Abstract
Growth factors are multi-functional signaling molecules that coordinate multi-stage process of wound healing. During wound healing, growth factors are transmitted to wound environment in a positive and physiologically related way, therefore, there is a broad prospect for studying the mediated healing process through growth factors. However, growth factors (GFs) themselves have disadvantages of instability, short life, rapid inactivation of physiological conditions, low safety and easy degradation, which hinder the clinical use of GFs. Rapid development of delivery strategies for GFs has been trying to solve the instability and insecurity of GFs. Particularly, in recent years, GFs delivered by scaffolds based on biomaterials have become a hotspot in this filed. This review introduces various delivery strategies for growth factors based on new biodegradable materials, especially polysaccharides, which could provide guidance for the development of the delivery strategies for growth factors in clinic.
1. Introduction
Growth factors (GF) are soluble proteins, which can participate in cell growth, proliferation, differentiation, migration, etc (Qian et al., Citation2017; Wang et al., Citation2017). They are derived from kinds of cells, including macrophages, fibroblasts, and so on, which coordinate immune responses, angiogenesis, and wound healing (Gallego-Muñoz et al., Citation2017; Duncan et al., Citation2018; Ishihara et al., Citation2018). They exert functions via specifically binding with receptors on the surface of cell membrane and participate in cell proliferation, differentiation and migration (Surmacz, Citation2003; Davydova et al., Citation2016; Cipitria & Salmeron-Sanchez, Citation2017). They have important applications in wound healing, skin tissue engineering, cartilage tissue engineering, and bone tissue engineering (Jia et al., Citation2016; Millette et al., Citation2006; Caballero Aguilar et al., Citation2019). For instance, vascular endothelial growth factor (VEGF) and fibroblast growth factor (FGF) families can promote cell migration and proliferation of endothelial cells; platelet-derived growth factor-BB (PDGF-BB) stimulate pericyte adhesion and blood vessel maturation, including stem cell recruitment and granulation tissue formation (Hellberg et al., Citation2010); The hepatocyte growth factor (HGF) can modulate the proliferation, migration and differentiation of mesenchymal stem cells (Catizone et al., Citation2006). Growth factors have various specific biological characteristics so that clinical applications are also different ().
Table 1. Growth factors and their clinical applications in clinical practice.
Growth factors facilitate the proliferation and differentiation of progenitor and stem cells, as well as directly induce growth of differentiated cells such as hepatocytes in the liver or osteoblasts in bone (Uebersax et al., Citation2009). They also have an important regulatory role in human immunity, hematopoietic regulation, tumorigenesis, inflammatory infection, wound healing, angiogenesis, cell differentiation, cell apoptosis, morphogenesis, and embryo formation (Arisaka & Yui, Citation2019; Chu et al., Citation2019; Evans et al., Citation2019). Growth factors mainly include epidermal growth factor (EGF), platelet-derived growth factor (PDGF), fibroblast growth factor (FGF), and transforming growth factor beta (TGF-β) families (Goh et al., Citation2016). In the past decades, many researches try to uncover more GFs functions in biomedical science and delivery of GFs using biomaterials has become a pretty hot topic. Currently, numerous researches have shown that scaffolds based on biomaterials could delivery growth factors to promote tissue repair and regeneration at a faster rate (Venkatesan et al., Citation2017). However, growth factors for clinical applications achieved little clinical success, which were still limited by the instability and safety of GFs (Nicoletti et al., Citation2019). The key to overcoming the challenges lies in better deliver GFs, maintain their activities and alleviate their adverse effects.
Although there are extensive studies on the safety and efficacy of growth factors in clinical trials, there are significant limitations associated with the use of growth factors, including ectopic bone formation, osteolysis, pain and swelling. Given the long-term regeneration process in live organism, it is difficult to resolve the contradiction between efficacy and adverse reactions in current studies. Clinically, excessive soluble protein or even milligram level (one million times the concentration required for physiological regeneration) is usually used to ensure clinical effectiveness (Caballero Aguilar et al., Citation2019). However, high doses of soluble growth factors will inevitably spread to nearby tissues or blood circulation, bringing many adverse effects (Caballero Aguilar et al., Citation2019). Although many carriers have adapted to control initial burst and rapid clearance of GFs, safety and efficacy of GFs remain mutually exclusive. The cost-effectiveness and legal issues associated with soluble recombinant growth factors are now challenging in the field of medicine. Precise delivery and safe release of growth factors have emerged as a promising spot in tissue engineering. Biomacromolecules including various polysaccharides have been widely studied as novel biomaterial scaffolds for GF delivery in regenerative medicine (Shelke et al., Citation2014; Degirolamo et al., Citation2016; Zhang et al., Citation2018). To date, researchers have designed a variety of biomaterial-based delivery systems to enhance tissue regeneration by maintaining high levels of GFs over a long period of time in situ (Hendrikse et al., Citation2018; Zheng et al., Citation2018; Guo et al., Citation2019). The polysaccharides and other biomacromolecules were developed into various nanofibers, scaffolds, sponges, microspheres, nanoparticles, and composites, which play a crucial role in the delivery of growth factors (Kumar et al., Citation2018; Miao et al., Citation2018; Tiwari et al., Citation2018). On the one hand, because of their biochemical similarity with human extracellular matrix (ECM) components, these biomaterials are readily recognized and tolerated by native body, on the other hand, natural biomaterials inherit numerous advantages including natural abundance and adequate capacity for chemical modification to meet varying technological requirements. In this review, we will focus on the biomaterials-based approaches to improve the delivery of GFs in tissue engineering, with the emphasis on the control of GFs activities using natural polysaccharides ().
2. Applications of natural polysaccharides in tissue engineering
Natural polysaccharides for tissue engineering are widely used for treating human damaged organs and dying tissues, discovering tissue engineered substitutes is the key in clinic (Pearman et al., Citation2019; Vandghanooni & Eskandani, Citation2019). However, there are still huge challenges in applications, the scaffolds must provide appropriate mechanical and chemical properties to guide appropriate cell behavior. Further, they enable regeneration and replacement of the necessary biological functions. The extracellular matrix of the implanted area is the ideal template and ECM has many unique properties including guiding cell migration, adhesion and differentiation, at the same time, it can be degraded and rebuilt as needed. Natural polysaccharides exhibit structure and function similarity with ECM (Hinz, Citation2015; Erdogan & Webb, Citation2017), as the extracellular matrix mainly consists of protein polysaccharides, glycosaccharides, glycoproteins, and glycolipids. Meanwhile, natural polysaccharides have good biocompatibility and biodegradability, which are more and more widely used in the field of tissue engineering (Le Dour et al., Citation2017; Yan et al., Citation2018). The natural polysaccharide, like cellulose, has the strong mechanical strength and biocompatibility, which can be applied for tissue engineering, including engineering vascular tissue, bone, cartilage, skeletal muscle, and cardiac muscle. Additionally, cellulose also can be used to establish nano-fibrous carrier for liver cells and create tubes for regeneration. The chitosan as a natural polysaccharide also can be modified into scaffolds in tissue engineering, which exhibits the significant effect on supporting and aiding the generation of extracellular matrix in vivo. The alginate derived from sea can be changed into gels and applied for cell transplantation in tissue engineering. Therefore, a large volume of research suggests that polysaccharides may interact with tissue cells and regulate these cells in diverse ways. Then the polysaccharides can be modified into versatile scaffolds in tissue engineering (Li et al., Citation2016; Wang et al., Citation2017) ().
Table 2. Various polysaccharides from nature.
2.1. Animal-derived polysaccharides for controlling growth factors
Polysaccharides extracted from animals play a vital role in growth factors delivery. The extracellular matrix in animal tissues is composed of interlocking reticular structures, which is filled with heteropolysaccharides, fibrins, and gelatinous substances, supporting cell adhesion, and providing porous pathways for the diffusion of nutrients and oxygen for individual cells (Bhaskar et al., Citation2012). For instance, heteropolysaccharides, called glycosaminoglycans, are repeated by disaccharide units, which include hyaluronic acid, heparin, heparin sulfate, chondroitin sulfate (CS), piperin sulfate, and keratin sulfate (DeAngelis, Citation2012). Additionally, chitosan and chitin derived from animals are also widely used for growth factors delivery in tissue engineering.
