers: Fabrication, characterization, and potential food applications. Critical Reviews in Food Science and Nutrition 63 (20):4554–78. doi: 10.1080/10408398.2021.2004991.
- Chen, J., T. Mu, D. Goffin, C. Blecker, G. Richard, A. Richel, and E. Haubruge. 2019. Application of soy protein isolate and hydrocolloids based mixtures as promising food material in 3D food printing. Journal of Food Engineering 261:76–86. doi: 10.1016/j.jfoodeng.2019.03.016.
- Chen, J., H. Sun, T. Mu, C. Blecker, A. Richel, G. Richard, N. Jacquet, E. Haubruge, and D. Goffin. 2022a. Effect of temperature on rheological, structural, and textural properties of soy protein isolate pastes for 3D food printing. Journal of Food Engineering 323:110917. doi: 10.1016/j.jfoodeng.2021.110917.
- Chen, Y., Y. Li, S. Qin, S. Han, and H. Qi. 2022b. Antimicrobial, UV blocking, water-resistant and degradable coatings and packaging films based on wheat gluten and lignocellulose for food preservation. Composites Part B: Engineering 238:109868. doi: 10.1016/j.compositesb.2022.109868.
- Chen, Y., H. Zhang, R. Liu, L. Mats, H. Zhu, K. P. Pauls, Z. Deng, and R. Tsao. 2019. Antioxidant and anti-inflammatory polyphenols and peptides of common bean (Phaseolus vulga L.) milk and yogurt in Caco-2 and HT-29 cell models. Journal of Functional Foods 53:125–35. doi: 10.1016/j.jff.2018.12.013.
- Coffmann, C. W., and V. V. Garciaj. 2007. Functional properties and amino acid content of a protein isolate from mung bean flour*. International Journal of Food Science & Technology 12 (5):473–84. doi: 10.1111/j.1365-2621.1977.tb00132.x.
- Corrêa, J. A. F., A. G. Evangelista, T. d M. Nazareth, and F. B. Luciano. 2019. Fundamentals on the molecular mechanism of action of antimicrobial peptides. Materialia 8:100494. doi: 10.1016/j.mtla.2019.100494.
- Cuq, B., F. Boutrot, A. Redl, and V. Lullien-Pellerin. 2000. Study of the temperature effect on the formation of wheat gluten network: Influence on mechanical properties and protein solubility. Journal of Agricultural and Food Chemistry 48 (7):2954–9. doi: 10.1021/jf991339b.
- D'Alessio, G., F. Flamminii, M. Faieta, R. Prete, A. Di Michele, P. Pittia, and C. D. Di Mattia. 2023. High pressure homogenization to boost the technological functionality of native pea proteins. Current Research in Food Science 6:100499. doi: 10.1016/j.crfs.2023.100499.
- da Silva, L. R., J. I. Velasco, and F. M. Fakhouri. 2023. Use of rice on the development of plant-based milk with antioxidant properties: From raw material to residue. Lwt 173:114271. doi: 10.1016/j.lwt.2022.114271.
- de Figueiredo, V. R. G., F. Yamashita, A. L. L. Vanzela, E. I. Ida, and L. E. Kurozawa. 2018. Action of multi-enzyme complex on protein extraction to obtain a protein concentrate from okara. Journal of Food Science and Technology 55 (4):1508–17. doi: 10.1007/s13197-018-3067-4.
- Di Domenico Ziero, H., L. S. Buller, A. Mudhoo, L. Castro Ampese, S. I. Mussatto, and T. Forster Carneiro. 2020. An overview of subcritical and supercritical water treatment of different biomasses for protein and amino acids production and recovery. Journal of Environmental Chemical Engineering 8 (5):104406. doi: 10.1016/j.jece.2020.104406.
- Ding, Y., Q. Ban, Y. Wu, Y. Sun, Z. Zhou, Q. Wang, J. Cheng, and H. Xiao. 2023. Effect of high hydrostatic pressure on the edible quality, health and safety attributes of plant-based foods represented by cereals and legumes: A review. Critical Reviews in Food Science and Nutrition 63 (20):4636–54. doi: 10.1080/10408398.2021.2005531.
- Doublier, J. L., C. Garnier, D. Renard, and C. Sanchez. 2000. Protein–polysaccharide interactions. Current Opinion in Colloid & Interface Science 5 (3-4):202–14. doi: 10.1016/S1359-0294(00)00054-6.
- Du, C., Z. Wang, and Z. Zheng. 2023. The preparation of plant-based milk substitutes with antioxidant properties using soybean protein isolate and curcumin composite nanoparticles. Lwt 182:114780. doi: 10.1016/j.lwt.2023.114780.
- Eckert, E., J. Han, K. Swallow, Z. Tian, M. Jarpa-Parra, and L. Chen. 2019. Effects of enzymatic hydrolysis and ultrafiltration on physicochemical and functional properties of faba bean protein. Cereal Chemistry 96 (4):725–41. doi: 10.1002/cche.10169.
- Elsohaimy, S. A., T. M. Refaay, and M. A. M. Zaytoun. 2015. Physicochemical and functional properties of quinoa protein isolate. Annals of Agricultural Sciences 60 (2):297–305. doi: 10.1016/j.aoas.2015.10.007.
- Fawzi, N. Y., D. Y. Abdelghani, M. A. Abdel-Azim, C. G. Shokier, M. W. Youssef, M. K. Gad El-Rab, A. I. Gad, and K. A. Abou-Taleb. 2022. The ability of probiotic lactic acid bacteria to ferment Egyptian broken rice milk and produce rice-based yoghurt. Annals of Agricultural Sciences 67 (1):107–18. doi: 10.1016/j.aoas.2022.06.004.
- Feyzi, S., M. Varidi, F. Zare, and M. J. Varidi. 2015. Fenugreek (Trigonella foenum graecum) seed protein isolate: Extraction optimization, amino acid composition, thermo and functional properties. Journal of the Science of Food and Agriculture 95 (15):3165–76. doi: 10.1002/jsfa.7056.
- Filho, A. M. M., M. R. Pirozi, J. T. D. S. Borges, H. M. Pinheiro Sant’Ana, J. B. P. Chaves, and J. S. D. R. Coimbra. 2017. Quinoa: Nutritional, functional, and antinutritional aspects. Critical Reviews in Food Science and Nutrition 57 (8):1618–30. doi: 10.1080/10408398.2014.1001811.
- Flores-Sosa, Á. R., E. N. Aquino-Bolaños, A. Cardador-Martínez, J. L. Chávez-Servia, A. M. Vera-Guzmán, J. C. Carrillo-Rodríguez, and J. E. A. Jiménez. 2020. Variation in protein and amino acids content among landraces of common bean (Phaseolus vulgaris L.). Emirates Journal of Food and Agriculture 32:750–60. doi: 10.9755/ejfa.2020.v32.i10.2175.
