Abstract
With the development of nanotechnology, self-assembled chitosan/phospholipid nanoparticles (SACPNs) show great promise in a broad range of applications, including therapy, diagnosis, in suit imaging and on-demand drug delivery. Here, a brief review of the SACPNs is presented, and its critical underlying formation mechanisms are interpreted with an emphasis on the intrinsic physicochemical properties. The state-of-art preparation methods of SACPNs are summarized, with particular descriptions about the classic solvent injection method. Then SACPNs microstructures are characterized, revealing the unique spherical core-shell structure and the drug release mechanisms. Afterwards, a comprehensive and in-depth depiction of their emerging applications, with special attention to drug delivery areas, are categorized and reviewed. Finally, conclusions and outlooks on further advancing the SACPNs toward a more powerful and versatile platform for investigations covering from fundamental understanding to developing multi-functional drug delivery systems are discussed.
1. Introduction
1.1. Background
Nanosystems with controllable structures and advanced functions, such as liposomes, micelles, nanoemulsions and nanoparticles, show great power in a wide range of applications, including diagnosis, therapy, in suit imaging and on-demand drug delivery (Barratt, Citation2000; Sonvico et al., Citation2005; Bahadori & Mohammadi, Citation2006; Ochekpe, et al., Citation2009). Among all the different types of nanosystems, nanoparticles, including silica nanoparticles, carbon nanoparticles, solid lipid nanoparticles, magnetic nanoparticles and polymeric nanoparticles (), have attracted broad scientific interests in recent years due to its distinct characteristics, such as the abilities to offer high stability and encapsulation capacity of drugs, possibility to deliver both hydrophilic and hydrophobic ingredients, and feasibility for providing multiple routes of administration capability (Sanguansri & Augustin, Citation2006; Kaur et al., Citation2008; Chuah et al., Citation2009; Joshy et al., Citation2018; Mathew et al., Citation2018). Several nanoparticle-based drug delivery systems have been introduced into clinical practice, in particular for cancer treatment (Torchilin, Citation2005; Sato et al., Citation2006). However, the vast majorities of these nanoparticles either needs complicated fabrication process (such as high pressure, temperature and long operation duration) or involve the utilization of harmful chemicals (such as DMSO and tetrahydrofuran) deemed to be nonbiocompatible, and are therefore not suitable for biomedical applications (Vauthier & Bouchemal, Citation2009; Battaglia & Gallarate, Citation2012). Therefore, proposing new methods to fabricate nanoparticles in a more succinct and biocompatible manner is highly appreciated.
Table 1. Summary of nanoparticles as drug delivery systems.
To overcome the above-mentioned problems, the recently appreciated ‘self-assembly approach’ is one of the most promising methods to fabricate nanoparticles without involving any organic solvents or cross-linking agents (Yoo et al., Citation2005; Cho et al., Citation2012). This method allows nanoparticles to be generated from individual molecules that are able to assemble spontaneously by electrostatic interactions or noncovalent interactions (Moraru et al., Citation2003; Schatz et al., Citation2004; Schug & Lindner, Citation2005). Besides saving energy, this approach is also more favorable than conventional approaches by protecting the encapsulated bioactive ingredients from harmful stresses involved in conventional fabrication process (Ichikawa et al., Citation2005).
1.2. Object
Various biocompatible and biodegradable natural polymers show the ability to self-assemble (Li et al., Citation2014). Among all the choices, chitosan and phospholipid are the well-accepted candidates due to their superior biocompatibility and biodegradability (S Duttagupta et al., Citation2015; Zhou et al., Citation2017). Specifically, chitosan is one positively charged natural alkaline polysaccharides derived from chitin that shows nontoxic in both animals and humans with many unique biological characteristics, such as the remarkable bioadhesiveness to mucosal surfaces and the ability to open tight junctions in epithelial cells to strongly promote drug penetration and absorption (Van der Lubben et al., Citation2001; Panos et al., Citation2008; Abdolhi et al., Citation2017; Raza & Anwar, Citation2017; Eid et al., Citation2018; Ejeromedoghene et al., Citation2018; Manuja et al., Citation2018; Augustine et al., Citation2019; Dutta et al., Citation2019). Phospholipids are negatively charged lipid mixtures and act as the basic component of biofilms with various surface activities. They have been widely used in the preparation of various lipid-based drug delivery systems. For instance, Dr. Joshy K. S. has demonstrated the formation of various phospholipid-based nanoparticles for delivering anti-virus drugs and for tissue engineering (Grant et al., Citation2005; Joshy & Sharma, Citation2012; Joshy et al., Citation2017, Citation2018). Nanoparticles can be formed through the self-assembly between phospholipids and chitosan via electrostatic interactions. Recently, a great deal of interests has been attracted to this self-assembled chitosan/phospholipid nanoparticles (SACPNs), which is the main object that this review would like to introduce, as illustrated schematically in .
1.3. Significance
The preparation of SACPNs is quite simple and can be directly prepared by triggering the self-assembly between chitosan and phospholipid; the preparation condition is mild, avoiding the chemical cross-linking agents required for the conventional preparation techniques and the involved tedious operations such as continuous cleaning and continuously performed precipitation (Rodriguez-Hernandez et al., Citation2005). Moreover, SACPNs have a lipid core and a hydration shell; drugs can be encapsulated in the lipid core by physical embedding or electrostatic interactions with phospholipid. SACPNs can offer high the encapsulation efficiency and superior protection over the encapsulated active drugs. Due to the inherent advantages of phospholipids and chitosan, SACPNs can significantly prolong the residence time of drugs at the action site, promote the penetration and absorption of drugs, and therefore optimize pharmacokinetics (Sahoo et al., Citation2008). Ever since the SACPNs were first proposed and demonstrated in 2006, plenty of researches on SACPNs have been carried out and significant progress has been made (Sonvico et al., Citation2006). Therefore, a comprehensive and in-depth depiction of the whole scene on SACPNs, from fundamentals to preparations for various advanced applications, is desired.
1.4. Contents
The contents of this review include (Yoo et al., Citation2011; Zhang et al., Citation2012): (1) the elucidation of the fundamentals of the self-assembly process; (2) the preparation of SACPNs with designed structures; (3) the elaborately characterization of microstructures for SACPNs; and (4) SACPNs with integrated functions for emerging various applications. We present an overview of SACPNs covering from basic principles to progresses made in preparation for advanced applications in recent years.
2. Preparation of SACPNs
SACPNs can be prepared by multiple techniques, such as spray drying, ion coagulation, emulsion crosslinking and solvent injection (Naskar et al., Citation2019). Among all the methods, the classic solvent injection method is the most convenient and widely applied one, as illustrated schematically in (Sonvico et al., Citation2006). In detail, the drug is first dissolved in an ethanol solution containing phospholipids, and then the ethanol phase is directly injected into the chitosan aqueous solution under certain stirring speed, subsequently leads to the successful SACPNs formation without involving any intermediate. The results show that the particle size of the generated SACPNs decreases significantly when decreasing the viscosity of chitosan. Moreover, the mass ratio of phospholipids to chitosan has a significant impact over the particle size, zeta potential and stability of the generated SACPNs (Ghosal et al., Citation2018). When the mass ratio of phospholipids to chitosan is in the range of 5:1–20:1, SACPNs with a small particle size (below 280 nm) and a narrow size distribution can be generated. The formed SACPNs hold a strong positive potential (+40 mV) and good stability. Besides, when conducting the self-assembly in the pH range of 2.5–5.0, the increase of pH leads to the decrease of the positive potential of the SACPNs surface, while the particle size are increased slightly. When the pH is above 5.0, the positive potential of SACPNs decreases rapidly and the particle size increases drastically, which may due to the decrease in the positive charge density of chitosan at high pH values. Therefore, the mass ratio of phospholipid to chitosan, viscosity of chitosan and pH values have significant effects on the particle size and surface charge of the generated SACPNs by conventional solvent injection methods (Sonvico et al., Citation2005; Citation2006; Gerelli et al., Citation2008).