2.1.1. Chitosan and chitin
Chitosan is a deacetylated derivative of chitin extracted from arthropods, usually in the form of particles, flakes, or powders. Both chitosan and chitin are linear polysaccharides consisting of repeated n-acetyl-2-amino-2-deoxyd-glucose (n-acetyl) and 2-amino-2-deoxyd-d-glucose residues (n-deacetyl, amino) (Venkatesan et al., Citation2015). Numerous studies have shown that chitosan and chitin can be used in a variety of applications in tissue engineering (Yilgor et al., Citation2009; Liu et al., Citation2016; Pangon et al., Citation2016; Islam et al., Citation2017; Ahsan et al., Citation2018). They can be designed into a variety of structures such as gels, membranes nanofibers, nanoparticle and sponges (Singh et al., Citation2017; Smirnova et al., Citation2019; Zubillaga et al., Citation2020). In combination with properties such as its good biocompatibility, biodegradability, nontoxic, anti-inflammatory, adhesion, antibacterial and nerve protection, chitosan can be made into a suitable candidate for growth factors delivery (Rajam et al., Citation2011). Furthermore, being one of the few natural polycationic polysaccharides, it permits chitosan to have many electrostatic interactions that can be leveraged for the production of biomaterials (such as in layer-by-layer polyelectrolyte assemblies). These chitosan based materials can easily be made into the shape needed for tissue support and regeneration scaffolds. For example, Azizian et al. claimed that chitosan nanoparticles loaded with basic fibroblast growth factor (bFGF) and bovine serum albumin (BSA) was introduced into the chitosan-gelatin scaffold, the experimental results showed that chitosan nanoparticles significantly affected the property of the scaffold, which could continuously release growth factors to promote the proliferation of fibroblasts (Azizian et al., Citation2018). This experiment incited a growing interest for chitosan - gelatin scaffold and provided a reference for delivering growth factors, which could be widely used in bone tissue engineering and provide a good matrix for bone tissue regeneration and repair. Venkatesan et al. reported that chitosan had the ability to increase alkaline phosphatase activity, enhance serum calcium concentration, and promote osteoblast differentiation and bmp-2 expression in rabbit models (Venkatesan et al., Citation2017). And Gohil et al. demonstrated that chitosan hydrophobic gel has great potential in delivering growth factors and proteins. The presence of amino and hydroxyl functional groups in chitosan plays an important role in the release of growth factors in the extracellular matrix. Chitosan combines with negatively charged biomolecules through electrostatic action of positive charge generated by amino protonation in the chain to control the release of encapsulating proteins (Gohil et al., Citation2017). Additionally, Song et al. (Oh & Lee, Citation2019) indicated that the combination of chitosan and silica dressing loaded with keratinocyte growth factor (KGF) had the characteristics of continuous KGF release. The results have shown that the dressing promotes skin regeneration. The chitosan scaffold found in the present study is mainly used to load growth factors, which can also be used in combination with other materials. The combination of heparin and chitosan to form scaffold - supporting nerve growth factor (NGF) was used to promote nerve regeneration. Li et al. (Citation2017) found that heparin/chitosan scaffold loaded with NGF significantly improved cell adhesion and proliferation. More importantly, the NGF heparin/chitosan scaffold can effectively promote cell morphological development. Sara et al. (Azizian et al., Citation2018) prepared the chitosan nanoparticles, which were synthesized by ionic gelation method (average size 266 nm), loaded with BSA-bFGF, and were incorporated into the 3 D porous chitosan-gelatin scaffold. The system achieved the sustained release of growth factors from the scaffold and extremely promoted fibroblast cell proliferation in vitro ().
Figure 2. (a) A schematic of chitosan nanoparticles prepared by ionic gelation method; (b) SEM showed the chitosan nanoparticles were incorporated within the chitosan-gelatin scaffold; (c) MTT assay for fibroblast cells cultured on scaffolds after 1, 3 and 7 days (**p < .01); (d) SEM image of fibroblast cells attached to the chitosan nanoparticles loading growth factors in chitosan-gelatin scaffold on day 7 after culture; The blue arrow stands for the attached cells (Azizian et al., Citation2018).
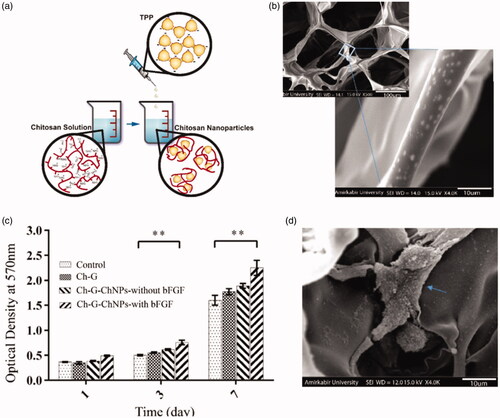
2.1.2. Hyaluronic acid
Hyaluronic acid (HA), an acidic polysaccharide, was isolated from bovine vitreous in 1934 by Meyer et al., a professor of ophthalmology at Columbia University, which is a non-sulfated glycosaminoglycan and major component of the extracellular matrix (Necas et al., Citation2008; Mangla et al., Citation2017). Hyaluronan (or hyaluronic acid) is a linear poly-saccharide made up of alternating β-(1 → 4)-D-glucuronic acid and β-(1 → 3)-N-acetyl-D-glucosamine residues that is found in cartilage, synovial fluid, and skin (Tchobanian et al., Citation2019). It is a kind of natural hydrophilic anion polymer, which can regulate the concentration of positive and negative ions around. Hyaluronan presents itself as a promising biomaterial in medical and cosmetic fields (Highley et al., Citation2016; Li et al., Citation2016;; Wickens et al., Citation2017). Furthermore, hyaluronic acid has multiple hydroxyl and amino groups on its surface, which can sequester growth factors and chemically modified by block polymers. More importantly, HA both has natural biocompatibility and biodegradability, which is also soluble in water, as well as showing fast absorption, short time of retention in tissues, fast degradation (Benedetti et al., Citation1993; Tan et al., Citation2009). In sum, hyaluronic acid has a promising prospect in the future for growth factors delivery.
Hyaluronic acid is an important component of extracellular matrix of vertebrates (Mercer et al., Citation2013). It has been reported that hyaluronic acid owning high molecular weight inhibits angiogenesis, while hyaluronic acid at low molecular weight stimulates endothelial cell proliferation and migration (Thönes et al., Citation2019). Because hyaluronic acid lacks sulfonic groups, it has a relative weak affinity for growth factors. The affinity of hyaluronic acid to growth factors can be improved by chemical modification, and the function of stabilizing growth factors and continuously controllable release can be realized. The modified HA achieves the functions of stabilizing growth factors and controllable release. For example, Zhou et al. (Citation2016) adopted a simple method for preparing silk fibroin (SF) and hyaluronic acid (HA) composite film. The composite film loaded with VEGF (vascular endothelial growth factor), and the experimental results showed that the modified composite membrane can accelerate the release of VEGF, exhibiting a huge potential for growth factor delivery. In addition, the results showed that lowering the temperature and adding HA led to the rapid release of VEGF (vascular endothelial growth factor) in the HA/SF membrane. Su et al. (Citation2014) developed a scaffold in the hope of replacing skin. The results showed that HA increased the adsorption of EGF and maintained the release of EGF from the scaffolds. Scanning tunneling microscope (STM) showed stenting was stable. Animal studies showed that mice treated with scaffolds containing EGF and HA healed well, and wounds treated with scaffolds containing both HA and EGF formed skin attachment 20 days after surgery. Thones et al. (2019) indicated that sulfated HA in hydrogel loaded with HB-EGF growth factor could induce the continuous release of HB-EGF for wound healing. HB-EGF is a growth factor that supports wound repair by activating epidermal keratinocytes and dermal fibroblasts. The experimental results showed that hyaluronic acid/collagen hydrogel containing sulfureted hyaluronic acid could significantly improve the efficacy of HB-EGF hydrogel. Functionalized hydrogel containing sulfur HA was a scaffold that effectively released growth factors ().