- Fu, D., S. Deng, D. J. McClements, L. Zhou, L. Zou, J. Yi, C. Liu, and W. Liu. 2019. Encapsulation of β-carotene in wheat gluten nanoparticle-xanthan gum-stabilized Pickering emulsions: Enhancement of carotenoid stability and bioaccessibility. Food Hydrocolloids. 89:80–9. doi: 10.1016/j.foodhyd.2018.10.032.
- Gentile, L. 2020. Protein–polysaccharide interactions and aggregates in food formulations. Current Opinion in Colloid & Interface Science 48:18–27. doi: 10.1016/j.cocis.2020.03.002.
- Graça, J., C. A. Godinho, and M. Truninger. 2019. Reducing meat consumption and following plant-based diets: Current evidence and future directions to inform integrated transitions. Trends in Food Science & Technology 91:380–90. doi: 10.1016/j.tifs.2019.07.046.
- Günal-Köroğlu, D., J. M. Lorenzo, and E. Capanoglu. 2023. Plant-Based Protein-Phenolic Interactions: Effect on different matrices and in vitro gastrointestinal digestion. Food Research International (Ottawa, Ont.) 173 (Pt 1):113269. doi: 10.1016/j.foodres.2023.113269.
- Guzmán-Lorite, M., M. L. Marina, and M. C. García. 2022. Successive extraction using natural deep eutectic solvents and pressurized liquids for a greener and holistic recovery of proteins from pomegranate seeds. Food Research International (Ottawa, Ont.) 161:111862. doi: 10.1016/j.foodres.2022.111862.
- Guzmán‐Maldonado, S. H., J. Acosta-Gallegos, and O. Paredes‐López. 2000. Protein and mineral content of a novel collection of wild and weedy common bean (Phaseolus vulgaris L). Journal of the Science of Food and Agriculture 80 (13):1874–81. doi: 10.1002/1097-0010(200010)80:13 < 1874::AID-JSFA722 > 3.0.CO;2-X.
- Hadidi, M., F. Aghababaei, and D. J. McClements. 2023. Enhanced alkaline extraction techniques for isolating and modifying plant-based proteins. Food Hydrocolloids. 145:109132. doi: 10.1016/j.foodhyd.2023.109132.
- Hadidi, M., J. C. Orellana Palacios, D. J. McClements, M. Mahfouzi, and A. Moreno. 2023. Alfalfa as a sustainable source of plant-based food proteins. Trends in Food Science & Technology 135:202–14. doi: 10.1016/j.tifs.2023.03.023.
- Halim, J. K., G. H. Wangrimen, and A. Fitriani. 2022. Production of coconut milk cheese and its organoleptic characteristics. Journal of Agri-Food Science and Technology 3 (1):1–9. doi: 10.12928/jafost.v3i1.6219.
- Hendawey, M. H., and A. M. A. Younes. 2013. Biochemical evaluation of some faba bean cultivars under rainfed conditions at El-Sheikh Zuwayid. Annals of Agricultural Sciences 58 (2):183–93. doi: 10.1016/j.aoas.2013.07.010.
- Hernández-Corroto, E., M. Plaza, M. L. Marina, and M. C. García. 2020. Sustainable extraction of proteins and bioactive substances from pomegranate peel (Punica granatum L.) using pressurized liquids and deep eutectic solvents. Innovative Food Science & Emerging Technologies 60:102314. doi: 10.1016/j.ifset.2020.102314.
- Hertzler, S. R., J. C. Lieblein-Boff, M. Weiler, and C. Allgeier. 2020. Plant Proteins: Assessing Their Nutritional Quality and Effects on Health and Physical Function. Nutrients 12 (12):3704. doi: 10.3390/nu12123704.
- Huang, K., Y. Liu, Y. Zhang, H. Cao, D-k Luo, C. Yi, and X. Guan. 2022. Formulation of plant-based yoghurt from soybean and quinoa and evaluation of physicochemical, rheological, sensory and functional properties. Food Bioscience 49:101831. doi: 10.1016/j.fbio.2022.101831.
- Ianovici, I., Y. Zagury, I. Redenski, N. Lavon, and S. Levenberg. 2022. 3D-printable plant protein-enriched scaffolds for cultivated meat development. Biomaterials 284:121487. doi: 10.1016/j.biomaterials.2022.121487.
- Ibrahem, E. S., and G. H. Mohamed. 2021. Effect of different preparatory processes on improving the nutritional value of quinoa (Chenopodium quinoa, Willd) and studying its effect on obese Anemic rats. Egyptian Journal of Nutrition and Health 16 (2):71–94. doi: 10.21608/ejnh.2021.239553.
- Iqbal, A., N. Ateeq, I. A. Khalil, S. Perveen, and S. Saleemullah. 2006. Physicochemical characteristics and amino acid profile of chickpea cultivars grown in Pakistan. Journal of Foodservice 17 (2):94–101. doi: 10.1111/j.1745-4506.2006.00024.x.
- Jan, N., S. Z. Hussain, B. Naseer, and T. A. Bhat. 2023. Amaranth and quinoa as potential nutraceuticals: A review of anti-nutritional factors, health benefits and their applications in food, medicinal and cosmetic sectors. Food Chemistry: X 18:100687. doi: 10.1016/j.fochx.2023.100687.
- Jiang, J., L. Shi, Z. Ren, and W. Weng. 2023. Preparation and characterization of soy protein isolate films by pretreatment with cysteine. Food Chemistry: X 18:100735. doi: 10.1016/j.fochx.2023.100735.
- Jiménez-Rosado, M., L. S. Zarate-Ramírez, A. Romero, C. Bengoechea, P. Partal, and A. Guerrero. 2019. Bioplastics based on wheat gluten processed by extrusion. Journal of Cleaner Production 239:117994. doi: 10.1016/j.jclepro.2019.117994.
- Joshi, S., S. Shah, and K. Kalantar-Zadeh. 2019. Adequacy of Plant-Based Proteins in Chronic Kidney Disease. Journal of Renal Nutrition: The Official Journal of the Council on Renal Nutrition of the National Kidney Foundation 29 (2):112–7. doi: 10.1053/j.jrn.2018.06.006.
- Juliano, B. O., A. A. Antonio, and B. V. Esmama. 1973. Effects of protein content on the distribution and properties of rice protein. Journal of the Science of Food and Agriculture 24 (3):295–306. doi: 10.1002/jsfa.2740240306.
- Kalman, D. S. 2014. Amino acid composition of an organic brown rice protein concentrate and isolate compared to soy and whey concentrates and isolates. Foods (Basel, Switzerland) 3 (3):394–402. doi: 10.3390/foods3030394.
