ABSTRACT
Polyphenoloxidase (PPO) from prawns (Penaeus vannamei) was purified by ammonium sulphate precipitation, ion-exchange column, and gel filtration chromatography. The specific activity of PPO increased by 9.51 fold to 575.03 U/mg, and the molecular weight of PPO was 66 kDa. The optimal temperature and pH were around 45°C and 6.8, determined by L-β-(3,4-dihydroxylphenyl) alanine (L-DOPA) as substrate. Km and Vmax values were found to be 2.5 mM and 0.062ΔA/min. The effect of thermal treatment on the activity, conformation, and microstructure of the PPO was investigated. Thermal stability of PPO was measured at 40°C, 50°C, 60°C, 70°C, and 80°C, and the half-life values of PPO were 105, 39.6, 13.4, 8.9, and 3.1 min, respectively. PPO activity slightly decreased at 60°C, while the relative enzyme activity remained 9.4% after 80°C for 10 min. The result of differential scanning calorimetry showed that the denatured temperature of PPO was 65°C. Thermal treatment resulted in the changes in the tertiary structure of PPO, and the λmax of fluorescence spectra was red-shifted with decreasing intensity. Curve-fitting analysis showed that α-helix and β-sheet decreased, but β-turns and random coil increased with increased temperature. Atomic force microscopy revealed that PPO molecules aggregated remarkably at 60°C. Moreover, the formation of large globular aggregates occurred as the temperature increased.
Introduction
Prawns are eaten regularly in Asia and are also one of the most important aquatic products consumed in many countries all over the world due to their delicacy and nutritional values.[Citation1] Shrimp are highly perishable with short shelf-life, mainly caused by melanosis (black spots or other discolouration) and microbial spoilage.[Citation2] Although melanosis seems harmless to consumers, it drastically reduces the product’s commercial value by negatively impacting its acceptability to consumers and, hence, causes considerable money loss.[Citation3] Most researchers found that melanosis was triggered by a special enzyme, which could oxidise phenols to quinines.[Citation4] The quinones are highly reactive organic compounds involved in various reaction pathways. They are strong electrophiles which may suffer nucleophilic attacked by other polyphenols, amino acids, or proteins to produce a dark-brown or black pigment.[Citation5] This browning reaction does not stop until all enzymes are inactivated.[Citation6] Such oxidation is mainly caused by polyphenoloxidase (PPO; EC 1.14.18.1), and thus, many studies have focused on inhibiting the activity of PPO during food processing in order to prevent browning.[Citation7,Citation8]
A lot of methods have been used to the inhibition of PPO from Pacific white shrimp. Basiri found that pomegranate peels extracts could inhibit the PPO of Pacific white shrimp during iced storage.[Citation9] Catechin and ferulic acid showed strong inhibitory effect on the PPO from cephalothorax of Pacific white shrimp.[Citation10] Manheem reported that PPO of Pacific white shrimp was quite stable when heated at temperature lower than 80°C, and the activities of enzymes decreased with increasing pre-cooking time.[Citation11] Thermal treatment is the most widely used technique in the food industry for inactivation of quality-reducing enzymes. Ndiaye found that PPO in mango slices was inhibited by steam.[Citation12] Schweiggert reported that PPO of chili powder was completely inactivated by heating at 80°C for 10 min.[Citation13] Some researchers have studied the inactivation of PPO under thermal treatment [Citation14,Citation15], and most of them mainly focused on simple kinetic approaches. However, there is little work about the purification of PPO from Pacific white shrimp and the changes about the conformation and microstructural changes of PPO under thermal treatment. Spectroscopy is an effective way to study the conformation of the protein and its microscopic characteristics.[Citation16] In this study, PPO from Penaeus vannamei was purified and characterised in terms of optimum pH and temperature. Thermal stability of the enzyme, along with the secondary and tertiary structural characteristics induced by thermal inactivation, was determined in an effort to explore the mechanism of thermal inactivation of PPO from Penaeus vannamei.
Materials and methods
Chemicals
L-β-(3,4-dihydroxylphenyl) alanine (L-DOPA), Brij-35, bovine serum albumin (BSA), Hydroxymethyl aminomethane (Tris), glycine, sodium dodecyl sulphate (SDS), N,N,N’,N’-tetramethyl-ethylenediamine (TEMED), acrylamide, methylene bis-acrylamide, and ammonium persulphate were purchased from Sigma Chemical Co. (St. Louis, MO, USA). Sephadex G-100 and DEAE Sepharose Fast Flow column materials were purchased from GE Healthcare (Shanghai, China). Coomassie brilliant blue G-250 and Coomassie brilliant blue R-250 were purchased from Amresco (Beijing, China). All other chemicals used were of analytical grade.