Figure 3. (a) Growth factors binding with GAGs (Hachim et al., Citation2019). (b) the chemical structure of hyaluronate-epidermal growth factor (HA-EGF) conjugates; the fluorescence graphs of the degradation behavior of EGF and HA-EGF conjugate in HA films after topical application of FITC-labelled samples on wounded tissues (Niu et al., Citation2019). (c) HB-EGF released from sHA1-AC-containing hydrogels promotes epidermal tip formation and the results were measured by Masson-Goldner Trichrome staining. Bars: 500 μm; 100 μm (insets). The combined tip lengths of both wound sites were shown (n = 3 with 3–4 sections per condition *p < .05, **p < .01, ***p < .005, ****p < .001) (Thönes et al., Citation2019).
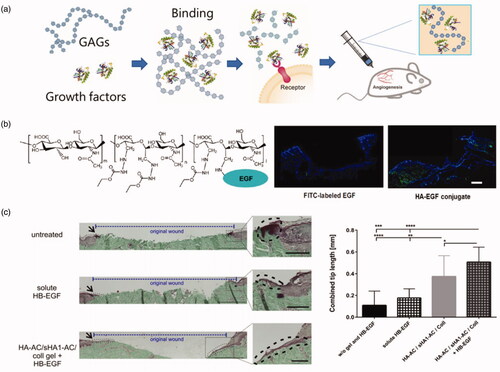
2.1.3. Heparin and heparin sulfate
Glycosaminoglycans (GAGs) represent a very promising group of components, which they could be functionally engineered and are well tolerated by the recipient tissues due to their relative immunological inertness (Dao et al., Citation2018). Both heparin and heparin sulfate are glycosaminoglycans (GAGs), which consist of repeated units of sulfonated hexatonic acid (1 → 4)-D-glucosamine (Hettiaratchi et al., Citation2017; Mohammadi et al., Citation2019). Heparin can be synthesized and stored in mast cells, while heparin sulfate, as a proteoglycan, is mainly present in the extracellular matrix of cells and tissues. Interestingly, heparin combines with many growth factors, which possesses a heparin-binding domain. This affinity-based binding ability slows down the diffusion of immobilized growth factors thus tempering the initial burst (Brasil et al., Citation2016). The heparin-based delivery systems can also prevent growth factors from enzymatic degradation thereby potentiating their biological functions (Choi et al., Citation2016). Heparin has a high affinity with various growth factors, which can regulate bioavailability, signal transduction and stability of growth factors. Heparin has the highest electronegative charge, which mediates its important biological roles as a multivalent binding agent for many proteins, including antithrombin III (to mediate thrombosis) and growth factors (to protect against degradation and potentiate receptor binding) (Nie et al., Citation2007). All these studies using covalently bound heparin were designed to enhance the biological properties of the hydrogel. Heparin-based delivery systems can also prevent enzymatic degradation of binding growth factors so that enhancing their biological functions. For example, levinson et al. (Caballero Aguilar et al., Citation2019) has previously described a system in which heparin was combined with hydrogel made by hyaluronic acid (HA) and transglutaminase (TG), the system showed the ability of controllable release of TGF-β (transforming growth factor). Another research developed a modified heparin impregnated with a light crosslinking alginate brine gel. After modified with heparin, the biodegradation properties of gel, swelling ratio and the modulus of elasticity were almost unchanged (Jeon et al., Citation2011). This controllable growth factor delivery system with independently controllable physical and cell adhesion properties provides a powerful therapeutic approach for a variety of therapeutic applications. Choi et al. (Citation2016) designed a system in which novel metal scaffold is fabricated by immobilizing heparin on the HA coated Co-Cr surface and loaded with vascular endothelial growth factor (VEGF) as well as hepatocyte growth factor (HGF). The results demonstrated that scaffolds modified with HA and heparin showed the capacity of rapid recovery of endothelial cells. Tang et al. (Citation2010) presented a system in which multi-functional delivery of basic fibroblast growth factor (bFGF) was incorporated with heparin-functionalized chitosan (CS)/poly(g-glutamic acid) (g-PGA) nanoparticles (HP-CS/g-PGA nanoparticles). With the increase of heparin content, the average particle size and bFGF loading efficiency are increasing. This delivery system may be a potential strategy for regeneration. Giulia et al. developed a novel 3 D scaffold platform consisting of a 3 D ring-shaped polycaprolactone (PCL) scaffold with heparinized surface to electrostatically bind vascular endothelial growth factor (VEGF) that can significantly promote vascularization. The scaffold demonstrated that heparin immobilization could be used for controlled delivery of VEGF and avoiding degradation (Marchioli et al., Citation2016) ().
Figure 4. (a) A schematic of showing the functionalized surface of PCL constructs binding VEGF. And the PCL and PCL/heparin plotted scaffolds stained with Azure II. (b) Graphs of neovascularization in PCL-VEGF and PCL-heparin-VEGF (Marchioli et al., Citation2016).
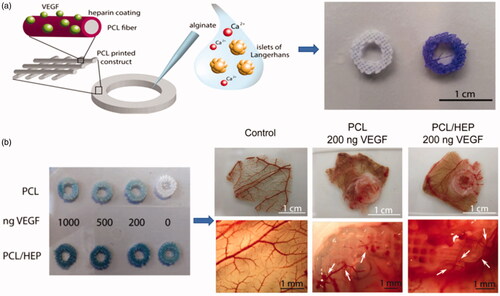
2.2. Microbial polysaccharide for controlling growth factors
Polysaccharides obtained from the microorganism are also one kind of the major polysaccharides existed in nature. Some of Microbial polysaccharides including the glycogen act as storage compound. Moreover, microbial polysaccharides show a potential prospect in medical, pharmaceutical and biomedical fields, which can be used as wound dressings, biomaterials, and tissue scaffolds.
2.2.1. Alginate
Alginate is a natural polysaccharide extracted from brown algae and is composed of various proportions of β-d-mannuronic acid (M) and α-l-guluronic acid (G) residues (Gu et al., Citation2004). Common alginate usually originates from algae and has a high degree of physicochemical heterogeneity, which can affect its quality and induce different applications (Mhanna et al., Citation2017). Alginate has many excellent properties, such as biocompatibility, low toxicity, low cost and has been widely studied in the field of biomedicine. Alginate gel induced by bivalent cation can be used for wound healing, therapeutic drugs, protein delivery and cell transplantation (Li et al., Citation2018). The main advantage of alginate is that stimulate the extracellular matrix and create a moist environment so that reduces the risk of bacterial infection in wounds and accelerates wound healing. What’s more, alginate hydrogel has also been used in cell transplantation in tissue engineering (Reakasame & Boccaccini, Citation2018). It transports cells to specific sites and provides an artificial matrix for new blood vessels. Alginate gels can also be administrated orally or injected into the body and can also be used in pharmaceutical fields (Kalaf et al., Citation2016; Yan et al., Citation2016).
Vascular endothelial growth factor (VEGF) is a potent pro-angiogenic signal transduction molecule (Jha et al., Citation2017; Roskoski, Citation2017). At an appropriate dosage, VEGF can up-regulate angiogenesis by signaling endothelial cells to undergo proliferation, migration and differentiation into new blood vessels (Gu et al., Citation2004). For example, Choi et al. (Citation2010) designed a system in which microcapsules made by PLGA and alginate were used for double-layer growth factor delivery, the bioactive growth factors were encapsulated in microcapsules. The experimental results showed that the system controlled the release of growth factors. Another example from Gu et al. (Citation2004) claimed that VEGF was encapsulated in calcium alginate beads using the extrusion/external gelation method, and was subsequently released in PBS and in serum media. The results showed that they obtained controlled release of VEGF in vitro. Additionally, alginate also could be modified with different degrees of sulfation using SO3/pyridine and obtained the ability of binding with FGF-2. The degree of modification mattered, and polysaccharides with higher sulfation degrees exhibited a stronger affinity for FGF-2 (Mhanna et al., Citation2017) ().
Figure 5. (a) Growth factors binding with polysaccharides for cell proliferation; (b) A schematic of encapsulation of rat BMSCs in the alginate hydrogel, along with BMP-2/Dex-loaded microcapsules; Then the surface morphology of alginate scaffolds was presented using SEM and a microcapsule was exposed on the surface of the alginate construct (Choi et al., Citation2010).