- Kannan, A., N. Hettiarachchy, M. G. Johnson, and R. Nannapaneni. 2008. Human Colon and Liver Cancer Cell Proliferation Inhibition by Peptide Hydrolysates Derived from Heat-Stabilized Defatted Rice Bran. Journal of Agricultural and Food Chemistry 56 (24):11643–7. doi: 10.1021/jf802558v.
- Kobbi, S., R. Balti, A. Bougatef, G. Le Flem, L. Firdaous, M. Bigan, G. Chataigné, S. Chaabouni, P. Dhulster, and N. Nedjar. 2015. Antibacterial activity of novel peptides isolated from protein hydrolysates of RuBisCO purified from green juice alfalfa. Journal of Functional Foods 18:703–13. doi: 10.1016/j.jff.2015.09.007.
- Koysuren, B., M. H. Oztop, and B. G. Mazi. 2021. Sesame seed as an alternative plant protein source: A comprehensive physicochemical characterisation study for alkaline, salt and enzyme-assisted extracted samples. International Journal of Food Science & Technology 56 (11):5471–84. doi: 10.1111/ijfs.15229.
- Kritikos, S., K. Papanikolaou, D. Draganidis, A. Poulios, K. Georgakouli, P. Tsimeas, T. Tzatzakis, D. Batsilas, A. Batrakoulis, C. K. Deli, et al. 2021. Effect of whey vs. soy protein supplementation on recovery kinetics following speed endurance training in competitive male soccer players: A randomized controlled trial. Journal of the International Society of Sports Nutrition 18 (1):23. doi: 10.1186/s12970-021-00420-w.
- Kumar, M., M. Tomar, J. Potkule, R. Verma, S. Punia, A. Mahapatra, T. Belwal, A. Dahuja, S. Joshi, M. K. Berwal, et al. 2021. Advances in the plant protein extraction: Mechanism and recommendations. Food Hydrocolloids. 115:106595. doi: 10.1016/j.foodhyd.2021.106595.
- Kumar, Manoj, Maharishi Tomar, Sneh Punia, Jyoti Dhakane-Lad, Sangram Dhumal, Sushil Changan, Marisennayya Senapathy, Mukesh K. Berwal, Vellaikumar Sampathrajan, Ali A. S. Sayed, Deepak Chandran, R. Pandiselvam, Nadeem Rais, Dipendra Kumar Mahato, Shashikant Shiddappa Udikeri, Varsha Satankar, T. Anitha, Reetu, Radha, Surinder Singh, Ryszard Amarowicz, and John F. Kennedy. 2022a. “Plant-based proteins and their multifaceted industrial applications.” LWT - Food Science and Technology 154:112620. doi: 10.1016/j.lwt.2021.112620.
- Kumar, S. R., M. B. Sadiq, and A. K. Anal. 2022b. Comparative study of physicochemical and functional properties of soaked, germinated and pressure cooked Faba bean. Journal of Food Science and Technology 59 (1):257–67. doi: 10.1007/s13197-021-05010-x.
- Langyan, S., R. Bhardwaj, J. Kumari, S. R. Jacob, I. Singh Bisht, S. R. Pandravada, A. Singh, P. B. Singh, Z. A. Dar, A. Kumar, et al. 2022. Nutritional Diversity in Native Germplasm of Maize Collected From Three Different Fragile Ecosystems of India. Frontiers in Nutrition 9:812599. doi: 10.3389/fnut.2022.812599.
- Lath, A., A. R. Santal, N. Kaur, P. Kumari, and N. P. Singh. 2023. Anti-cancer peptides: Their current trends in the development of peptide-based therapy and anti-tumor drugs. Biotechnology & Genetic Engineering Reviews 39 (1):45–84. doi: 10.1080/02648725.2022.2082157.
- Lee, J. H., C. E. Hwang, E. J. Cho, Y. H. Song, S. C. Kim, and K. M. Cho. 2018. Improvement of nutritional components and in vitro antioxidative properties of soy-powder yogurts using Lactobacillus plantarum. Journal of Food and Drug Analysis 26 (3):1054–65. doi: 10.1016/j.jfda.2017.12.003.
- Li, C., J. Yang, L. Yao, F. Qin, G. Hou, B. Chen, L. Jin, J. Deng, and Y. Shen. 2020a. Characterisation, physicochemical and functional properties of protein isolates from Amygdalus pedunculata Pall seeds. Food Chemistry 311:125888. doi: 10.1016/j.foodchem.2019.125888.
- Li, C., L. Chen, D. J. McClements, X. Peng, Z. Xu, M. Meng, H. Ji, C. Qiu, J. Long, and Z. Jin. 2023. Encapsulation of polyphenols in protein-based nanoparticles: Preparation, properties, and applications. Critical Reviews in Food Science and Nutrition 1–15. doi: 10.1080/10408398.2023.2237126.
- Li, T., L. Wang, Z. Chen, D. Sun, and Y. Li. 2019. Electron beam irradiation induced aggregation behaviour, structural and functional properties changes of rice proteins and hydrolysates. Food Hydrocolloids. 97:105192. doi: 10.1016/j.foodhyd.2019.105192.
- Li, Z., S. Chu, P. Wang, S. Gao, S. Li, and X. Yu. 2020b. Effects of irradiation treatment on protein structure and digestion characteristics of seed-watermelon (Citrullus lanatus var.) kernel protein. Food Science and Biotechnology 29 (9):1201–11. doi: 10.1007/s10068-020-00777-9.
- Li, Z., Y. Fan, and J. Xi. 2019a. Recent advances in high voltage electric discharge extraction of bioactive ingredients from plant materials. Food Chemistry 277:246–60. doi: 10.1016/j.foodchem.2018.10.119.
- Liang, H.-N., and C.-H. Tang. 2013. pH-dependent emulsifying properties of pea [Pisum sativum (L.)] proteins. Food Hydrocolloids. 33 (2):309–19. doi: 10.1016/j.foodhyd.2013.04.005.
- Lin, M., S. Hong Tay, H. Yang, B. Yang, and H. Li. 2017. Development of eggless cakes suitable for lacto-vegetarians using isolated pea proteins. Food Hydrocolloids. 69:440–9. doi: 10.1016/j.foodhyd.2017.03.014.
- Liu, C., X. Wang, H. Ma, Z. Zhang, W. Gao, and L. Xiao. 2008. Functional properties of protein isolates from soybeans stored under various conditions. Food Chemistry 111 (1):29–37. doi: 10.1016/j.foodchem.2008.03.040.
- Liu, Q., J. Cheng, X. Sun, and M. Guo. 2021. Preparation, characterization, and antioxidant activity of zein nanoparticles stabilized by whey protein nanofibrils. International Journal of Biological Macromolecules 167:862–70. doi: 10.1016/j.ijbiomac.2020.11.043.