Figure 2. Schematic illustration of the preparation of SACPNs by the classic solvent injection method.
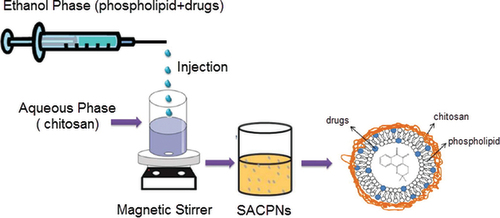
In order to further improve the performance of the generated SACPNs, several modified methods have been proposed and demonstrated. For instance, by adding isopropyl myristate into the ethanol phase, the encapsulation efficiency of the clobetasol propionate can be increased from less than 60% to above 90%. The reason may be attributed to the fact that the addition of isopropyl myristate facilitates the distribution of the clobetasol propionate in the lipid core and thus improve its encapsulation efficiency (Şenyiğit et al., Citation2010). Besides, tocopheryl propylene glycol succinct is also chosen as the surfactant. The resultant SACPNs show a mean particle size of 95.3 nm, and the entrapment efficiency and drug loading for quercetin are 48.5% and 2.45%, respectively, as shown in . Compared with pure quercetin solution, the quercetin-loaded SACPNs showed higher permeation ability, and significantly increased accumulation of quercetin in the skin (Tan et al., Citation2011). Modified solvent injection method has also been applied to prepare novel insulin-loaded SACPNs. The cryo-SEM results show that the generated SACPNs exhibit a multi-layered cystic structure with an average particle size of 180 nm, an insulin encapsulation efficiency of 94% with a drug loading capacity of 4.5%, which can greatly improve the stability of insulin in artificial gastric and intestinal fluids (Liu et al., Citation2016).
Figure 3. (a) Schematic illustration of quercetin-loaded SACPNs. (b) TEM images of quercetin-loaded SACPNs. (c) Visual observation of crude quercetin in water (left) and quercetin-loaded SACPNs suspension (right). (a, b, c) Reproduced with permission (Tan et al., Citation2011). Copyright 2011, Informa PLC.
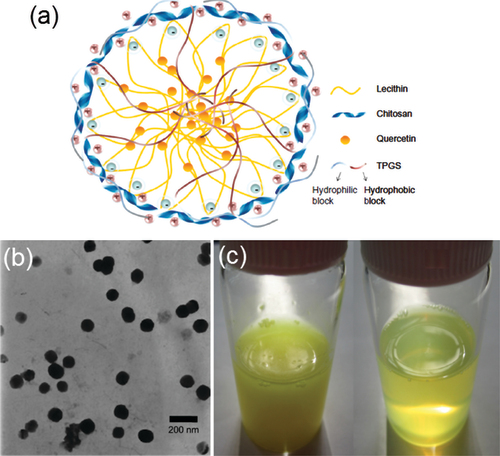
3. Formation mechanism of SACPNs
The formation mechanism of SACPNs has been investigated in depth and made great progress recently (Park et al., Citation2004; Quiñones et al., Citation2018). The Langmuir monolayer technique has been used to characterize the interactions between chitosan and phospholipid at interfaces. Specifically, monolayers of phospholipid were formed on the subphase containing different concentrations of chitosan, and their isotherms were measured. The results showed that chitosan significantly modified the monolayers. Their expansion provided evidence for chitosan binding to the phospholipid. The expansion was found to reach the saturation with increasing chitosan concentration. The degree of saturation of the acid hydrocarbon chain had a significant impact on the architecture developed, namely, the higher was the number of the double bonds, the higher the monolayer expansion at saturation took place. As a conclusion, electrostatic interactions, hydrogen bonding, and hydrophobic interactions were considered as possibly participating in the overall mechanism of chitosan-lipid interactions at interfaces (Wydro et al., Citation2007). Typically, the electrostatic interactions between negatively charged phospholipids and positively charged chitosan contribute primarily to the formation of SACPNs. For instance, for the prepared SACPNs with an average diameter of 150 nm (), Fourier transform infrared spectroscopy (FTIR) results show that the shear vibration absorption peak for -NH2 at 1590 cm−1 of chitosan disappears, while the main absorption peak for amide at 1660 cm−1 shows no significant change; moreover, the absorption peak for phosphate group in phospholipid shifts from 1236 cm−1 to 1217 cm−1, while the absorption peak for the fatty acid chain carbonyl at 1738 cm−1 shows no significant change with only a slight decrease in intensity, as shown by the FTIR plots in , demonstrating that the positively charged functional group in chitosan interacts with the polar portion of the phospholipid to induce the self-assembly formation (Sonvico et al., Citation2006). Moreover, studies by neutron scattering have also confirmed the strong electrostatic interactions between phospholipid and chitosan. Specifically, SACPNs were prepared, freeze-dried and re-hydrated in a D2O atmosphere. Then the neutron scattering were performed in the temperature range of 20–50 K using the backscattering spectrometer. The comparison of SACPNs in the dry state with similar ones at an hydration level of about 0.3–0.4 (g D2O/g hydrated sample), as shown in Sonvico et al. Citation2006. The comparison results indicate that the presence of an outer chitosan ‘coating’ reduces the mean square fluctuations of the hydrogen bonding in the lipid component, thus leading to a stiffer nanostructure.
Figure 4. (a) Transmission electron micrographs of SACPNs. (b) Atomic force microscopy images of SACPNs. (c) FTIR spectra of SACPNs and of the two components separately. (d) Comparison of the mean square hydrogen fluctuation differences of a dry SACPNs (open triangles) and a pure phospholipid one (closed triangles). (e) FTIR spectra of (e1) chitosan, (e2) phospholipid, (e3) natamycin and (e4) natamycin-loaded SACPNs. (a, b, c) Reproduced with permission (Sonvico et al., Citation2006). Copyright 2006, Elsevier. (d) Reproduced with permission (Sonvico et al., Citation2006). Copyright 2006, Elsevier. (e) Reproduced with permission (Bhatta et al., Citation2012). Copyright 2012, Elsevier.
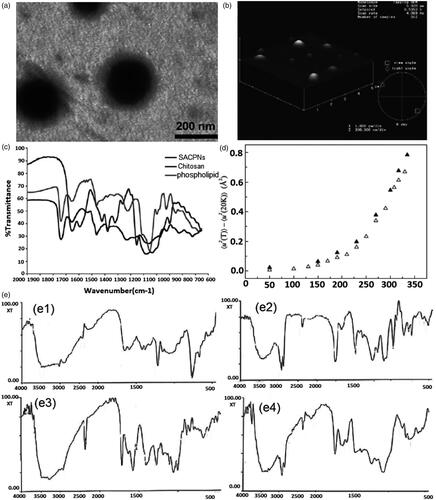
Besides, SACPNs encapsulated with drugs are also used as models for investigating the formation mechanism. For instance, for SACPNs loaded with natamycin, the FTIR results show that the shear vibration absorption peak for –NH2 at 1590 cm−1 of chitosan disappears, and the absorption peak of the phosphate group in the phospholipid shifts from 1245 cm−1 to 1216 cm−1, while there are no interactions between natamycin and the chitosan or phospholipid, as shown by the plots in Bhatta et al. (Citation2012). These results demonstrate that in an acidic medium, the amino groups of the chitosan can be protonated with positive charges and can interact with the phosphate group of the phospholipid through the non-covalent bond-charge interactions to form stable SACPNs spontaneously (Lawrie et al., Citation2007; Chuah et al., Citation2009). During the self-assembly process, drugs can be loaded into the SACPNs by means like dissolution, physical embedding and chemical bonding (Goldberg et al., Citation2007).