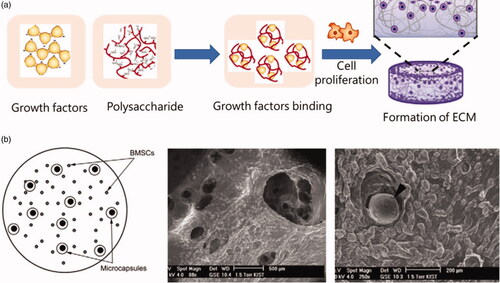
2.2.2. Dextran
Dextran is a high molecular-weight polysaccharide, consisted of α-1, 6 linking glucose of the backbone, α-1, 4 linking glucose of side chain. It can be extracted from different microbial strain owning different structures. It can be cross-linked for the separation and purification of proteins. Dextran possessing well biocompatibility can also be applied as the plasma expander in clinic. As for delivering growth factors, Zhang et al. (Citation2019) established a dextran/poly(lactic-co-glycolic acid)-combined microsphere system for sustained delivery of vascular endothelial growth factor us for therapeutic neovascularization. In this study, the VEGF-loaded dextran microparticles were prepared and then they were encapsulated into poly(lactic-co-glycolic acid) (PLGA) microspheres in order to obtain VEGF–dextran–PLGA microspheres. Finally, a novel growth factor sustained-release system based on the combination of protein-loaded dextran microparticles and PLGA microspheres was fabricated, and achieved a great progress in promoting mature vessel formation in a rat hind-limb ischemic model.
2.3. Botanical polysaccharides for controlling growth factors
Botanical polysaccharides comply with many requirements of pharmaceutical excipients such as nontoxicity, stability, availability and renewability, they are extensively investigated for use in solid oral dosage forms (Wang et al., Citation2017; Chen et al., Citation2018). Meanwhile, botanical polysaccharides have been used for industrial applications, e.g. pharmaceuticals, biomaterials, food stuff, nutrition, and biofuels (Jiao et al., Citation2016; Yin et al., Citation2019). Furthermore, polysaccharides with varying physicochemical properties can be extracted from plants at a relatively low cost and can be chemically modified to suit specific requirements (Beneke et al., Citation2009). Numerous researches indicate that polysaccharides can be used in biomedical science and have a diverse of therapeutic properties, such as antioxidant activity (Chen et al., Citation2019), immune activity (Miandare et al., Citation2017). It has been proved by experiments that adding active polysaccharides in feed can significantly improve the immune function of livestock and poultry. It can not only promote the development of immune organs, activate lymphocytes, but also promote the generation of antibodies and reduce animal mortality (Debele et al., Citation2016). Botanical polysaccharides have comprehensive physiological activity in human body and much studied indicated that polysaccharides can potently regulate the immune system. The main immune cell- macrophages can be activated by many botanical polysaccharides through specific membrane receptors. Based on this property, botanical polysaccharides have a promising prospect in tissue engineering. For instance, the Bletilla striata polysaccharide is obtained from B. striata, which composed of α-mannose, β-mannose and β-glucose at the mole ratio of 2.4:1. It can interact with macrophages and widely used in tissue regeneration. It was found that after the wound treated with BSP gel based on this polysaccharide, it was proved to control the inflammatory responses and accelerate the wound closure. Therefore, botanical polysaccharides are promising therapeutic natural materials, which can be widely used in biomedical science. But the studies of botanical polysaccharides on controlling growth factors are relative less and we summarize here.
2.3.1. Cellulose
Cellulose is the most abundant renewable polymer in nature, which is isolated from the secondary cell wall of plant cells, accounting for 20% ∼ 30% of the dry weight of plant cells (Rao et al., Citation2019). It plays a vital role in the field of functional foods. For instance, a variety of indigestible plant polysaccharides including cellulose, hemicelluloses, pectins, oligosaccharides, gums, were defined as the dietary fiber by the Food and Agriculture Organization (FAO). Among these, cellulose and hemicellulose can directly stimulate the bowel movement, which is the most widely spreading polymeric material in nature, is a fibrous, tough, water-insoluble material (Lynam et al., Citation2017; Xu et al., Citation2018). The basic unit of cellulose macromolecules is the glycan consisting of β-1, 4-glycoside bonds (Wang et al., Citation2016, Citation2018). Cellulose semi-synthetic derivatives are widely used in cosmetic and pharmaceutical fields, as well as used as dialysis membrane and biosensor (Amsden, Citation2015).
As for controlling growth factors, Roger et al. synthesized a delivery vehicle composed of an injectable hydrogel of hyaluronan (HA) and methyl cellulose (MC). Methyl cellulose was modified using thiol-maleimide and biotin–streptavidin chemistry to covalently bind the cell adhesive peptide, glycine–arginine–glycine–aspartic acid–serine (GRGDS), and the oligodendrocyte-differentiating factor, recombinant platelet-derived growth factor A (rPDGF-A). The experimental results indicated that this scaffold could induce more NSPCs differentiating into oligodendrocytes, compared with control groups (Tam et al., Citation2012).
2.3.2. Polysaccharides from Chinese medicinal herbs—new prospects for controlling growth factors
Of these fractions in herbal medicines, polysaccharides have been identified as major active ingredients, responsible for various pharmacological activities. Although the detailed mechanism of these effects is under exploration, the immunostimulatory activities of many polysaccharides are confirmed. Botanical polysaccharides can interact with macrophage receptors on the surface and activate different signaling pathways. For example, it can enhance the phagocytic activity of macrophages and promote the secretion of cytokines (Pearman et al., Citation2019). In the past decades, many polysaccharides were obtained from Chinese medicinal herbs; they have received massive attention as promising biomaterials in tissue engineering because of their biocompatible, safe and biodegradable properties.
In terms of controlling growth factors, new discoveries have been found and developed. For instance, Feng et al. obtained a novel polysaccharide form Konjac glucomannan, possessing affinitive for macrophages, and this polysaccharide could stimulate macrophages to release growth factors. This polysaccharide was further modified with heparin, and then they fabricated an injectable hydrogel scaffold consisted of KGM polysaccharide and heparin. They tested the efficacy of this scaffold in promoting angiogenesis. The results indicated that this scaffold based on polysaccharides had a potent prospect in tissue engineering (Feng et al., Citation2017). Additionally, except for KGM polysaccharide, Li et al. obtained a series of functional polysaccharides from herbs, expecting that they could have outstanding properties of controlling growth factors. They purified a polysaccharide from Eucommia ulmoides, named EUP3, containing a certain percentage of galacturonic acid. Unlike polysaccharides from animals such as glycosaminoglycans, EUP3 polysaccharide had no significant affinity for VEGF and bFGF, but had a clear binding affinity for PDGF-BB. Furthermore, Li et al. changed EUP3 polysaccharide into a growth factor-affinitive scaffold using electrospinning technology. The results demonstrated that this engineered scaffold based on EUP3 polysaccharide could enhance angiogenesis and promote wound healing via binding PDGF-BB (Li et al., Citation2016, Citation2017). As we mentioned above, polysaccharides from the Chinese medicinal herbs are special and have a promising potential for the applications in tissue engineering. Compared with other herbal polysaccharides, they have better mechanical properties and biological activities. Moreover, the polysaccharides avoid the risk of immune response and other side effects. Therefore, these polysaccharides exhibit a promising prospect, which have specific properties, can be used for growth factor delivery in tissue engineering ().
Figure 6. (a) a polysaccharide (EUP3) derived from Eucommia ulmoides was characterized, and showed potent capacity for binding with PDGF-BB. The in vivo experiment indicated that EUP3 could significantly promote vessel formation via sequestering PDGF-BB, compared with other groups. (b) Schematic illustration of the mechanisms of the designed ECM-mimetic sponge (EGS) for wound healing (Li et al., Citation2017).