- Liu, Y., X.-Y. Ma, L.-N. Liu, Y.-P. Xie, Y.-J. Ke, Z.-J. Cai, and G.-J. Wu. 2019. Ultrasonic-assisted extraction and functional properties of wampee seed protein. Food Science and Technology 39 (suppl 1):324–31. doi: 10.1590/fst.03918.
- Liu, Y., K. Huang, Y. Zhang, H. Cao, D-k Luo, C. Yi, and X. Guan. 2023. Manufacture and characterization of a novel dairy-free quinoa yogurt fermented by modified commercial starter with Weissella confusa. Food Chemistry: X 19:100823. doi: 10.1016/j.fochx.2023.100823.
- Loushigam, G., and A. Shanmugam. 2023. Modifications to functional and biological properties of proteins of cowpea pulse crop by ultrasound-assisted extraction. Ultrasonics Sonochemistry 97:106448. doi: 10.1016/j.ultsonch.2023.106448.
- Lynch, H. M., M. P. Buman, J. M. Dickinson, L. B. Ransdell, C. S. Johnston, and C. M. Wharton. 2020. No Significant Differences in Muscle Growth and Strength Development When Consuming Soy and Whey Protein Supplements Matched for Leucine Following a 12 Week Resistance Training Program in Men and Women: A Randomized Trial. International Journal of Environmental Research and Public Health 17 (11):3871. doi: 10.3390/ijerph17113871.
- Ma, M., Y. Ren, W. Xie, D. Zhou, S. Tang, M. Kuang, Y. Wang, and S-k Du. 2018. Physicochemical and functional properties of protein isolate obtained from cottonseed meal. Food Chemistry 240:856–62. doi: 10.1016/j.foodchem.2017.08.030.
- Mala, T., M. B. Sadiq, and A. K. Anal. 2021. Comparative extraction of bromelain and bioactive peptides from pineapple byproducts by ultrasonic- and microwave-assisted extractions. Journal of Food Process Engineering 44 (6):e13709. doi: 10.1111/jfpe.13709.
- Malik, M. A., H. K. Sharma, and C. S. Saini. 2016. Effect of removal of phenolic compounds on structural and thermal properties of sunflower protein isolate. Journal of Food Science and Technology 53 (9):3455–64. doi: 10.1007/s13197-016-2320-y.
- Marc, R. A., V. Mureșan, A. E. Mureșan, C. C. Mureșan, A. E. Tanislav, A. Pușcaș, G. S. Marţiș, and R. A. Ungur. 2022. Spicy and Aromatic Plants for Meat and Meat Analogues Applications. Plants (Basel, Switzerland) 11 (7):960. doi: 10.3390/plants11070960.
- Mattice, K. D., and A. G. Marangoni. 2020. Physical properties of plant-based cheese products produced with zein. Food Hydrocolloids. 105:105746. doi: 10.1016/j.foodhyd.2020.105746.
- Mbithi-Mwikya, S., W. Ooghe, J. Van Camp, D. Ngundi, and A. Huyghebaert. 2000. Amino acid profiles after sprouting, autoclaving, and lactic acid fermentation of finger millet (Eleusine coracan) and kidney beans (Phaseolus vulgaris L.). Journal of Agricultural and Food Chemistry 48 (8):3081–5. doi: 10.1021/jf0002140.
- Messina, M. 2016. Soy and Health Update: Evaluation of the Clinical and Epidemiologic Literature. Nutrients 8 (12):754. doi: 10.3390/nu8120754.
- Mikhaylin, S. 2023. Chapter ten - Impact of high-voltage electrical treatments on the biofunctional properties of proteins and peptides. In Processing of Food Products and Wastes with High Voltage Electrical Discharges, edited by Eugene Vorobiev, Nadia Boussetta and Nikolai Lebovka, 247–61. Academic Press, London, United Kingdom. doi: 10.1016/B978-0-323-95403-7.00003-9.
- Mirón, I. J., C. Linares, and J. Díaz. 2023. The influence of climate change on food production and food safety. Environmental Research 216 (Pt 3):114674. doi: 10.1016/j.envres.2022.114674.
- Molina, E., A. Papadopoulou, and D. A. Ledward. 2001. Emulsifying properties of high pressure treated soy protein isolate and 7S and 11S globulins. Food Hydrocolloids. 15 (3):263–9. doi: 10.1016/S0268-005X(01)00023-6.
- Navarro-Lisboa, R., C. Herrera, R. N. Zúñiga, J. Enrione, F. Guzmán, S. Matiacevich, and C. Astudillo-Castro. 2017. Quinoa proteins (Chenopodium quinoa Willd.) fractionated by ultrafiltration using ceramic membranes: The role of pH on physicochemical and conformational properties. Food and Bioproducts Processing 102:20–30. doi: 10.1016/j.fbp.2016.11.005.
- Nazari, B., M. Amin Mohammadifar, S. Shojaee-Aliabadi, E. Feizollahi, and L. Mirmoghtadaie. 2018. Effect of ultrasound treatments on functional properties and structure of millet protein concentrate. Ultrasonics Sonochemistry 41:382–8. doi: 10.1016/j.ultsonch.2017.10.002.
- Ngui, S. P., C. E. Nyobe, C. B. Bakwo Bassogog, E. Nchuaji Tang, S. R. Minka, and M. A. Mune Mune. 2021. Influence of pH and temperature on the physicochemical and functional properties of Bambara bean protein isolate. Heliyon 7 (8):e07824. doi: 10.1016/j.heliyon.2021.e07824.
- Nitrayová, S., M. Brestenský, J. Heger, P. Patráš, J. Rafay, and A. Sirotkin. 2014. Amino acids and fatty acids profile of chia (Salvia hispanica L.) and flax (Linum usitatissimum L.) seed. Potravinarstvo Slovak Journal of Food Sciences 8 (1):72–6. doi: 10.5219/332.
- Norouzi, P., M. Mirmohammadi, and M. H. Houshdar Tehrani. 2022. Anticancer peptides mechanisms, simple and complex. Chemico-Biological Interactions 368:110194. doi: 10.1016/j.cbi.2022.110194.
- Obaroakpo, J. U., W. Nan, L. Hao, L. Liu, S. Zhang, J. Lu, X. Pang, and J. Lv. 2020. The hyperglycemic regulatory effect of sprouted quinoa yoghurt in high-fat-diet and streptozotocin-induced type 2 diabetic mice via glucose and lipid homeostasis. Food & Function 11 (9):8354–68. doi: 10.1039/D0FO01575J.
- Olabiran, T. E., O. O. Awolu, and H. N. Ayo-Omogie. 2023. Quality chracterization of functional soy-based yoghurt incorporated with scent leaf (Ocimum gratissimum) essential oil microcapsules. Food Chemistry Advances 3:100336. doi: 10.1016/j.focha.2023.100336.