4. Characterization of SACPNs microstructures
The microstructure of SACPNs has a significant impact on its functional properties, such as encapsulation efficiency, release behavior in vitro and in vivo, and storage stability. Transmission electron microscopy (TEM), atomic force microscopy (AFM) and neutron scattering results show that the SACPNs have a spherical core-shell structure, as demonstrated in and illustrated schematically in . Particularly, the interior is a dense core mainly composed of phospholipid, providing an ideal place for the encapsulation and delivery of hydrophobic drugs, such as curcumin (Pathak et al., Citation2015), artesunate (Chadha et al., Citation2012), diflumethasone valerate (Özcan et al., Citation2013), and clobetasol propionate (Şenyiğit et al., Citation2010), and their encapsulation efficiency in SACPNs can be as high as 80%. The extranuclear is a positively charged hydration shell, which is beneficial to exerting the unique biological characteristics of chitosan. Moreover, mesoscopic simulation has been applied to study the self-assembly behavior and the structure of SACPNs. It is found that phospholipid and chitosan spontaneously form stable nanoparticles, and the drug can be encapsulated in the lipid core, while chitosan distributes around the exteriors of the SACPNs, as shown by the simulated schematics in Terrón-Mejía et al. (Citation2018).
Figure 5. (a) Schematic illustrations of the mesoscopic models of SACPNs. The yellow spheres represent the hydrophobic part of the phospholipid, while the red spheres represent the hydrophilic part. The chitosan are assembled in a random configuration around the phospholipid. (b) Distribution of chitosan on the xy plane. (b1) 50 chains of chitosan; (b2) 100 chains of chitosan; (b3) 150 chains of chitosan; (b4) 200 chains of chitosan. (c) Cryo-TEM image of SACPNs containing tamoxifen (c1) and progesterone (c2). (a, b) Reproduced with permission (Terrón-Mejía et al., Citation2018). Copyright 2018, MDPI. (c) Reproduced with permission (Gerelli et al., Citation2008). Copyright 2007, IOP Publishing.
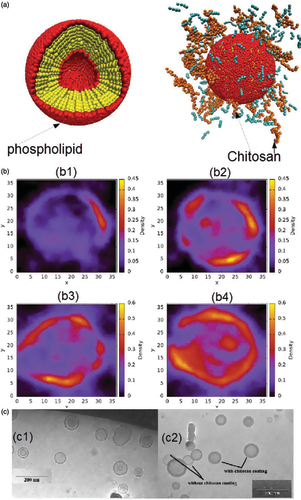
Moreover, the microstructures of SACPNs are also closely related with the hydrophobicity and charge properties of the encapsulated drugs. For instance, when loading the uncharged hydrophobic progesterone into SACPNs, the formed nanoparticles are with a single-chamber vesicle structure composed of a lipid bilayer and surrounded by an outer layer of chitosan, as shown in , which is similar to the structure of unloaded blank SACPNs. However, when loading the positively charged hydrophobic tamoxifen, the microstructure of the formed nanoparticles is diversified. Specifically, the microstructure is changed into a single-chamber vesicle with a particle size of 20 nm formed by a chitosan-coated phospholipid layer, as well as a multi-chamber vesicle with particle size around 80 nm, and the vesicles are composed of the lipid bilayer and chitosan which are alternately separated and multi-layered. Most of the tamoxifen (above sixty percent) is loaded into multi-compartment vesicles and is distributed in multiple layers of lipids, with only less than thirty percent into single-chamber vesicles (Gerelli et al., Citation2008).
5. Drug release mechanism of SACPNs
The drug delivery mechanism of SACPNs is critical for their applications described afterwards and deserved to be stated in detail (Narayanan et al., Citation2014). There are mainly three types of release mechanisms of SACPNs: release from the surface, erosion-based release and release from the swelled matrix, as illustrated schematically in (Nathanson et al., Citation2018). The release of drugs from SACPNs is a complicated process and typically comprises not only one mechanism (Jourghanian et al., Citation2016). For instance, when the drug-loaded SACPNs are subjected to physiological environment, the encapsulated drugs on the surface of SACPNs will be first released, leading to the ‘burst release’ phenomenon. Gradually, the chitosan shell of SACPNs would be eroded by the physiological environment, resulting in the release of drugs from the shell. Besides, different stimuli from the physiological environment, such as pH changes and ionic strength changes, would weaken the electrostatic interactions between chitosan and phospholipid, leading to the swelling of SACPNs and triggering the release of drugs encapsulated in the lipid cores of SACPNs (Son et al., Citation2017).
6. Emerging applications for SACPNs
SACPNs create an excellent eco-friendly environment for delivering active components, which can be harnessed in many applications including the drug delivery, production of cosmetics and foods (Chaouat et al., Citation2017), tissue engineering (Shanmugam & Banerjee, Citation2011), cell sorting and capture, biochemical assays (Zhang et al., Citation2008). Here, we limit our discussion to the progress made in recent emerging applications including drug delivery systems, advanced foods and antibacterial field.
6. 1. Advanced drug delivery systems
Ever since the SACPNs were proposed and fabricated, the applications of SACPNs in drug delivery systems have been extensively studied. Various active pharmaceutical ingredients (APIs) have been encapsulated with optimized encapsulation efficiency, drug loading, particle sizes and unique characteristics, as summarized in (Hafner et al., Citation2011; Barbieri et al., Citation2013; Chhonker et al., Citation2015; Moreno et al., Citation2015; Clementino, Batger, et al., 2016; Liu et al., Citation2016; Clementino, Pozzoli, et al. Citation2018; Alkholief, Citation2019; Khan, Citation2019; Terrón-Mejía et al., Citation2018). Due to the remarkable biocompatible and biodegradable of SACPNs, it can be used as ideal drug vehicles for transdermal, mucosal, ocular and oral drug delivery. It is worth mentioning that the biocompatibility and bioactivity of SACPNs are extremely important when used for drug delivery. The biocompatibility and bioactivity of SACPNs are typically investigated by MTT assay and hemolysis test in vitro. Moreover, intraperitoneally administration into mouse is used to investigate the acute toxicity in vivo and biodistribution test is also applied to identify whether it has good biocompatibility and bioactivity (Cardoso et al., Citation2018; Nosrati et al., Citation2018; Yuan et al., Citation2018). Among all these techniques, MTT assay is the primary one that is typically used as the first test to investigate the biocompatibility and bioactivity of SACPNs.
Table 2. Summary of recent studies of SACPNs as drug delivery systems.
6.1.1 Transdermal delivery
Nanocarriers have appeared as an effective strategy for transdermal drug delivery due to the overcome of steric obstruction and protection of active ingredients at both extracellular and intracellular environments (Liu et al., Citation2015). SACPNs can promote percutaneous penetration of drugs therefore can be used as desirable platform for mucosal drug delivery. The improvement of percutaneous penetration is closely related to the unique property of chitosan. Specifically, adhesive chitosan helps to prolong the residence time of the drug on the skin surface and facilitate the skin hydration and swelling. At the same time, chitosan has the ability to reversibly open the tight junctions between keratinocytes and weakening the barrier layer of the stratum corneum. Moreover, phospholipids can be well fused with the lipid components in the skin, both of which are beneficial for the drug to penetrate the skin from the surface (van der Lubben et al., Citation2001). For instance, clobetasol propionate-loaded SACPNs with a particle size around 250 nm are generated, as shown in . The anti-inflammatory activity is then evaluated using carrageenan-induced hind paw edema test on rats, and histological analysis is performed to evaluate the possible presence of morphological changes, as shown in . The results indicate that SACPNs-in-gel formulation show significantly higher edema inhibition compared to other formulations tested. Furthermore, histological analysis of rat abdominal skin shows neither morphological tissue changes nor cell infiltration signs after application of the formulations. Taken together, the results show that the use of SACPNs in chitosan gel as a drug carrier significantly improves the risk-benefit ratio as compared with sodium-deoxycholate gel and commercial cream formulations of clobetasol propionate (Şenyiğit et al., Citation2016). Besides, SACPNs for dermal delivery of diflucortolone valerate (DFV) that would maintain the localization in skin layers without any penetration have also been prepared. The results of rat skin permeation test show that the SACPNs-based gel can significantly increase the retention time of the drug on the skin, especially the stratum corneum and epidermis, and the retention amount is 6.33 times that of diflumiconic acid valerate cream, without any penetration, as shown in . In vivo pharmacodynamic results show that the anti-inflammatory effect of the SACPNs-based gel is better than that of diflumiconate valerate cream, and there is no change in the skin barrier function, as demonstrated by the plots in (Citation2013).