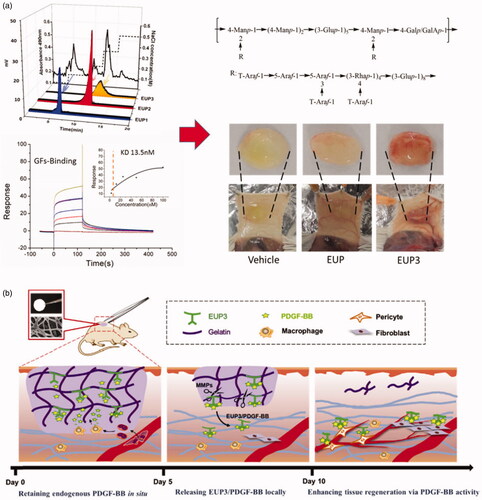
3. New biodegradable polymers in tissue engineering
Many new biodegradable polymers were expected to use for drug delivery, which were found to consistently induce ectopic bone formation when they combined with GFs and were implanted into the muscles of experimental animals (Saito & Takaoka, Citation2003). Given the challenge in growth factor delivery, the potential to accurately control the delivery process of growth factors is prospective. Nevertheless, it is a very challenging problem because multiple problems must be solved in order to develop the fine system (Sun et al., Citation2019). A vital requirement for a tissue engineering scaffold is that it degrades and resorbs at a suitable rate. New degradable polymers have many advantages as scaffold materials in tissue engineering. Among the advantages of polymers, the ability to tailor mechanical properties and degradation kinetics is very useful. The polymers are also attractive because they can be changed into various delivery systems with the required morphologic features. Furthermore, the polymers can also be modified with chemical functional groups which can induce tissue regeneration. There are many biodegradable synthetic polymers such as poly (glycolic acid), poly (p-dioxanone), and so on, which have been widely used in clinic. This chapter describes the effects of nanoparticles, hydrogels, and layer-by-layer film assembly systems based on biodegradable polymers on growth factors delivery ().
Figure 7. Nanoparticles (Saito & Takaoka, Citation2003), Scaffolds, Microspheres (Dinoro et al., Citation2019), Hydrogels (Hachim et al., Citation2019) based on biodegradable materials for delivering growth factors.
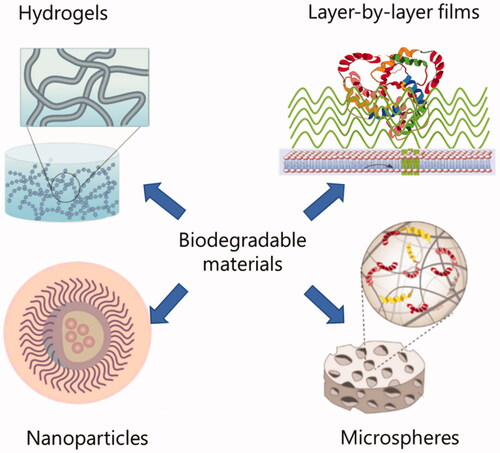
3.1. Nanoparticles for controlling growth factors
Nanotechnology has had a significant impact on a variety of therapeutics for decades. Advances in materials and formulation have produced safer and more efficient delivery of a myriad of drugs (Barclay et al., Citation2019). Targeted delivery strategies ensure a specific action and reduced systemic side effects. The flexibility and specificity of nanoparticle-based delivery systems provide an opportunity for targeting the immune system to initiate potent immune response.
The neuron growth factor (NGF) has the effect of restoring nerve damage and can regenerate the nerve. However, the effective delivery of drugs through the blood-brain barrier is still a major challenge. Lu et al. have invented an effective delivery system that enables NGF delivery to pass through the blood-brain barrier smoothly. The study selected a novel nanocarrier to encapsulate nerve growth factors and release in the nervous center system. In detail, this nanocapsule selected 2-methacryl phosphamidoline (MPC) as a monomer and polylactic acid (PLA) diacrylate as a cross-linking agent, which gathered around the NGF molecules through electrostatic interaction, and further transferred NGFs into a thin layer, which enclosed NGFs into the capsule. Due to the similarity of MPC with choline and acetylcholine, the capsule can be intravenously administered and recognized by choline transport protein and niacin acetylcholine receptor (nAChRs). Then the encapsulated neurotrophic factors can be released through the blood-brain barrier. This delivery system can effectively prolong the half-life in blood circulation without causing systemic reactions, and continuously release NGFs in damaged areas of the nervous system and reduce the occurrence of adverse reactions. Rajam et al. (Citation2011) conducted a novel strategy, which prepared chitosan nanoparticles (CNP) and combined them with growth factors including epidermal growth factor (EGF) and fibroblast growth factor (FGF). The experimental results indicated that chitosan nanoparticles can be used as a dual growth factor delivery system in tissue engineering.
3.2. Hydrogels and microspheres for controlling growth factors
Hydrogel and microspheres are vital platforms for growth factor delivery, which usually have mechanical properties like those of many tissues, so they do not cause a strong inflammatory response through mechanical stimulation. In addition, the property of high-water content provides a means of releasing large growth factor molecules by diffusion in the water region between the polymer chains. The structure and mechanical properties of hydrogels can be regulated by a variety of different chemical methods. They can be made into injectable forms, such as nanoparticles, thixotropic systems or in-situ chemical or physical cross-linked polymers, so they are easily implanted into tissues through minimally invasive means (Amsden, Citation2015). It has been discovered that growth factors released from various hydrogel formulations have been designed, which binding growth factors with the hydrogel polymer network. Kobayashi et al. (Citation2017) indicated that hydrogels could be explored for targeting delivery and controlled release of bFGF. The experiment results demonstrated that hydrogels have a better effect than solution injection alone. Rensburg et al. reported that hydrogels covalently modified by heparin could continue to deliver active growth factors, which also acted as a matrix significantly increasing angiogenesis in vivo (van Rensburg et al., Citation2017). Similarly, Jason et al. showed that degradable PEG hydrogel significantly influenced osteoblast differentiation by transmitting growth factors for osteoinduction. The results of all the study have demonstrated the potential of hydrogels to deliver growth factors, providing a reference for growth factor delivery strategies with endless potential (Rajam et al., Citation2011). Perez et al. (Citation2014) developed novel microcarriers made of sol–gel-derived bioactive glasses for delivering growth factors for cultivating stem cells in bone tissue engineering. Basic fibroblast growth factor (bFGF) was incorporated into the microcarriers and showed a sustained release pattern, which promoted cell adhesion and proliferation on the bFGF-loaded microcarriers. It is indicated that the engineered mesoporous bioactive glass microspheres represent a new class of growth factor delivery carrier, potentially useful in tissue engineering.
3.3. Layer-by-layer films for controlling growth factors
In general, biomedical delivery systems require good mechanical functions to locally release growth factors to promote tissue response, and the perfect systems are deemed to achieve these aspects simultaneously with a single material. One way to fulfill it is to select a material with appropriate mechanical properties and then use a layer-by-layer assembly method to coat the surface with a film containing growth factors. The laminar assembly of the film on different substrate surfaces is based on alternating electrostatic adsorption of polyelectrolytes with opposite charges in aqueous solution (Amsden, Citation2015). Since growth factors have a net charge in an aqueous solution, they are easily incorporated into the film. The incorporation of the membranes depends on the isoelectric point of the growth factor and the pKa value of the polyelectrolyte. The release of growth factors from these components is a complex process that is influenced by the strength of the growth factors bound to the polyelectrolyte, the diffusivity of growth factors in the polyelectrolyte layer, and the degradation rate of the polyelectrolyte. This method has many advantages including basing on the water and no use of potentially toxic organic solvent in the assembly of the film. Certainly, this approach also has disadvantages. To achieve continuous release and minimal fracture effect, a large number of layers are usually required, which requires a lengthy preparation process. Kaminski et. al studied polymer-coated liposomes to deliver growth factors. Three-dimensional layer-by-layer structures of xanthan gum and galactans on liposome templates of dimethyl bromide (DODAB) have been studied and characterized. The experimental results showed that double-coated nanoparticles increase the continuous release of epithelial growth (EGF) by up to five times (Kaminski et al., Citation2016).