- Oubannin, S., L. Bijla, J. Gagour, J. Hajir, N. A. Aabd, E. H. Sakar, M. A. Salama, and S. Gharby. 2022. A comparative evaluation of proximate composition, elemental profiling and oil physicochemical properties of black cumin (Nigella sativa L.) seeds and argan (Argania spinosa L. Skeels) kernels. Chemical Data Collections 41:100920. doi: 10.1016/j.cdc.2022.100920.
- Palanisamy, B. D., V. Rajendran, S. Sathyaseelan, R. Bhat, and B. P. Venkatesan. 2012. Enhancement of nutritional value of finger millet-based food (Indian dosa) by co-fermentation with horse gram flour. International Journal of Food Sciences and Nutrition 63 (1):5–15. doi: 10.3109/09637486.2011.591367.
- Park, J.-S., Y.-R. Jeong, and B.-S. Chun. 2019b. Physiological activities and bioactive compound from laver (Pyropia yezoensis) hydrolysates by using subcritical water hydrolysis. The Journal of Supercritical Fluids 148:130–6. doi: 10.1016/j.supflu.2019.03.004.
- Patnode, K., Z. Demchuk, S. Johnson, A. Voronov, and B. Rasulev. 2021. Computational Protein–Ligand Docking and Experimental Study of Bioplastic Films from Soybean Protein, Zein, and Natural Modifiers. ACS Sustainable Chemistry & Engineering 9 (32):10740–8. doi: 10.1021/acssuschemeng.1c01202.
- Peng, X., Y. Liao, K. Ren, Y. Liu, M. Wang, A. Yu, T. Tian, P. Liao, Z. Huang, H. Wang, et al. 2022. Fermentation performance, nutrient composition, and flavor volatiles in soy milk after mixed culture fermentation. Process Biochemistry 121:286–97. doi: 10.1016/j.procbio.2022.07.018.
- Peyrano, F., F. Speroni, and M. V. Avanza. 2016. Physicochemical and functional properties of cowpea protein isolates treated with temperature or high hydrostatic pressure. Innovative Food Science & Emerging Technologies 33:38–46. doi: 10.1016/j.ifset.2015.10.014.
- Pojić, M., A. Mišan, and B. Tiwari. 2018. Eco-innovative technologies for extraction of proteins for human consumption from renewable protein sources of plant origin. Trends in Food Science & Technology 75:93–104. doi: 10.1016/j.tifs.2018.03.010.
- Pomeranz, Y., G. S. Robbins, and L. W. Briggle. 1971. Amino acid composition of oat groats. Journal of Agricultural and Food Chemistry 19 (3):536–9. doi: 10.1021/jf60175a016.
- Prandi, B., M. Di Massimo, T. Tedeschi, L. Rodríguez-Turienzo, and Ó. Rodríguez. 2022. Ultrasound and Microwave-assisted Extraction of Proteins from Coffee Green Beans: Effects of Process Variables on the Protein Integrity. Food and Bioprocess Technology 15 (12):2712–22. doi: 10.1007/s11947-022-02907-z.
- Pu, C., W. Tang, M. Liu, Y. Zhu, and Q. Sun. 2020. Resveratrol-loaded hollow kafirin nanoparticles via gallic acid crosslinking: An evaluation compared with their solid and non-crosslinked counterparts. Food Research International (Ottawa, Ont.) 135:109308. doi: 10.1016/j.foodres.2020.109308.
- Qamar, S., Y. J. Manrique, H. Parekh, and J. R. Falconer. 2020. Nuts, cereals, seeds and legumes proteins derived emulsifiers as a source of plant protein beverages: A review. Critical Reviews in Food Science and Nutrition 60 (16):2742–62. doi: 10.1080/10408398.2019.1657062.
- Qiu, Y., D. J. McClements, J. Chen, C. Li, C. Liu, and T. Dai. 2023. Construction of 3D printed meat analogs from plant-based proteins: Improving the printing performance of soy protein- and gluten-based pastes facilitated by rice protein. Food Research International (Ottawa, Ont.) 167:112635. doi: 10.1016/j.foodres.2023.112635.
- Rahate, K. A., M. Madhumita, and P. K. Prabhakar. 2021. Nutritional composition, anti-nutritional factors, pretreatments-cum-processing impact and food formulation potential of faba bean (Vicia faba L.): A comprehensive review. Lwt 138:110796. doi: 10.1016/j.lwt.2020.110796.
- Rashwan, A. K., N. Karim, S. Liu, B. Paul, Y. Xu, and W. Chen. 2024. Physicochemical and antioxidant properties of set-type yogurt supplemented by lyophilized water-soluble Melastoma dodecandrum extract-bearded chitosan-coated nutriosomes. Food Hydrocolloids. 146:109311. doi: 10.1016/j.foodhyd.2023.109311.
- Rashwan, A. K., N. Karim, Y. Xu, H. Cui, J. Fang, K. Cheng, J. Mo, and W. Chen. 2022. Chemical composition, quality attributes and antioxidant activity of stirred-type yogurt enriched with Melastoma dodecandrum Lour fruit powder. Food & Function 13 (3):1579–92. doi: 10.1039/D1FO03448K.
- Rashwan, A. K., A. I. Osman, and W. Chen. 2023. Natural nutraceuticals for enhancing yogurt properties: A review. Environmental Chemistry Letters 21 (3):1907–31. doi: 10.1007/s10311-023-01588-0.
- Rashwan, A. K., A. I. Osman, N. Karim, J. Mo, and W. Chen. 2023. Unveiling the Mechanisms of the Development of Blueberries-Based Functional Foods: An Updated and Comprehensive Review. Food Reviews International 1–28. doi: 10.1080/87559129.2023.2245025.
- Rashwan, A. K., H. A. Yones, N. Karim, E. M. Taha, and W. Chen. 2021. Potential processing technologies for developing sorghum-based food products: An update and comprehensive review. Trends in Food Science & Technology 110:168–82. doi: 10.1016/j.tifs.2021.01.087.
- Rinaldoni, A. N., D. R. Palatnik, N. Zaritzky, and M. E. Campderrós. 2014. Soft cheese-like product development enriched with soy protein concentrates. LWT - Food Science and Technology 55 (1):139–47. doi: 10.1016/j.lwt.2013.09.003.
- Ruiz de la Bastida, A., S. Langa, Á. Peirotén, R. Fernández-Gonzalez, A. Sánchez-Jiménez, M. Maroto, J. Antonio Curiel, E. Guillamon, J. L. Arqués, A. Gutiérrez-Adán, et al. 2023. Effect of fermented soy beverage in aged female mice model. Food Research International (Ottawa, Ont.) 169:112745. doi: 10.1016/j.foodres.2023.112745.