Figure 7. (a, b) SEM images of the clobetasol propionate-loaded SACPNs at 80 Kx (a) and 500 Kx (b) magnifications. (c) Histology of the rat skin samples: (c1) control; (c2) commercial cream; (c3) SACPNs; (c4) Na-DOC gel. (d) Amount of DFV accumulated from formulations in the SC + epidermis (dark bars) and dermis (light bars). (e) Changes in the paw thickness of the rats with respect to time after formulation applications. (a, b, c) Reproduced with permission (Şenyiğit et al., Citation2016). Copyright 2017, MDPI. (d, e) Reproduced with permission (Özcan et al., Citation2013). Copyright 2013, Dove Press.
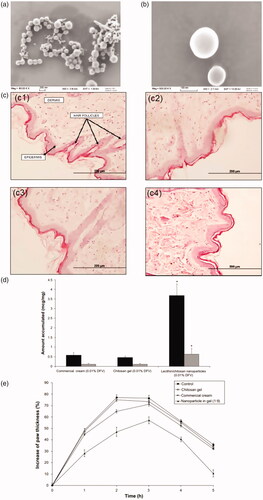
6.1.2 Mucosal delivery
Due to the mucoadhesive property of chitosan, SACPNs have been used extensively as a carrier for mucosal drug deliver (Baltzley et al., Citation2014), which can facilitate the drugs to act onset rapidly, avoiding the first pass effect, bypassing the blood-brain barrier and entering the central nervous system. Therefore, SACPNs can perform as vehicles for drugs that are not suitable for oral delivery with low bioavailability. Moreover, the positively charges on the surface of SACPNs are inclined to interact with the negatively charges on the mucus surface, which further facilitates the penetration of drugs through mucosal epithelial cells in vivo. For example, SACPNs loaded with simvastatin are prepared for nasal mucosal administration as a novel approach to deliver the statins to the brain. The optimized SACPNs can have an average diameter of 200 nm, positive surface charge (+48 mV) and a particular high encapsulation efficiency of simvastatin around 98%. The encapsulated simvastatin can be released in a quick manner in vitro with around 35.6% released in 6 hours, as shown in . Moreover, the MTT test results of human nasal epithelial cells show that the SACPNs have good cytocompatiblity while the cytotoxicity of simvastatin-loaded SACPNs (IC50) is found to be three times lower than the simvastatin suspension. In vivo studies have shown that after intranasal administration of simvastatin-loaded SACPNs, the 25% of delivered simvastatin can be distributed in the brain and the kidney, with few distribution in other tissues; while for the simvastatin suspension, the drug is mainly distributed in the lung, liver and kidney, but is rarely distributed in the brain, as demonstrated by the plots in . The results demonstrate that the SACPNs overcome the blood-brain barrier and significantly increase the distribution of the drug in the brain through the nasal cavity to the brain, with good biocompatibility and cytocompatibility (Clementino et al., Citation2016).
Figure 8. (a) Simvastatin release profile from simvastatin-loaded SACPNs (filled circle) and a control simvastatin suspension (open circle) in simulated nasal fluid (b) Radioactivity biodistribution in rats 90 minutes after the nasal instillation of 20 μL (10 μL in each nostril) of 99 mTc-labeled simvastatin-loaded SACPNs, simvastatin suspension, and pertechnetate (TcO4–) (a, b) Reproduced with permission (Clementino et al., Citation2016). Copyright 2016, Dove Press.
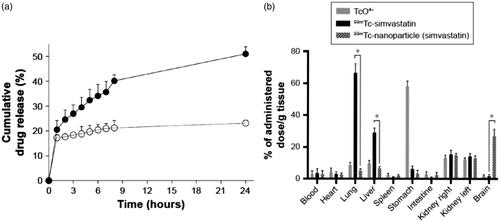
6.1.3 Ocular delivery
Chitosan can form disulfide bonds with mucus glycoprotein on the mucus gel layer, therefore improve precorneal retention and enhance the interaction with eye mucosa (Partenhauser & Bernkop-Schnürch, Citation2016). Moreover, chitosan also holds other special characteristics suitable for ocular drug delivery including nontoxic, low eye irritation, mucoadhesive, in situ gelling, transfection and permeation enhancing properties. All of these advantages contribute to the applications of SACPNs as suitable ocular deliver vehicles (Kapanigowda et al., Citation2015; Werle & Bernkop-Schnürch, Citation2008. Fox instance, amphotericin-B is encapsulated in SACPNs for prolonged ocular application (Chhonker et al., Citation2015). The prepared SACPNs are in the size range of 161.9–230.5 nm, entrapment efficiency of 70–75% with positive zeta potential of 26.6–38.3 mV. Moreover, the minimum inhibitory concentration (MIC) and inhibition zone size of the antibacterial in vitro are compared with commercial products. As demonstrated by antifungal susceptibility against Candida albicans and Aspergillus fumigatus, amphotericin-B-laded SACPNs exhibit pronounced mucoadhesive properties, as shown in , b and c). In vivo pharmacokinetic studies in rabbit eyes indicate the bioavailability and precorneal residence time can be improved by 2.04 folds and 3.36 folds, respectively, as demonstrated by the plots in . Therefore, the prepared amphotericin-B-laded SACPNs are of great significance for prolonging the acting duration of amphotericin B in eye medication and improving patient compliance.
Figure 9. (a, b) Zone of inhibition of amphotericin-B, Fungizone and amphotericin-B-laded SACPNs against A. fumigates. (c) Bar graphs represent zone of inhibition of different formulations against A. fumigates. (d) Cular pharmacokinetic profile of amphotericin-B following topical instillation in rabbit eyes. (a, b, c, d) Reproduced with permission (Chhonker et al., Citation2015). Copyright 2015, Elsevier.
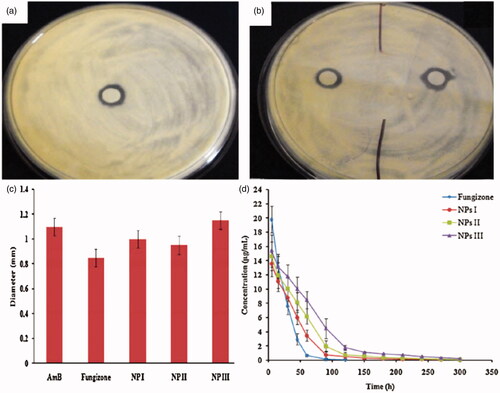
6.1.4 Oral delivery
Oral administration is the most convenient, least invasive, and minimum hepatic uptake among all the drug administration methods. However, the bioavailability of orally administered drug is always damaged because of their instabilities in the gastrointestinal tract and inefficient absorption due to low permeability across biological membranes. SACPNs have excellent mucoadhesiveness, which is beneficial to prolong the residence duration of the drug in the gastrointestinal mucosa; at the same time, SACPNs can open the tightly-packed intestinal epithelial cells, therefore can significantly improve the oral bioavailability of the drug. For instance, insulin-loaded SACPNs can be prepared through a modified solvent-injection method (Liu et al., Citation2016). Generated insulin-loaded SACPNs have a mean size of 180 nm, an insulin-entrapment efficiency of 94%, and an insulin-loading efficiency of 4.5%. In vitro analysis revealed that insulin-loaded SACPNs that are orally administered to streptozotocin-induced diabetic rats can exert a significant hypoglycemic effect, as demonstrated by the plots in . The relative pharmacological bioavailability following oral administration of SACPNs can be 6.01%. Therefore, by applying SACPNs, some hydrophilic peptides, such as insulin, can be successfully entrapped and delivered orally with improved oral bioavailability, time-dependent release, and therapeutic effects.