4. Conclusions
At present, precious growth factor delivery has exhibited a potential prospect in tissue engineering. With the tremendous development of novel biomaterials, it will increase our capacity to recapitulate tissue repair and regeneration through the precious delivery of various growth factors. Among these, the most commonly used biomaterials are natural polysaccharides and its analogues, which can be fabricated into scaffolds through versatile technologies. Polysaccharides derived from animals and herbs are natural biomaterials which are easily obtained. Among theses, a series of polysaccharides have been explored into functional biomaterial scaffolds for delivering growth factors. However, although a lot of polysaccharides were acquired and used as various biomaterial scaffolds in tissue engineering, the mechanism of polysaccharides interacting with bodies was still obscure due to the complicated structures. The detailed mechanisms and relationship between structure and activity should be observed further. Moreover, how the scaffolds made by polysaccharides exert functions is still a challenge. Acquisition of high purity and clear structure of polysaccharides is also difficult. Additionally, as for the application of functional polysaccharides in tissue engineering, although lots of researches have been performed for exploiting the functional scaffolds, there is still a long way to transform from research to clinic. In addition, there are still many limitations including the immunogenicity of scaffolds, low stability and high cost. In the future, we should develop the polysaccharide scaffolds with the requirements of clear structure, solid bioactivities, biocompatibility and security. Besides, the use of nanomaterials for delivering growth factors in tissue engineering is also extensively existing and multiple materials showed many advantages, where the materials can provide controlled and precise growth factors delivery. Although the same problem of transformation from research to clinic is existing, further development of scaffolds based on nanomaterials could be a worthy try. Meanwhile, many other fields could readily obtain a benefit from the utilization of biomaterials as carriers for delivering growth factors to the target tissues in vitro and in vivo.
Disclosure statement
The authors declare that there is no conflict of interest.
Additional information
Funding
References
- Ahsan SM, Thomas M, Reddy KK, et al. (2018). Chitosan as biomaterial in drug delivery and tissue engineering. Int J Biol Macromol 110:97–109.
- Aloe L, Rocco ML, Balzamino BO, Micera A. (2016). Nerve growth factor: role in growth, differentiation and controlling cancer cell development. J Exp Clin Cancer Res 35:116.
- Amsden B. (2015). Novel biodegradable polymers for local growth factor delivery. Eur J Pharm Biopharm 97:318–28.
- Arisaka Y, Yui N. (2019). Engineering molecularly mobile polyrotaxane surfaces with heparin-binding EGF-like growth factors for improving hepatocyte functions. J Biomed Mater Res A 107:1080–5.
- Azizian S, Hadjizadeh A, Niknejad H. (2018). Chitosan-gelatin porous scaffold incorporated with Chitosan nanoparticles for growth factor delivery in tissue engineering. Carbohydr Polym 202:315–22.
- Benedetti L, Cortivo R, Berti T, et al. (1993). Biocompatibility and biodegradation of different hyaluronan derivatives (Hyaff) implanted in rats. Biomaterials 14:1154–60.
- Barclay TG, Day CM, Petrovsky N, Garg S. (2019). Review of polysaccharide particle-based functional drug delivery. Carbohydr Polym 221:94–112.
- Beneke CE, Viljoen AM, Hamman JH. (2009). Polymeric plant-derived excipients in drug delivery. Molecules 14:2602–20.
- Bhaskar U, Sterner E, Hickey AM, et al. (2012). Engineering of routes to heparin and related polysaccharides. Appl Microbiol Biotechnol 93:1–16.
- Brasil FH, Ferrer V, Navone NM, et al. (2016). Heparan sulfate modifying enzymes deliver cancer, stromal and macrophage-produced heparin-binding growth factors in the prostate cancer microenvironment. Can Res, 76:5102.
- Caballero Aguilar LM, Silva SM, Moulton SE. (2019). Growth factor delivery: defining the next generation platforms for tissue engineering. J Control Release 306:40–58.
- Catizone A, Ricci G, Del Bravo J, Galdieri M. (2006). Hepatocyte growth factor modulates in vitro survival and proliferation of germ cells during postnatal testis development. J Endocrinol 189:137–46.
- Chen Z, Cheng L, He Y, Wei X. (2018). Extraction, characterization, utilization as wound dressing and drug delivery of Bletilla striata polysaccharide: a review. Int J Biol Macromol 120:2076–85.
- Chen S, Huang H, Huang G. (2019). Extraction, derivatization and antioxidant activity of cucumber polysaccharide. Int J Biol Macromol 140:1047–53.
- Choi DH, Kang SN, Kim SM, et al. (2016). Growth factors-loaded stents modified with hyaluronic acid and heparin for induction of rapid and tight re-endothelialization. Colloids Surf B Biointerfaces 141:602–10.
- Choi DH, Park CH, Kim IH, et al. (2010). Fabrication of core-shell microcapsules using PLGA and alginate for dual growth factor delivery system. J Control Release 147:193–201.
- Chu X, Chen C, Chen C, et al. (2019). Evidence for lung repair and regeneration in humans: key stem cells and therapeutic functions of fibroblast growth factors. Front Med 14:262–272 .
- Cipitria A, Salmeron-Sanchez M. (2017). Mechanotransduction and growth factor signalling to engineer cellular microenvironments. Adv Healthcare Mater 6:1700052.
- Dao DT, Anez-Bustillos L, Adam RM, et al. (2018). Heparin-binding epidermal growth factor-like growth factor as a critical mediator of tissue repair and regeneration. Am J Pathol 188:2446–56.
- Davydova N, Harris NC, Roufail S, et al. (2016). Differential receptor binding and regulatory mechanisms for the lymphangiogenic growth factors vascular endothelial growth factor (VEGF)-C and -D. J Biol Chem 291:27265–78.
- DeAngelis PL. (2012). Glycosaminoglycan polysaccharide biosynthesis and production: today and tomorrow. Appl Microbiol Biotechnol 94:295–305.
- Debele TA, Mekuria SL, Tsai H-C. (2016). Polysaccharide based nanogels in the drug delivery system: application as the carrier of pharmaceutical agents. Mater Sci Eng C Mater Biol Appl 68:964–81.
- Degirolamo C, Sabbà C, Moschetta A. (2016). Therapeutic potential of the endocrine fibroblast growth factors FGF19, FGF21 and FGF23. Nat Rev Drug Discov 15:51–69.
- Dinoro J, Maher M, Talebian S, et al. (2019). Sulfated polysaccharide-based scaffolds for orthopaedic tissue engineering. Biomaterials 214:119214
- Duncan HF, Kobayashi Y, Shimizu E. (2018). Growth factors and cell homing in dental tissue regeneration. Curr Oral Health Rep 5:276–85.
- Erdogan B, Webb DJ. (2017). Cancer-associated fibroblasts modulate growth factor signaling and extracellular matrix remodeling to regulate tumor metastasis. Biochem Soc Trans 45:229–36.
- Evans BC, Fletcher RB, Kilchrist KV, et al. (2019). An anionic, endosome-escaping polymer to potentiate intracellular delivery of cationic peptides, biomacromolecules, and nanoparticles. Nat Commun 10:1–19.,
- Feng Y, Li Q, Wu D, et al. (2017). A macrophage-activating, injectable hydrogel to sequester endogenous growth factors for in situ angiogenesis. Biomaterials 134:128–42.,
- Figueroa V, Rodríguez MS, Lanari C, Lamb CA. (2019). Nuclear action of FGF members in endocrine-related tissues and cancer: interplay with steroid receptor pathways. Steroids 152:108492
- Gallego-Muñoz P, Ibares-Frías L, Valsero-Blanco MC, et al. (2017). Effects of TGFβ1, PDGF-BB, and bFGF, on human corneal fibroblasts proliferation and differentiation during stromal repair. Cytokine 96:94–101.,
- Goh M, Hwang Y, Tae G. (2016). Epidermal growth factor loaded heparin-based hydrogel sheet for skin wound healing. Carbohydr Polym 147:251–60.
- Gohil S, Padmanabhan A, Deschamps J, Nair L. (2017). Chitosan-based scaffolds for growth factor delivery. In: Chitosan Based Biomaterials Volume 2. Woodhead Publishing: Elsevier, 175–207.
- Gu F, Amsden B, Neufeld R. (2004). Sustained delivery of vascular endothelial growth factor with alginate beads. J Control Release 96:463–72.
- Guo JL, Kim YS, Mikos AG. (2019). Biomacromolecules for tissue engineering: emerging biomimetic strategies. Biomacromolecules 20:2904–12.
- Hachim D, Whittaker TE, Kim H, Stevens MM. (2019). Glycosaminoglycan-based biomaterials for growth factor and cytokine delivery: making the right choices. J Control Release 313:131–47.