- Sahni, P., S. Sharma, and V. K. R. Surasani. 2020. Influence of processing and pH on amino acid profile, morphology, electrophoretic pattern, bioactive potential and functional characteristics of alfalfa protein isolates. Food Chemistry 333:127503. doi: 10.1016/j.foodchem.2020.127503.
- Sampson, H. A., S. Aceves, S. Allan Bock, J. James, S. Jones, D. Lang, K. Nadeau, A. Nowak-Wegrzyn, J. Oppenheimer, T. Tamara, et al. 2014. Food allergy: A practice parameter update—2014. The Journal of Allergy and Clinical Immunology 134 (5):1016–25.e43. doi: 10.1016/j.jaci.2014.05.013.
- Sánchez-Velázquez, O. A., M. L. Manzanilla-Valdez, Y. Wang, M. Mondor, and A. J. Hernández-Álvarez. 2023. Micellar Precipitation and Reverse Micelle Extraction of Plant Proteins. In Green Protein Processing Technologies from Plants: Novel Extraction and Purification Methods for Product Development, edited by Alan Javier Hernández-Álvarez, Martin Mondor and Matthew G. Nosworthy, 237–63. Cham: Springer International Publishing. doi: 10.1007/978-3-031-16968-7_10.
- Sathe, S. K., W. J. Wolf, K. H. Roux, S. S. Teuber, M. Venkatachalam, and K. W. C. Sze-Tao. 2002. Biochemical Characterization of Amandin, the Major Storage Protein in Almond (Prunus dulcis L.). Journal of Agricultural and Food Chemistry 50 (15):4333–41. doi: 10.1021/jf020007v.
- Schmitt, C., and S. L. Turgeon. 2011. Protein/polysaccharide complexes and coacervates in food systems. Advances in Colloid and Interface Science 167 (1-2):63–70. doi: 10.1016/j.cis.2010.10.001.
- Sengupta, S., R. Goswami, S. Basu, and J. Bhowal. 2016. Hypolipidemic effects of soy yogurt fortified with antioxidant rich vegetable oil on albino mice fed high cholesterol diet. Materials Today: Proceedings 3 (10):3222–37. doi: 10.1016/j.matpr.2016.10.004.
- Sha, L., and Y. L. Xiong. 2020. Plant protein-based alternatives of reconstructed meat: Science, technology, and challenges. Trends in Food Science & Technology 102:51–61. doi: 10.1016/j.tifs.2020.05.022.
- Shaghaghian, S., D. J. McClements, M. Khalesi, M. Garcia-Vaquero, and A. Mirzapour-Kouhdasht. 2022. Digestibility and bioavailability of plant-based proteins intended for use in meat analogues: A review. Trends in Food Science & Technology 129:646–56. doi: 10.1016/j.tifs.2022.11.016.
- Sharma, P., J. Kaur, G. Sharma, and P. Kashyap. 2022. Plant derived antimicrobial peptides: Mechanism of target, isolation techniques, sources and pharmaceutical applications. Journal of Food Biochemistry 46 (10):e14348. doi: 10.1111/jfbc.14348.
- Shchekoldina, T., and M. Aider. 2014. Production of low chlorogenic and caffeic acid containing sunflower meal protein isolate and its use in functional wheat bread making. Journal of Food Science and Technology 51 (10):2331–43. doi: 10.1007/s13197-012-0780-2.
- Shevkani, K., N. Singh, A. Kaur, and J. C. Rana. 2015. Structural and functional characterization of kidney bean and field pea protein isolates: A comparative study. Food Hydrocolloids. 43:679–89. doi: 10.1016/j.foodhyd.2014.07.024.
- Shevkani, K., N. Singh, C. Patil, A. Awasthi, and M. Paul. 2022. Antioxidative and antimicrobial properties of pulse proteins and their applications in gluten-free foods and sports nutrition. International Journal of Food Science & Technology 57 (9):5571–84. doi: 10.1111/ijfs.15666.
- Shevkani, K., N. Singh, J. Chand Rana, and A. Kaur. 2014. Relationship between physicochemical and functional properties of amaranth (Amaranthus hypochondriacus) protein isolates. International Journal of Food Science & Technology 49 (2):541–50. doi: 10.1111/ijfs.12335.
- Shi, H., J. Li, E. Xu, H. Yang, D. Liu, and J. Yin. 2023b. Microscale 3D printing of fish analogues using soy protein food ink. Journal of Food Engineering 347:111436. doi: 10.1016/j.jfoodeng.2023.111436.
- Shi, L., F. Tianqi, C. Zhang, X. Deng, Y. Zhou, J. Wang, and L. Wang. 2023a. High-protein compound yogurt with quinoa improved clinical features and metabolism of high-fat diet–induced nonalcoholic fatty liver disease in mice. Journal of Dairy Science 106 (8):5309–27. doi: 10.3168/jds.2022-23045.
- Sitthiya, K., L. Devkota, M. B. Sadiq, and A. K. Anal. 2018. Extraction and characterization of proteins from banana (Musa Sapientum L) flower and evaluation of antimicrobial activities. Journal of Food Science and Technology 55 (2):658–66. doi: 10.1007/s13197-017-2975-z.
- Skovbjerg, C. K., J. N. Knudsen, W. Füchtbauer, J. Stougaard, F. L. Stoddard, L. Janss, and S. U. Andersen. 2020. Evaluation of yield, yield stability, and yield–protein relationship in 17 commercial faba bean cultivars. Legume Science 2 (3):e39. doi: 10.1002/leg3.39.
- Soleymani, F., E. Paquet, H. Viktor, W. Michalowski, and D. Spinello. 2022. Protein–protein interaction prediction with deep learning: A comprehensive review. Computational and Structural Biotechnology Journal 20:5316–41. doi: 10.1016/j.csbj.2022.08.070.
- Sorour, M. A., A. E. Mehanni, E. M. Taha, and A. K. Rashwan. 2017. Changes of total phenolics, tannins, phytate and antioxidant activity of two sorghum cultivars as affected by processing. Journal of Food and Dairy Sciences 8 (7):267–74. doi: 10.21608/jfds.2017.38699.
- Sujak, A., A. Kotlarz, and W. Strobel. 2006. Compositional and nutritional evaluation of several lupin seeds. Food Chemistry 98 (4):711–9. doi: 10.1016/j.foodchem.2005.06.036.
- Sun, X., and N. Bandara. 2019. Applications of reverse micelles technique in food science: A comprehensive review. Trends in Food Science & Technology 91:106–15. doi: 10.1016/j.tifs.2019.07.001.
- Sutter, R., M. Assad-Bustillos, and E. Windhab. 2023. Zein improves desirable melt-stretch properties in plant-based cheeses made from pea protein. Food Hydrocolloids. 144:108981. doi: 10.1016/j.foodhyd.2023.108981.