Figure 10. (a) In vitro time-dependent release profiles of insulin-loaded SACPNs with different phospholipid/chitosan ratios. (b) Time-dependent reduction in blood glucose levels in diabetic rats. (a, b) Reproduced with permission (Liu et al., Citation2016). Copyright 2016, Dove Press.
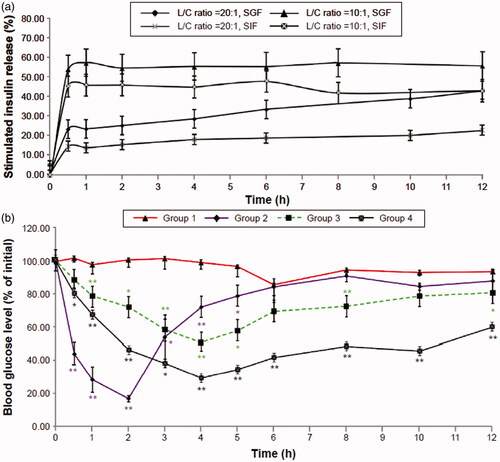
6.2 Other promising applications
6.2.1 Functional foods
Besides using as advanced drug delivery vehicles, SACPNs can also be applied as platforms for production of functional foods. For instance, curcumin encapsulated SACPNs can be obtained through solvent-injection method, as illustrated by the microscopic images in Citation2015. The in vitro antioxidant lipid peroxidation (TBARS), radical scavenging (DPPH, NO, H2O2, reducing power) activity assays of powdered curcumin and curcumin-loaded SACPNs are performed. It is found that the generated curcumin-loaded SACPNs are roughly spherical in shape, present high positive zeta potential (>30 mV) and stable in the pH range of 2–6. Moreover, they have enhanced antioxidant ability in comparison to curcumin aqueous suspension, as shown by the plots in . The curcumin-loaded SACPNs have great potential as functional food ingredient of natural origin.
Figure 11. (a) Morphological characterization of curcumin-loaded SACPNs by TEM (left) and SEM (right). (b) The inhibition of Nitric oxide activity (left) and H2O2 activity (right) in blank nanoparticles (Blank), curcumin powder (CP) and curcumin-loaded SACPNs. (a, b) Reproduced with permission (Pathak et al., Citation2015). Copyright 2015, Springer Nature.
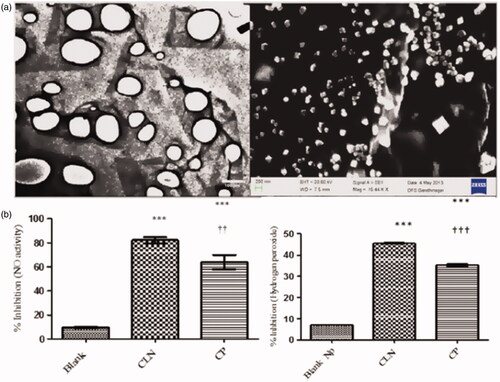
6.2.2 Antibacterial
Flavonoid compounds are strong antioxidant and antibacterial agents, but their applications are hampered due to their poor dissolution and bioavailability (Gupta et al., Citation2013; Ruesgas-Ramón et al., Citation2017). The use of SACPNs for the encapsulation and delivery of antibacterial agents has received increasing attentions. For instance, kaempferol (KAE) is loaded into SACPNs and compared with pure KAE to determine antifungal activity against the phytopathogenic fungus, as illustrated schematically and shown by the microscopic images in (Ilk et al., Citation2017). KAE can be successfully encapsulated in SACPNs with an efficiency of 93.8 ± 4.28% and the generated SACPNs show good physicochemical stability. Moreover, in vitro evaluation of the KAE-SACPNs is tested by the release kinetics, antioxidant and antifungal activity in a time-dependent manner against free KAE. Encapsulated KAE exhibited a significantly inhibition efficacy against Fusarium oxysporium during 60 days’ storage period, as demonstrated in . The results indicate that KAE-SACPNs can be used as suitable antibacterial agents and could solve the problems related to the solubility and loss of KAE during use and storage.
Figure 12. (a) Schematic illustration of KAE-SACPNs. (b) Morphology and dispersivity of KAE-SACPNs. (c) Effect of KAE-SACPNs and pure KAE on the radial growth of Fusarium oxysporium in time dependent manner. (a, b, c) Reproduced with permission (Ilk et al., Citation2017). Copyright 2016, Taylor & Francis Group.
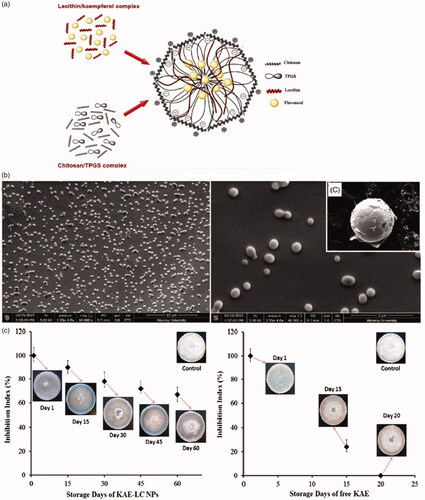
7. Conclusions and outlooks
7.1. Conclusions
The novel self-assembled chitosan/phospholipid nanoparticles (SACPNs) break through the limitation of drug hydrophobicity on vehicle construction, not only can improve the encapsulation efficiency, but also can increase the stability against the surrounding enzyme attack. The SACPNs are easy for preparation, simple for scale-up production, and can have high encapsulation efficiency by optimizing the generation process. SACPNs can have superior gastrointestinal mucosa and transdermal penetration, which have attracted widespread attention and can be used in a broad range of applications in drug delivery.
7.2. Outlooks
While a great number of SACPNs have been generated and applied in multiple areas, most SACPNs perform their functions based on the natural bioadhesion of chitosan and the regulation of tight junctions on epithelial cells, and therefore leave large spaces for further investigations. There are three major directions for the future developments of SACPNs that we believe worth stating and sharing with scientists who are interested in SACPNs: (1) proposing novel techniques to fabricate SACPNs; (2) investigating modifications to chitosan and phospholipid to functionalized SACPNs; (3) personalized designing of SACPNs based on unique characteristics of delivered drugs. Specifically, since conventional techniques are mainly conducted in bulk phase, more precisely control over the fabrication process needs further explorations to achieve more accurate regulation of the microstructure, size, and drug distribution of SACPNs. Microfluidics, as the emerging novel technique to precisely manipulate small volume of liquids, can be used as the suitable method for investigating the sophisticated control over the properties of SACPNs since it can increase the control of the entire fabrication process up to an unprecedented level (Ma et al., Citation2016; Ma et al., Citation2016; Ma et al., 2016; Deng et al., Citation2019). Moreover, chemical modifications of chitosan and phospholipid can be used to functionalize SACPNs to broaden its applications. For instance, near-infrared cyanine dyes can be used to modify chitosan. The resultant anticancer drug-loaded SACPNs can therefore generate active singlet oxygen under near-infrared irradiation and can be used as novel vehicles for the combination of chemotherapy and photodynamic therapy (Sharma et al., Citation2013; Liu et al. Citation2016; Cao et al., Citation2018, Citation2019). Furthermore, personalized design of SACPNs based on unique characteristics of delivered drugs is another major direction. For instance, By investigating the special properties of the delivered drugs and considering their specific application scenarios, SACPNs should be designed to be more versatile to deliver drugs more effectively toward powerful personalized medicines (Corbo et al., Citation2017, Citation2018).
Disclosure statement
No potential conflict of interest was reported by the authors.
Additional information
Funding
Reference
- Abdel-Mottaleb MM, Neumann D, Lamprecht A. (2011). Lipid nanocapsules for dermal application: a comparative study of lipid-based versus polymer-based nanocarriers. Eur. J. Pharm. Biopharm 79:36–42.