- Hellberg C, Östman A. Heldin C-H. (2010). PDGF and vessel maturation, Angiogenesis inhibition. Berlin, Heidelberg: Springer, 103–114.
- Hendrikse SI, Spaans S, Meijer E, Dankers PY. (2018). Supramolecular platform stabilizing growth factors. Biomacromolecules 19:2610–7.
- Hettiaratchi MH, Chou C, Servies N, et al. (2017). Competitive protein binding influences heparin-based modulation of spatial growth factor delivery for bone regeneration. Tissue Eng Part A 23:683–95.
- Highley CB, Prestwich GD, Burdick JA. (2016). Recent advances in hyaluronic acid hydrogels for biomedical applications. Curr Opin Biotechnol 40:35–40.
- Hinz B. (2015). The extracellular matrix and transforming growth factor-β1: tale of a strained relationship. Matrix Biol 47:54–65.
- Ishihara J, Ishihara A, Fukunaga K, et al. (2018). Laminin heparin-binding peptides bind to several growth factors and enhance diabetic wound healing. Nat Commun 9:1–14.
- Islam S, Bhuiyan MR, Islam M. (2017). Chitin and chitosan: structure, properties and applications in biomedical engineering. J Polym Environ 25:854–66.
- Jeon O, Powell C, Solorio LD, et al. (2011). Affinity-based growth factor delivery using biodegradable, photocrosslinked heparin-alginate hydrogels. J Control Release 154:258–66.
- Jha SK, Rauniyar K, Karpanen T, et al. (2017). Efficient activation of the lymphangiogenic growth factor VEGF-C requires the C-terminal domain of VEGF-C and the N-terminal domain of CCBE1. Sci Rep 7:1–13.
- Jia J, Ye T, Cui P, et al. (2016). AP-1 transcription factor mediates VEGF-induced endothelial cell migration and proliferation. Microvasc Res 105:103–8.
- Jiao R, Liu Y, Gao H, et al. (2016). The anti-oxidant and antitumor properties of plant polysaccharides. Am J Chin Med 44:463–88.
- Kalaf EAG, Flores R, Bledsoe JG, Sell SA. (2016). Characterization of slow-gelling alginate hydrogels for intervertebral disc tissue-engineering applications. Mater Sci Eng C Mater Biol Appl 63:198–210.
- Kaminski GA, Sierakowski MR, Pontarolo R, et al. (2016). Layer-by-layer polysaccharide-coated liposomes for sustained delivery of epidermal growth factor. Carbohydr Polym 140:129–35.
- Kobayashi T, Mizuta M, Hiwatashi N, et al. (2017). Drug delivery system of basic fibroblast growth factor using gelatin hydrogel for restoration of acute vocal fold scar. Auris Nasus Larynx 44:86–92.
- Kumar A, Rao KM, Han SS. (2018). Application of xanthan gum as polysaccharide in tissue engineering: a review. Carbohydr Polym 180:128–44.
- Le Dour C, Wu W, Béréziat V, et al. (2017). Extracellular matrix remodeling and transforming growth factor-β signaling abnormalities induced by lamin A/C variants that cause lipodystrophy. J Lipid Res 58:151–63.
- Li Q, Guo G, Meng F, et al. (2016). A naturally derived, growth factor-binding polysaccharide for therapeutic angiogenesis. ACS Macro Lett 5:617–21.
- Li Q, Niu Y, Diao H, et al. (2017). In situ sequestration of endogenous PDGF-BB with an ECM-mimetic sponge for accelerated wound healing. Biomaterials 148:54–68.
- Li Q, Niu Y, Xing P, Wang C. (2018). Bioactive polysaccharides from natural resources including Chinese medicinal herbs on tissue repair. Chin Med 13:7.
- Liu M, Zheng H, Chen J, et al. (2016). Chitosan-chitin nanocrystal composite scaffolds for tissue engineering. Carbohydr Polym 152:832–40.
- Li G, Xiao Q, Zhang L, et al. (2017). Nerve growth factor loaded heparin/chitosan scaffolds for accelerating peripheral nerve regeneration. Carbohydr Polym 171:39–49.
- Li W, Yi X, Liu X, et al. (2016). Hyaluronic acid ion-pairing nanoparticles for targeted tumor therapy. J Control Release 225:170–82.
- Lynam JG, Kumar N, Wong MJ. (2017). Deep eutectic solvents' ability to solubilize lignin, cellulose, and hemicellulose; thermal stability; and density. Bioresour Technol 238:684–9.
- Mancarella C, Calzolari L, Ferrari S, et al. (2016). Insulin-like growth factor 2 (IGF-2) mRNA binding protein 3 predicts poor prognosis and promotes cell proliferation in Ewing sarcoma. Clin Res, 76(14):3201.
- Mangla B, Joshi SK, Khanna K, et al. (2017). Hyaluronic acid: the ingenious molecule. Biopharm J 1:64–70.
- Marchioli G, Luca AD, de Koning E, et al. (2016). Hybrid polycaprolactone/alginate scaffolds functionalized with VEGF to promote de novo vessel formation for the transplantation of islets of Langerhans. Adv Healthc Mater 5:1606–16.
- Mercer SE, Odelberg SJ, Simon H-G. (2013). A dynamic spatiotemporal extracellular matrix facilitates epicardial-mediated vertebrate heart regeneration. Dev Biol 382:457–69.
- Mhanna R, Becher J, Schnabelrauch M, et al. (2017). Sulfated alginate as a mimic of sulfated glycosaminoglycans: binding of growth factors and effect on stem cell behavior. Adv Biosys 1:1700043.
- Miandare HK, Mirghaed AT, Hosseini M, et al. (2017). Dietary Immunogen((R)) modulated digestive enzyme activity and immune gene expression in Litopenaeus vannamei post larvae. Fish Shellfish Immunol 70:621–7.
- Miao T, Wang J, Zeng Y, et al. (2018). Polysaccharide-based controlled release systems for therapeutics delivery and tissue engineering: from bench to bedside. Adv Sci 5:1700513.
- Millette E, Rauch BH, Kenagy RD, et al. (2006). Platelet-derived growth factor-BB transactivates the fibroblast growth factor receptor to induce proliferation in human smooth muscle cells. Trends Cardiovasc Med 16:25–8.
- Mohammadi S, Ramakrishna S, Laurent S, et al. (2019). Fabrication of nanofibrous PVA/alginate-sulfate substrates for growth factor delivery. J Biomed Mater Res A 107:403–13.
- Necas J, Bartosikova L, Brauner P, Kolar J. (2008). Hyaluronic acid (hyaluronan): a review. Vet Med 53:397–411.
- Nicoletti F, Mazzon E, Fagone P, et al. (2019). Prevention of clinical and histological signs of MOG-induced experimental allergic encephalomyelitis by prolonged treatment with recombinant human EGF. J Neuroimmunol 332:224–32.
- Nie T, Baldwin A, Yamaguchi N, Kiick KL. (2007). Production of heparin-functionalized hydrogels for the development of responsive and controlled growth factor delivery systems. J Control Release 122:287–96.
- Niu Y, Li Q, Ding Y, et al. (2019). Engineered delivery strategies for enhanced control of growth factor activities in wound healing. Adv Drug Deliv Rev 146:190–208.
- Oh J-S, Lee E-J. (2019). Engineered dressing of hybrid chitosan-silica for effective delivery of keratin growth factor and acceleration of wound healing. Mater Sci Eng C Mater Biol Appl 103:109815.
- Onodera Y, Teramura T, Takehara T, Fukuda K. (2019). Transforming growth factor β-activated kinase 1 regulates mesenchymal stem cell proliferation through stabilization of Yap1/Taz proteins. Stem Cells 37:1595–605.
- Pangon A, Saesoo S, Saengkrit N, et al. (2016). Hydroxyapatite-hybridized chitosan/chitin whisker bionanocomposite fibers for bone tissue engineering applications. Carbohydr Polym 144:419–27.
- Pearman N, Moxon S, Carnachan SM, et al. (2019). Investigating potential wound healing properties of polysaccharides extracted from Grewia mollis Juss. and Hoheria populnea A. Cunn.(Malvaceae). Bioact Carbohydr Diet Fibre 20:100201.