- Taha, A., F. Casanova, P. Šimonis, V. Stankevič, M. A. E. Gomaa, and A. Stirkė. 2022. Pulsed Electric Field: Fundamentals and Effects on the Structural and Techno-Functional Properties of Dairy and Plant Proteins. Foods (Basel, Switzerland) 11 (11):1556. doi: 10.3390/foods11111556.
- Tan, M., M. A. Nawaz, and R. Buckow. 2023. Functional and food application of plant proteins – a review. Food Reviews International 39 (5):2428–56. doi: 10.1080/87559129.2021.1955918.
- Tang, C.-H., and X. Sun. 2010. Physicochemical and structural properties of 8S and/or 11S globulins from mungbean [Vigna radiata (L.) Wilczek] with various polypeptide constituents. Journal of Agricultural and Food Chemistry 58 (10):6395–402. doi: 10.1021/jf904254f.
- Tang, C.-H., and X. Sun. 2011. A comparative study of physicochemical and conformational properties in three vicilins from Phaseolus legumes: Implications for the structure–function relationship. Food Hydrocolloids. 25 (3):315–24. doi: 10.1016/j.foodhyd.2010.06.009.
- Taylor, J. R. N., and J. Taylor. 2023. Do kafirin bioplastic materials have unique functional characteristics? Cereal Chemistry 100 (3):539–55. doi: 10.1002/cche.10648.
- Tsubaki, S., M. Nakauchi, Y. Ozaki, and J-i Azuma. 2009. Microwave heating for solubilization of polysaccharide and polyphenol from soybean residue (Okara). Food Science and Technology Research 15 (3):307–14. doi: 10.3136/fstr.15.307.
- Varghese, T., and A. Pare. 2019. Effect of microwave assisted extraction on yield and protein characteristics of soymilk. Journal of Food Engineering 262:92–9. doi: 10.1016/j.jfoodeng.2019.05.020.
- Veerichetty, V. 2018. Antidiabetic potential of the combination of fermented soy milk and flaxseed milk in alloxan-induced diabetic rats. International Journal of Green Pharmacy (IJGP) 12:S763–S768. doi: 10.22377/ijgp.v1.
- Venkateswara Rao, M., S. C K, A. Rawson, C. D V, and V. N. 2023. Modifying the plant proteins techno-functionalities by novel physical processing technologies: A review. Critical Reviews in Food Science and Nutrition 63 (19):4070–91. doi: 10.1080/10408398.2021.1997907.
- Vilcacundo, R., B. Miralles, W. Carrillo, and B. Hernández-Ledesma. 2018. In vitro chemopreventive properties of peptides released from quinoa (Chenopodium quinoa Willd.) protein under simulated gastrointestinal digestion. Food Research International (Ottawa, Ont.) 105:403–11. doi: 10.1016/j.foodres.2017.11.036.
- Wahlström, R., K. Rommi, P. Willberg-Keyriläinen, D. Ercili-Cura, U. Holopainen-Mantila, J. Hiltunen, O. Mäkinen, H. Nygren, A. Mikkelson, and L. Kuutti. 2017. High yield protein extraction from Brewer’s spent grain with novel carboxylate salt - urea aqueous deep eutectic solvents. ChemistrySelect 2 (29):9355–63. doi: 10.1002/slct.201701492.
- Wang, L., Y. Ding, X. Zhang, Y. Li, R. Wang, X. Luo, Y. Li, J. Li, and Z. Chen. 2017a. Effect of electron beam on the functional properties and structure of defatted wheat germ proteins. Journal of Food Engineering 202:9–17. doi: 10.1016/j.jfoodeng.2017.01.024.
- Wang, L., X. Zhang, F. Liu, Y. Ding, R. Wang, X. Luo, Y. Li, and Z. Chen. 2017b. Study of the functional properties and anti-oxidant activity of pea protein irradiated by electron beam. Innovative Food Science & Emerging Technologies 41:124–9. doi: 10.1016/j.ifset.2017.01.005.
- Wang, N., and J. K. Daun. 2006. Effects of variety and crude protein content on nutrients and anti-nutrients in lentils (Lens culinaris. Food Chemistry 95 (3):493–502. doi: 10.1016/j.foodchem.2005.02.001.
- Wang, S., Y. Lu, X-k Ouyang, and J. Ling. 2020. Fabrication of soy protein isolate/cellulose nanocrystal composite nanoparticles for curcumin delivery. International Journal of Biological Macromolecules 165 (Pt A):1468–74. doi: 10.1016/j.ijbiomac.2020.10.046.
- Wang, T., L. Kaur, A. Singh Beniwal, Y. Furuhata, H. Aoyama, and J. Singh. 2023d. Physico-chemical and Textural Properties of 3D Printed Plant-based and Hybrid Soft Meat Analogs. Plant Foods for Human Nutrition (Dordrecht, Netherlands) 78 (2):375–82. doi: 10.1007/s11130-023-01068-4.
- Wang, X.-S., C.-H. Tang, X.-Q. Yang, and W.-R. Gao. 2008. Characterization, amino acid composition and in vitro digestibility of hemp (Cannabis sativa L.) proteins. Food Chemistry 107 (1):11–8. doi: 10.1016/j.foodchem.2007.06.064.
- Wang, X., X. Kong, C. Zhang, Y. Hua, Y. Chen, and X. Li. 2023c. Comparison of physicochemical properties and volatile flavor compounds of plant-based yoghurt and dairy yoghurt. Food Research International (Ottawa, Ont.) 164:112375. doi: 10.1016/j.foodres.2022.112375.
- Wang, Y., B. Lyu, H. Fu, J. Li, L. Ji, H. Gong, R. Zhang, J. Liu, and H. Yu. 2023b. The development process of plant-based meat alternatives: Raw material formulations and processing strategies. Food Research International (Ottawa, Ont.) 167:112689. doi: 10.1016/j.foodres.2023.112689.
- Wang, Y., W. Cai, L. Li, Y. Gao, and K-h Lai. 2023a. Recent advances in the processing and manufacturing of plant-based meat. Journal of Agricultural and Food Chemistry 71 (3):1276–90. doi: 10.1021/acs.jafc.2c07247.
- Warnakulasuriya, S. N., and M. T. Nickerson. 2018. Review on plant protein–polysaccharide complex coacervation, and the functionality and applicability of formed complexes. Journal of the Science of Food and Agriculture 98 (15):5559–71. doi: 10.1002/jsfa.9228.
- Webb, D., Y. Li, and S. Alavi. 2023. Chemical and physicochemical features of common plant proteins and their extrudates for use in plant-based meat. Trends in Food Science & Technology 131:129–38. doi: 10.1016/j.tifs.2022.11.006.
- Wei, L., W. Zhang, J. Yang, Y. Pan, H. Chen, and Z. Zhang. 2023. The application of deep eutectic solvents systems based on choline chloride in the preparation of biodegradable food packaging films. Trends in Food Science & Technology 139:104124. doi: 10.1016/j.tifs.2023.104124.