- Abdolhi N, Soltani A, Fadafan HK, et al. (2017). Preparation, characterization and toxicity evaluation of Co3O4 and NiO-filled multi-walled carbon nanotubes loaded to chitosan. Nano-Structures & Nano-Objects 12:182–7.
- Alkholief M. (2019). Optimization of Lecithin-Chitosan nanoparticles for simultaneous encapsulation of doxorubicin and piperine. J. Drug Deliv. Sci. Technol 52:204–14.
- Arias JL, López-Viota M, Delgado ÁV, Ruiz MA. (2010). Iron/ethylcellulose (core/shell) nanoplatform loaded with 5-fluorouracil for cancer targeting. Colloids Surf. B. Biointerfaces 77:111–6.
- Attama A, Schicke B, Paepenmüller T, Müller-Goymann C. (2007). Solid lipid nanodispersions containing mixed lipid core and a polar heterolipid: characterization. Eur. J. Pharm. Biopharm 67:48–57.
- Augustine R, Dan P, Schlachet I, et al. (2019). Chitosan ascorbate hydrogel improves water uptake capacity and cell adhesion of electrospun poly (epsilon-caprolactone) membranes. Int J Pharma 559:420–6.
- Bahadori M, Mohammadi F. (2006). Nanomedicine. Iran J Pathol 1:41–8.
- Bajpai A, Gupta R. (2011). Magnetically mediated release of ciprofloxacin from polyvinyl alcohol based superparamagnetic nanocomposites. J Mater Sci: Mater Med 22:357–69.
- Baltzley S, Mohammad A, Malkawi AH, Al-Ghananeem AM. (2014). Intranasal drug delivery of olanzapine-loaded chitosan nanoparticles. AAPS PharmSciTech 15:1598–602.
- Barbieri S, Sonvico F, Como C, et al. (2013). Lecithin/chitosan controlled release nanopreparations of tamoxifen citrate: Loading, enzyme-trigger release and cell uptake. J Con Release 167:276–83.
- Barratt GM. (2000). Therapeutic applications of colloidal drug carriers. Pharma Sci Technol Today 3:163–71.
- Battaglia L, Gallarate M. (2012). Lipid nanoparticles: state of the art, new preparation methods and challenges in drug delivery. Expert Opin Drug Del 9:497–508.
- Bhatta RS, Chandasana H, Chhonker YS, et al. (2012). Mucoadhesive nanoparticles for prolonged ocular delivery of natamycin: in vitro and pharmacokinetics studies. Int J Pharma 432:105–12.
- Cao J, Chi J, Xia J, et al. (2019). Iodinated cyanine dyes for fast near-infrared-guided deep tissue synergistic phototherapy. ACS Appl Mater Interfaces 11:25720–9.
- Cao J, Ge R, Zhang M, et al. (2018). A triple modality BSA-coated dendritic nanoplatform for NIR imaging, enhanced tumor penetration and anticancer therapy. Nanoscale 10:9021–37.
- Cardoso PB, Machado TO, Feuser PE, et al. (2018). Biocompatible polymeric nanoparticles from castor oil derivatives via thiol‐ene miniemulsion polymerization. Eur J Lipid Sci Technol 120:1700212.
- Chadha R, Gupta S, Pathak N. (2012). Artesunate-loaded chitosan/lecithin nanoparticles: preparation, characterization, and in vivo studies. Drug Dev Ind Pharm 38:1538–46.
- Chaouat C, Balayssac S, Malet-Martino M, et al. (2017). Green microparticles based on a chitosan/lactobionic acid/linoleic acid association. Characterisation and evaluation as a new carrier system for cosmetics. J Microencapsul 34:162–70.
- Chhonker YS, Prasad YD, Chandasana H, et al. (2015). Amphotericin-B entrapped lecithin/chitosan nanoparticles for prolonged ocular application. Int J Bio Macromol 72:1451–8.
- Cho H-J, Yoon I-S, Yoon HY, et al. (2012). Polyethylene glycol-conjugated hyaluronic acid-ceramide self-assembled nanoparticles for targeted delivery of doxorubicin. Biomaterials 33:1190–200.
- Chuah AM, Kuroiwa T, Ichikawa S, et al. (2009). Formation of biocompatible nanoparticles via the self‐assembly of chitosan and modified lecithin. J Food Sci 74:N1–N8.
- Clementino A, Batger M, Garrastazu G, et al. (2016). The nasal delivery of nanoencapsulated statins–an approach for brain delivery. Indian J. Nephro 11:6575–90.
- Clementino A, Pozzoli M, Botti E, Sonvico F. (2018). Lecithin/chitosan-based nanoparticles for the nasal delivery of statins: enzyme-trigger controlled release and cellular transport. Respiratory Drug Del 2:589–594.
- Corbo C, Mahmoudi M, Farokhzad OC. (2018). Personalized cancer-specific protein corona affects the therapeutic impact of nanoparticles. Am Assoc Cancer Res 5:378–387.
- Corbo C, Molinaro R, Tabatabaei M, et al. (2017). Personalized protein corona on nanoparticles and its clinical implications. Biomater Sci 5:378–87.
- Deng Y, Ma Q, Yuan H, et al. (2019). Development of dual-component protein microparticles in all-aqueous systems for biomedical applications. J Mater Chem B 7:3059–65.
- Di Crescenzo A, Velluto D, Hubbell JA, Fontana A. (2011). Biocompatible dispersions of carbon nanotubes: a potential tool for intracellular transport of anticancer drugs. Nanoscale 3:925–8.
- Di Pasqua AJ, Wallner S, Kerwood DJ, Dabrowiak JC. (2009). Adsorption of the Pt(II) anticancer drug carboplatin by mesoporous silica. Chem Biodivers 6:1343–9.
- Dutta T, Ghosh NN, Chattopadhyay AP, Das M. (2019). Chitosan encapsulated water-soluble silver bionanocomposite for size-dependent antibacterial activity. Nano-Structures & Nano-Objects 20:100393.
- Eid M, El-Hallouty S, El-Manawaty M, et al. (2018). Preparation conditions effect on the physico-chemical properties of magnetic–plasmonic core–shell nanoparticles functionalized with chitosan: green route. Nano-Structures & Nano-Objects 16:215–23.
- Ejeromedoghene O, Adewuyi S, Amolegbe SA, et al. (2018). Electrovalent chitosan functionalized methyl-orange/metal nanocomposites as chemosensors for toxic aqueous anions. Nano-Structures & Nano-Objects 16:174–9.
- Gerelli Y, Di Bari M, Deriu A, et al. (2008). Structure and organization of phospholipid/polysaccharide nanoparticles. J Phys Condens Mat 20:104211.
- Ghosal K, Ghosh D, Das SK. (2018). Preparation and evaluation of naringin-loaded polycaprolactone microspheres based oral suspension using Box-Behnken design. J. Mol. Liq 256:49–57.
- Goldberg M, Langer R, Jia X. (2007). Nanostructured materials for applications in drug delivery and tissue engineering. J Biomat Sci 18:241–68.
- Grant J, Blicker M, Piquette-Miller M, Allen C. (2005). Hybrid films from blends of chitosan and egg phosphatidylcholine for localized delivery of paclitaxel. J Pharma Sci 94:1512–27.
- Gupta A, Kagliwal LD, Singhal RS. (2013) Biotransformation of polyphenols for improved bioavailability and processing stability. Adv. Food Nutr. Res 69:183–217.
- Hafner A, Dürrigl M, Pepić I, Filipović-Grčić J. (2011). Short- and long-term stability of lyophilised melatonin-loaded lecithin/chitosan nanoparticles. Chem Pharm Bull 59:1117–23.
- He Q, Gao Y, Zhang L, et al. (2011). A pH-responsive mesoporous silica nanoparticles-based multi-drug delivery system for overcoming multi-drug resistance. Biomaterials 32:7711–20.