- Perez RA, El-Fiqi A, Park JH, et al. (2014). Therapeutic bioactive microcarriers: co-delivery of growth factors and stem cells for bone tissue engineering. Acta Biomater 10:520–30.
- Qian Y, Han Q, Chen W, et al. (2017). Platelet-rich plasma derived growth factors contribute to stem cell differentiation in musculoskeletal regeneration. Front Chem 5:89.
- Rajam M, Pulavendran S, Rose C, Mandal A. (2011). Chitosan nanoparticles as a dual growth factor delivery system for tissue engineering applications. Int J Pharm 410:145–52.
- Rao SS, Rekha P, Anil S, et al. (2019). Natural polysaccharides for growth factors delivery. In: Natural polysaccharides in drug delivery and biomedical applications. Academic Press: Elsevier, 495–512.
- Reakasame S, Boccaccini AR. (2018). Oxidized alginate-based hydrogels for tissue engineering applications: a review. Biomacromolecules 19:3–21.
- Roskoski R. Jr, (2017). Vascular endothelial growth factor (VEGF) and VEGF receptor inhibitors in the treatment of renal cell carcinomas. Pharmacol Res 120:116–32.
- Saito N, Takaoka K. (2003). New synthetic biodegradable polymers as BMP carriers for bone tissue engineering. Biomaterials 24:2287–93.
- Singh G, Manohar M, Arya SK, et al. (2017). Potential biomedical applications of chitosan–and chitosan-based nanomaterials. Chitosan Deriv Compos Appl 14:385–408.
- Shelke NB, James R, Laurencin CT, Kumbar SG. (2014). Polysaccharide biomaterials for drug delivery and regenerative engineering. Polym Adv Technol 25:448–60.
- Smirnova NV, Kolbe KA, Dresvyanina EN, et al. (2019). Effect of chitin nanofibrils on biocompatibility and bioactivity of the chitosan-based composite film matrix intended for tissue engineering. Materials 12:1874.
- Su Z, Ma H, Wu Z, et al. (2014). Enhancement of skin wound healing with decellularized scaffolds loaded with hyaluronic acid and epidermal growth factor. Mater Sci Eng C Mater Biol Appl 44:440–8.
- Sun S, Yau A, Chen Y. (2019). Biomedical applications and biomaterial delivery strategies of growth factors. Biomater Transl Med 11:257–68.
- Surmacz E. (2003). Growth factor receptors as therapeutic targets: strategies to inhibit the insulin-like growth factor I receptor. Oncogene 22:6589–97.
- Tam RY, Cooke MJ, Shoichet MSJJoMC. (2012). A covalently modified hydrogel blend of hyaluronan–methyl cellulose with peptides and growth factors influences neural stem/progenitor cell fate. J Mater Chem 22:19402–11.
- Tan H, Chu CR, Payne KA, Marra KG. (2009). Injectable in situ forming biodegradable chitosan-hyaluronic acid based hydrogels for cartilage tissue engineering. Biomaterials 30:2499–506.
- Tang D-W, Yu S-H, Ho Y-C, et al. (2010). Heparinized chitosan/poly(γ-glutamic acid) nanoparticles for multi-functional delivery of fibroblast growth factor and heparin. Biomaterials 31:9320–32.
- Tchobanian A, Van Oosterwyck H, Fardim P. (2019). Polysaccharides for tissue engineering: current landscape and future prospects. Carbohydr Polym 205:601–25.
- Thönes S, Rother S, Wippold T, et al. (2019). Hyaluronan/collagen hydrogels containing sulfated hyaluronan improve wound healing by sustained release of heparin-binding EGF-like growth factor. Acta Biomater 86:135–47.
- Tiwari S, Patil R, Bahadur P. (2018). Polysaccharide based scaffolds for soft tissue engineering applications. Polymers 11:1.
- Uebersax L, Merkle HP, Meinel L. (2009). Biopolymer-based growth factor delivery for tissue repair: from natural concepts to engineered systems. Tissue Eng Part B Rev 15:263–89.
- van Rensburg AJ, Davies NH, Oosthuysen A, et al. (2017). Improved vascularization of porous scaffolds through growth factor delivery from heparinized polyethylene glycol hydrogels. Acta Biomater 49:89–100.
- Vanderwerff BR, Church KJ, Kawas LH, Harding JW. (2019). Comparative characterization of the HGF/Met and MSP/Ron systems in primary pancreatic adenocarcinoma. Cytokine 123:154762.
- Vandghanooni S, Eskandani M. (2019). Electrically conductive biomaterials based on natural polysaccharides: challenges and applications in tissue engineering. Int J Biol Macromol 141:636–62.
- Venkatesan J, Anil S, Kim S-K, Shim MS. (2017). Chitosan as a vehicle for growth factor delivery: various preparations and their applications in bone tissue regeneration. Int J Biol Macromol 104:1383–97.
- Venkatesan J, Kim S-K, Wong TW. (2015). Chitosan and its application as tissue engineering scaffolds. In Nanotechnology applications for tissue engineering. William Andrew Publishing: Elsevier, 133–47.
- Wang X, Ding J, Feng Y, et al. (2017). Targeting of growth factors in the treatment of hepatocellular carcinoma: the potentials of polysaccharides. Oncol Lett 13:1509–17.
- Wang B, Lv X, Chen S, et al. (2018). Use of heparinized bacterial cellulose based scaffold for improving angiogenesis in tissue regeneration. Carbohydr Polym 181:948–56.
- Wang K, Nune K, Misra R. (2016). The functional response of alginate-gelatin-nanocrystalline cellulose injectable hydrogels toward delivery of cells and bioactive molecules. Acta Biomater 36:143–51.
- Wang Z, Wang Z, Lu WW, et al. (2017). Novel biomaterial strategies for controlled growth factor delivery for biomedical applications. NPG Asia Mater 9:e435–e435.
- Wickens JM, Alsaab HO, Kesharwani P, et al. (2017). Recent advances in hyaluronic acid-decorated nanocarriers for targeted cancer therapy. Drug Discov Today 22:665–80.
- Xu Y, Ding H, Luo C, et al. (2018). Effect of lignin, cellulose and hemicellulose on calcium looping behavior of CaO-based sorbents derived from extrusion-spherization method. Chem Eng J. 334:2520–2529.
- Yan HJ, Casalini T, Hulsart-Billström G, et al. (2018). Synthetic design of growth factor sequestering extracellular matrix mimetic hydrogel for promoting in vivo bone formation. Biomaterials 161:190–202.
- Yan J, Miao Y, Tan H, et al. (2016). Injectable alginate/hydroxyapatite gel scaffold combined with gelatin microspheres for drug delivery and bone tissue engineering. Mater Sci Eng C Mater Biol Appl 63:274–84.
- Yilgor P, Tuzlakoglu K, Reis RL, et al. (2009). Incorporation of a sequential BMP-2/BMP-7 delivery system into chitosan-based scaffolds for bone tissue engineering. Biomaterials 30:3551–9.
- Yin M, Zhang Y, Li H. (2019). Advances in research on immunoregulation of macrophages by plant polysaccharides. Front. Immunol 10:145.
- Zhang Y, Sun T, Jiang C. (2018). Biomacromolecules as carriers in drug delivery and tissue engineering. Acta Pharmaceutica Sinica B 8:34–50.
- Zhang ZD, Xu YQ, Chen F, et al. (2019). Sustained delivery of vascular endothelial growth factor using a dextran/poly(lactic-co-glycolic acid)-combined microsphere system for therapeutic neovascularization. Heart Vessels 34:167–76.
- Zheng Y, Wu J, Shan W, et al. (2018). Multifunctional nanoparticles enable efficient oral delivery of biomacromolecules via improving payload stability and regulating the transcytosis pathway. ACS Appl Mater Interfaces 10:34039–49.
- Zhou J, Zhang B, Liu X, et al. (2016). Facile method to prepare silk fibroin/hyaluronic acid films for vascular endothelial growth factor release. Carbohydr Polym 143:301–9.
- Zubillaga V, Alonso-Varona A, Fernandes S, et al. (2020). Adipose-derived mesenchymal stem cell chondrospheroids cultured in hypoxia and a 3D porous chitosan/chitin nanocrystal scaffold as a platform for cartilage tissue engineering. IJMS 21:1004.