- Wei, Y., Z. Cai, M. Wu, Y. Guo, P. Wang, R. Li, A. Ma, and H. Zhang. 2020. Core-shell pea protein-carboxymethylated corn fiber gum composite nanoparticles as delivery vehicles for curcumin. Carbohydrate Polymers 240:116273. doi: 10.1016/j.carbpol.2020.116273.
- Yamada, M., S. Morimitsu, E. Hosono, and T. Yamada. 2020. Preparation of bioplastic using soy protein. International Journal of Biological Macromolecules 149:1077–83. doi: 10.1016/j.ijbiomac.2020.02.025.
- Yan, X., S. Liang, T. Peng, G. Zhang, Z. Zeng, P. Yu, D. Gong, and S. Deng. 2020. Influence of phenolic compounds on physicochemical and functional properties of protein isolate from Cinnamomum camphora seed kernel. Food Hydrocolloids. 102:105612. doi: 10.1016/j.foodhyd.2019.105612.
- Yang, M., L. Chen, J. Wang, G. Msigwa, A. I. Osman, S. Fawzy, D. W. Rooney, and P.-S. Yap. 2023. Circular economy strategies for combating climate change and other environmental issues. Environmental Chemistry Letters 21 (1):55–80. doi: 10.1007/s10311-022-01499-6.
- Yao, G., Y. Guo, T. Cheng, Z. Wang, B. Li, C. Xia, J. Jiang, Y. Zhang, Z. Guo, and H. Zhao. 2022. Effect of γ-irradiation on the physicochemical and functional properties of rice protein. Food Science and Technology 42:e12422. doi: 10.1590/fst.12422.
- Yi, J., Q. He, G. Peng, and Y. Fan. 2022. Improved water solubility, chemical stability, antioxidant and anticancer activity of resveratrol via nanoencapsulation with pea protein nanofibrils. Food Chemistry 377:131942. doi: 10.1016/j.foodchem.2021.131942.
- Yildiz, G., J. Ding, J. Andrade, N. J. Engeseth, and H. Feng. 2018. Effect of plant protein-polysaccharide complexes produced by mano-thermo-sonication and pH-shifting on the structure and stability of oil-in-water emulsions. Innovative Food Science & Emerging Technologies 47:317–25. doi: 10.1016/j.ifset.2018.03.005.
- Yong Sik, C., and S. K. Bin. 2000. Effect of γ-Irradiation on the Molecular Properties of Bovine Serum Albumin and β-Lcatoglobulin. BMB Reports 33:133–7. https://kiss.kstudy.com/Detail/Ar?key=427566.
- Yu, X., O. Bals, N. Grimi, and E. Vorobiev. 2015. A new way for the oil plant biomass valorization: Polyphenols and proteins extraction from rapeseed stems and leaves assisted by pulsed electric fields. Industrial Crops and Products 74:309–18. doi: 10.1016/j.indcrop.2015.03.045.
- Yuan, De-Bao, Xiao-Quan Yang, Chuan-He Tang, Zhi-Xiong Zheng, Min Wei, Ijaz Ahmad, and Shou-Wei Yin. 2009. “Physicochemical and functional properties of acidic and basic polypeptides of soy glycinin.” Food Research International, 42(5–6):700–706. doi: 10.1016/j.foodres.2009.02.005.
- Yuan, X., W. Jiang, D. Zhang, H. Liu, and B. Sun. 2021. Textural, Sensory and Volatile Compounds Analyses in Formulations of Sausages Analogue Elaborated with Edible Mushrooms and Soy Protein Isolate as Meat Substitute. Foods (Basel, Switzerland) 11 (1):52. doi: 10.3390/foods11010052.
- Zhang, Z., Y. Hu, H. Ji, Q. Lin, X. Li, S. Sang, D. Julian McClements, J. Long Chen, A. Long, X. Jiao, et al. 2023. Physicochemical stability, antioxidant activity, and antimicrobial activity of quercetin-loaded zein nanoparticles coated with dextrin-modified anionic polysaccharides. Food Chemistry 415:135736. doi: 10.1016/j.foodchem.2023.135736.
- Zhao, S., X. Yuan, L. Yang, M. Zhu, H. Ma, and Y. Zhao. 2023a. The effects of modified quinoa protein emulsion as fat substitutes in frankfurters. Meat Science 202:109215. doi: 10.1016/j.meatsci.2023.109215.
- Zhao, Y., R. Tian, Z. Xu, L. Jiang, and X. Sui. 2023b. Recent advances in soy protein extraction technology. Journal of the American Oil Chemists’ Society 100 (3):187–95. doi: 10.1002/aocs.12676.
- Zhou, J-z., H. Zhang, L. Gao, L. Wang, and H.-F. Qian. 2015. Influence of pH and ionic strength on heat-induced formation and rheological properties of cottonseed protein gels. Food and Bioproducts Processing 96:27–34. doi: 10.1016/j.fbp.2015.06.004.
- Zhou, M., J. Liu, Y. Zhou, X. Huang, F. Liu, S. Pan, and H. Hu. 2016. Effect of high intensity ultrasound on physicochemical and functional properties of soybean glycinin at different ionic strengths. Innovative Food Science & Emerging Technologies 34:205–13. doi: 10.1016/j.ifset.2016.02.007.
- Zhou, X., J. Zhao, X. Zhao, R. Sun, C. Sun, D. Hou, X. Zhang, L. Jiang, J. Hou, and Z. Jiang. 2022a. Oil bodies extracted from high-oil soybeans (Glycine max) exhibited higher oxidative and physical stability than oil bodies from high-protein soybeans. Food & Function 13 (6):3271–82. doi: 10.1039/D1FO03934B.
- Zhou, Y., W. Wu, N. Zhang, O. P. Soladoye, Y. Zhang, and Y. Fu. 2022b. Deep eutectic solvents as new media for green extraction of food proteins: Opportunity and challenges. Food Research International (Ottawa, Ont.) 161:111842. doi: 10.1016/j.foodres.2022.111842.
- Zhu, G., X. Zhu, Q. Fan, X. Liu, Y. Shen, and J. Jiang. 2010. Study on Production of Amino Acids from Bean Dregs by Hydrolysis in Sub-critical Water. Chinese Journal of Chemistry 28 (10):2033–8. doi: 10.1002/cjoc.201090339.
- Zou, Y., L. Wang, P. Li, P. Cai, M. Zhang, Z. Sun, C. Sun, Z. Geng, W. Xu, X. Xu, et al. 2017. Effects of ultrasound assisted extraction on the physiochemical, structural and functional characteristics of duck liver protein isolate. Process Biochemistry 52:174–82. doi: 10.1016/j.procbio.2016.09.027.