- Hua M-Y, Yang H-W, Chuang C-K, et al. (2010). Magnetic-nanoparticle-modified paclitaxel for targeted therapy for prostate cancer. Biomaterials 31:7355–63.
- Ichikawa S, Iwamoto S, Watanabe J. (2005). Formation of biocompatible nanoparticles by self-assembly of enzymatic hydrolysates of chitosan and carboxymethyl cellulose. Biosci Biotechnol Biochem 69:1637–42.
- Ilk S, Saglam N, Özgen M. (2017). Kaempferol loaded lecithin/chitosan nanoparticles: preparation, characterization, and their potential applications as a sustainable antifungal agent. Artif Cells Nanomed Biotechnol 45:907–16.
- Joshy K, Sharma CP. (2012). Blood Compatible nanostructured lipid carriers for the enhanced delivery of azidothymidine to brain. Adv Sci Lett 6:47–55.
- Joshy KS, Snigdha S, Anne G, et al. (2018). Poly (vinyl pyrrolidone)-lipid based hybrid nanoparticles for anti viral drug delivery. Chem. Phys. Lipids 210:82–9.
- Joshy K, Snigdha S, Kalarikkal N, et al. (2017). Gelatin modified lipid nanoparticles for anti-viral drug delivery. Chem. Phys. Lipids 207:24–37.
- Joshy K, Susan MA, Snigdha S, et al. (2018). Encapsulation of zidovudine in PF-68 coated alginate conjugate nanoparticles for anti-HIV drug delivery. Int J Biolog Macromol 107:929–37.
- Jourghanian P, Ghaffari S, Ardjmand M, et al. (2016). Sustained release curcumin loaded solid lipid nanoparticles. Adv Pharm Bull 6:17–21.
- Kapanigowda UG, Nagaraja SH, Ramaiah B, Boggarapu PR. (2015). Improved intraocular bioavailability of ganciclovir by mucoadhesive polymer based ocular microspheres: development and simulation process in Wistar rats. Daru J Pharm Sci 23:49.
- Kaur IP, Bhandari R, Bhandari S, Kakkar V. (2008). Potential of solid lipid nanoparticles in brain targeting. J Con Rel 127:97–109.
- Khan MM, Madni A, Torchilin V, et al. (2019). Lipid-chitosan hybrid nanoparticles for controlled delivery of cisplatin. Drug Delivery 26:765–72.
- Lawrie G, Keen I, Drew B, et al. (2007). Interactions between alginate and chitosan biopolymers characterized using FTIR and XPS. Biomacromolec 8:2533–41.
- Li L, Raghupathi K, Song C, et al. (2014). Self-assembly of random copolymers. Chemical Communications 50:13417–32.
- Li Z, Su K, Cheng B, et al. (2010). Organically modified MCM-type material preparation and its usage in controlled amoxicillin delivery. J. Colloid Interface Sci 342:607–13.
- Liu J, Gong T, Fu H, et al. (2008). Solid lipid nanoparticles for pulmonary delivery of insulin. Int J Pharma 356:333–44.
- Liu M, Zhang J, Shan W, Huang Y. (2015). Developments of mucus penetrating nanoparticles. Asian J Pharma Sci 10:275–82.
- Liu M, Zhang J, Zhu X, et al. (2016). Efficient mucus permeation and tight junction opening by dissociable “mucus-inert” agent coated trimethyl chitosan nanoparticles for oral insulin delivery. J Con Release 222:67–77.
- Liu L, Zhou C, Xia X, Liu Y. (2016). Self-assembled lecithin/chitosan nanoparticles for oral insulin delivery: preparation and functional evaluation. Int J Nanomedicine 11:761–9.
- Luo X, Matranga C, Tan S, et al. (2011). Carbon nanotube nanoreservior for controlled release of anti-inflammatory dexamethasone. Biomaterials 32:6316–23.
- Manuja A, Dilbaghi N, Kaur H, et al. (2018). Chitosan quinapyramine sulfate nanoparticles exhibit increased trypanocidal activity in mice. Nano-Structures & Nano-Objects 16:193–9.
- Ma Q, Song Y, Baier G, et al. (2016). Osmo-solidification of all-aqueous emulsion with enhanced preservation of protein activity. J Mater Chem B 4:1213–8.
- Ma Q, Song Y, Kim JW, et al. (2016). Affinity partitioning-induced self-assembly in aqueous two-phase systems: templating for polyelectrolyte microcapsules. ACS Macro Lett 5:666–70.
- Mathew S, Snigdha S, Mathew J, Radhakrishnan E. (2018). Poly (vinyl alcohol): montmorillonite: boiled rice water (starch) blend film reinforced with silver nanoparticles; characterization and antibacterial properties. Applied Clay Science 161:464–73.
- Ma Q, Yuan H, Song Y, et al. (2018). Partitioning-dependent conversion of polyelectrolyte assemblies in an aqueous two-phase system. Soft Matter 14:1552–8.
- Moraru CI, Panchapakesan CP, Huang Q, et al. (2003). Facets of Nanotechnology as Seen in Food Processing, Packaging, and Preservation Industry. Nanotechnol 57:24–29.
- Moreno E, Schwartz J, Larrea E, et al. (2015). Assessment of β-lapachone loaded in lecithin-chitosan nanoparticles for the topical treatment of cutaneous leishmaniasis in L. major infected BALB/c mice. Nanomed Nanotechnol Biol Med 11:2003–12.
- Muchow M, Maincent P, Müller RH. (2008). Lipid nanoparticles with a solid matrix (SLN®, NLC®, LDC®) for oral drug delivery. Drug Dev Ind Pharm 34:1394–405.
- Narayanan D, Ninan N, Jayakumar R, et al. (2014). O-carboxymethyl chitosan nanoparticles for controlled release of non-steroidal anti-inflammatory drugs. Adv Sci Engng Med 6:522–30.
- Naskar S, Kuotsu K, Sharma S. (2019). Chitosan-based nanoparticles as drug delivery systems: a review on two decades of research. J. Drug Targeting 27:379–93.
- Nathanson M, Kanhaiya K, Pryor A, Jr, et al. (2018). Atomic-Scale structure and stress release mechanism in core–shell nanoparticles. ACS Nano 12:12296–304.
- Nayak AP, Tiyaboonchai W, Patankar S, et al. (2010). Curcuminoids-loaded lipid nanoparticles: novel approach towards malaria treatment. Colloids Surf. B. Biointerfaces 81:263–73.
- Nosrati H, Sefidi N, Sharafi A, et al. (2018). Bovine serum albumin (BSA) coated iron oxide magnetic nanoparticles as biocompatible carriers for curcumin-anticancer drug. Bioorg. Chem 76:501–9.
- Ochekpe NA, Olorunfemi PO, Ngwuluka NC. (2009). Nanotechnology and drug delivery part 2: nanostructures for drug delivery. Trop J Pharm Res 8:276.
- Özcan I, Azizoğlu E, Senyiğit T, et al. (2013). Enhanced dermal delivery of diflucortolone valerate using lecithin/chitosan nanoparticles: in-vitro and in-vivo evaluations. Int J Nanomedicine 8:461–75,
- Pandey R, Ahmad Z, Sharma S, Khuller G. (2005). Nano-encapsulation of azole antifungals: potential applications to improve oral drug delivery. Int J Pharma 301:268–76.
- Panos I, Acosta N, Heras A. (2008). New drug delivery systems based on chitosan. Curr Drug Discov Technol 5:333–41.
- Park K, Kim K, Kwon IC, et al. (2004). Preparation and characterization of self-assembled nanoparticles of heparin-deoxycholic acid conjugates. Langmuir 20:11726–31.
- Partenhauser A, Bernkop-Schnürch A. (2016). Bernkop-Schnuerch, A. Mucoadhesive polymers in the treatment of dry X syndrome. Drug Discovery Today 21:1051–62.
- Pathak L, Kanwal A, Agrawal Y. (2015). Curcumin loaded self assembled lipid-biopolymer nanoparticles for functional food applications. J Food Sci Technol 52:6143–56.
- Popovici R, Seftel E, Mihai G, et al. (2011). Controlled drug delivery system based on ordered mesoporous silica matrices of captopril as angiotensin-converting enzyme inhibitor drug. J Pharma Sci 100:704–14.
- Quiñones JP, Peniche H, Peniche C. (2018). Chitosan based self-assembled nanoparticles in drug delivery. Polymers 10:235.
- Raza ZA, Anwar F. (2017). Fabrication of chitosan nanoparticles and multi-response optimization in their application on cotton fabric by using a Taguchi approach. Nano-Structures & Nano-Objects 10:80–90.
- Rejinold NS, Chennazhi K, Nair S, et al. (2011). Biodegradable and thermo-sensitive chitosan-g-poly (N-vinylcaprolactam) nanoparticles as a 5-fluorouracil carrier. Carbohydr. Polym 83:776–86.
- Rodriguez-Hernandez J, Chécot F, Gnanou Y, Lecommandoux S. (2005). Toward ‘smart’nano-objects by self-assembly of block copolymers in solution. Prog Polym Sci 30:691–724.
- Ruesgas-Ramón M, Figueroa-Espinoza MC, Durand E. (2017). Application of deep eutectic solvents (DES) for phenolic compounds extraction: overview, challenges, and opportunities. J Agric Food Chem 65:3591–601.
- Duttagupta DS, Jadhav VM, Kadam VJ. (2015). Chitosan: a propitious biopolymer for drug delivery. Curr Drug Deliv 12:369–81.
- Sahoo SK, Dilnawaz F, Krishnakumar S. (2008). Nanotechnology in ocular drug delivery. Drug Discovery Today 13:144–51.
- Sanguansri P, Augustin MA. (2006). Nanoscale materials development–a food industry perspective. Trends Food Sci Tech 17:547–56.
- Saraogi GK, Gupta P, Gupta U, et al. (2010). Gelatin nanocarriers as potential vectors for effective management of tuberculosis. Int J Pharma 385:143–9.
- Sato AK, Viswanathan M, Kent RB, Wood CR. (2006). Therapeutic peptides: technological advances driving peptides into development. Cur Opin Biotech 17:638–42.
- Schatz C, Lucas J-M, Viton C, et al. (2004). Formation and properties of positively charged colloids based on polyelectrolyte complexes of biopolymers. Langmuir 20:7766–78.
- Schug KA, Lindner W. (2005). Noncovalent binding between guanidinium and anionic groups: focus on biological-and synthetic-based arginine/guanidinium interactions with phosph [on] ate and sulf [on] ate residues. Chem Rev 105:67–114.
- Şenyiğit T, Sonvico F, Barbieri S, et al. (2010). Lecithin/chitosan nanoparticles of clobetasol-17-propionate capable of accumulation in pig skin. J Con Release 142:368–73.
- Şenyiğit T, Sonvico F, Rossi A, et al. (2016). In vivo assessment of clobetasol propionate-loaded lecithin-chitosan nanoparticles for skin delivery. Int J Mol Sci 18:32.
- Shanmugam T, Banerjee R. (2011). Nanostructured self assembled lipid materials for drug delivery and tissue engineering. Ther Deliv 2:1485–516.
- Sharma K, Somavarapu S, Colombani A, et al. (2013). Nebulised siRNA encapsulated crosslinked chitosan nanoparticles for pulmonary delivery. Int J Pharmaceutics 455:241–7.
- Son G-H, Lee B-J, Cho C-W. (2017). Mechanisms of drug release from advanced drug formulations such as polymeric-based drug-delivery systems and lipid nanoparticles. J Pharma Investigation 47:287–96.
- Sonvico F, Cagnani A, Rossi A, et al. (2006). Formation of self-organized nanoparticles by lecithin/chitosan ionic interaction. Int J Pharma 324:67–73.
- Sonvico F, Di Bari MT, Bove L, et al. (2006). Mean square hydrogen fluctuations in chitosan/lecithin nanoparticles from elastic neutron scattering experiments. Physica B 385:725–7.
- Sonvico F, Dubernet C, Colombo P, Couvreur P. (2005). Metallic colloid nanotechnology, applications in diagnosis and therapeutics. Curr Pharm Des 11:2091–105.
- Sznitowska M, Gajewska M, Janicki S, et al. (2001). Bioavailability of diazepam from aqueous-organic solution, submicron emulsion and solid lipid nanoparticles after rectal administration in rabbits. Eur. J. Pharm. Biopharm 52:159–63.
- Tan Q, Liu W, Guo C, Zhai G. (2011). Preparation and evaluation of quercetin-loaded lecithin-chitosan nanoparticles for topical delivery. Int J Nanomed 6:1621–30.
- Terrón-Mejía K, Martínez-Benavidez E, Higuera-Ciapara I, et al. (2018). Mesoscopic modeling of the encapsulation of capsaicin by lecithin/chitosan liposomal nanoparticles. Nanomaterials 8:425.
- Tong Q, Li H, Li W, et al. (2011). In vitro and in vivo anti-tumor effects of gemcitabine loaded with a new drug delivery system. J Nanosci Nanotech 11:3651–8.
- Torchilin VP. (2005). Recent advances with liposomes as pharmaceutical carriers. Nat Rev Drug Discov 4:145–60.
- Tripisciano C, Costa S, Kalenczuk R, Borowiak-Palen E. (2010). Cisplatin filled multiwalled carbon nanotubes–a novel molecular hybrid of anticancer drug container. Eur Phys J B 75:141–6.
- Van der Lubben I, Verhoef J, Borchard G, Junginger H. (2001). Chitosan for mucosal vaccination. Adv Drug Deliv Rev 52:139–44.
- Vauthier C, Bouchemal K. (2009). Methods for the preparation and manufacture of polymeric nanoparticles. Pharm Res 26:1025–58.
- Werle M, Bernkop-Schnürch A. (2008). Thiolated chitosans: useful excipients for oral drug delivery. J Pharma Pharmacol 60:273–81.
- Wydro P, Krajewska B, Ha̧c-Wydro K. (2007). Chitosan as a lipid binder: a Langmuir monolayer study of chitosan − lipid interactions. Biomacromolecules 8:2611–7.
- Yang J, Park S-B, Yoon H-G, et al. (2006). Preparation of poly ɛ-caprolactone nanoparticles containing magnetite for magnetic drug carrier. Int J Pharma 324:185–90.
- Yoo J-W, Irvine DJ, Discher DE, Mitragotri S. (2011). Bio-inspired, bioengineered and biomimetic drug delivery carriers. Nat Rev Drug Discov 10:521–35.
- Yoo HS, Lee JE, Chung H, et al. (2005). Self-assembled nanoparticles containing hydrophobically modified glycol chitosan for gene delivery. J Con Release 103:235–43.
- Yuan W, He X, Zhou X, Zhu Y. (2018). Hydroxyapatite nanoparticle-coated 3D-printed porous Ti6Al4V and CoCrMo alloy scaffolds and their biocompatibility to human osteoblasts. J Nanosci Nanotechnol 18:4360–5.
- Zhang L, Chan JM, Gu FX, et al. (2008). Self-assembled lipid − polymer hybrid nanoparticles: a robust drug delivery platform. ACS Nano 2:1696–702.
- Zhang J, Coulston RJ, Jones ST, et al. (2012). One-step fabrication of supramolecular microcapsules from microfluidic droplets. Science 335:690–4.
- Zhang D, Pan B, Wu M, et al. (2011). Adsorption of sulfamethoxazole on functionalized carbon nanotubes as affected by cations and anions. Environ. Pollut 159:2616–21.
- Zhou Y, Dong W, Ye J, et al. (2017). A novel matrix dispersion based on phospholipid complex for improving oral bioavailability of baicalein: preparation, in vitro and in vivo evaluations. Drug Delivery 24:720–8.