Sample preparation
Prawns (Penaeus vannamei) with an average weight of 15 ± 2 g were purchased from the local seafood market (Jinzhou, China). The shrimp were transported to the laboratory in 0.5 h. The carapace was removed from the cephalothorax. Twenty cephalothoraxes for each sample were separated, frozen using liquid nitrogen, and ground to a fine powder. The powder was then vacuum-packed and stored at −80°C for enzyme extraction.
Extraction and purification of PPO enzyme
Extraction and purification of the enzyme were done at 4°C according to the method of Nirmal and Benjakul with slight modifications.[Citation10] Cephalothoraxes powder (100 g) was mixed with 400 mL of 0.067 M phosphate buffer (pH 7.2, containing 0.1% Brij-35). The mixture was stirred continuously at 4°C for 2 h, filtered through six layers of cheeseclot, and then centrifuged at 11363 g for 20 min at 4°C. The obtained supernatant was filtered through Whatman No.1 filter paper and used as the crude extract.
The crude extract was precipitated by gradient ammonium sulphate solutions, beginning with 10% concentration and increased to 20% and finally up to 90% to find the proper saturation point. The precipitate was collected by centrifugation at 11363 g for 20 min at 4°C, and the recovered solid, partially purified enzyme was dissolved in a minimum volume of 0.067 M phosphate buffer (pH 7.2) and dialyzed at 4°C with 50 volumes of the buffer with four changes during 16 h.
The obtained fractions with high activity were isolated by DEAE Sepharose Fast Flow ion-exchange column chromatography. The enzyme (12 mL) was loaded onto the column (1.6 × 20 cm), which was pre-equilibrated with 0.02 M phosphate buffer (pH 7.2). The elution of PPO was performed with increasing NaCl concentrations (0–0.6 M) in 420 mL of 0.02 M sodium phosphate buffer (pH 7.2) at a flow rate of 1 mL /min.[Citation17] The fractions were tested for enzyme activity; the fraction with the highest enzymatic activity was collected and concentrated in 10 kDa cutoff dialysis tubes. Enzyme fraction was transferred to a column (1.6 × 75 cm) filled with Sephadex G-100 gel and equilibrated with 0.067 M phosphate buffer (pH 7.2). The enzyme was eluted with the equilibration buffer by a peristaltic pump at a flow rate of 18 mL/h. The fractions were tested for enzyme activity; the fractions with higher activity were collected and concentrated and then were stored in small aliquots at −80°C for further analysis.
Denaturing SDS-PAGE and native-page
The protein samples for native and SDS-PAGE were prepared, electrophoresed, and visualised as described by Davis [Citation18] and Laemmli.[Citation19] For SDS-PAGE, the concentration of the separating gel was 10%, and the stacking gel was 4%. Proteins were stained with Coomassie Brilliant Blue R-250. Molecular weight was estimated by comparison to the molecular weight markers (RuiTaibio, Beijing, China). For Native-PAGE, the procedure was the same as SDS-PAGE, but the protein markers were obtained from GE Healthcare (Beijing, China). The substrate staining of the gel was performed with L-DOPA (0.02 M) substrate in phosphate buffer (pH 6.8, 0.067 M) for up to 40 min at 45°C until the bands became prominent.
Protein determination
Protein concentrations of the extracts obtained after each step of the PPO fractionation procedure were determined by Coomassie Brilliant Blue G-250 dye-binding using bovine serum albumin as the standard.[Citation20]
Measurement of PPO activity
PPO activity was determined by measuring the initial rate of quinone formation, as indicated by an increase in absorbance at 470 nm using a UV-2550 spectrophotometer (Shimadzu, Japan) and L-DOPA as a substrate.[Citation21,Citation22] The reaction mixture contained 1.8 mL of 0.02 M L-DOPA solution (0.067 M phosphate buffer, pH 6.8), 0.4 mL of PPO (containing 0.8% SDS), and 1.8 mL of 0.067 M phosphate buffer (pH 6.8). The blank sample contained 1.8 mL of substrate solution and 2 mL of phosphate buffer (pH 6.8). The reaction mixture was incubated at 45°C for 3 min in water bath (DK-8D, BluePard Instruments Co., Ltd, Shanghai, China), then immediately placed in the spectrophotometer, and measured every 5 s for 8 min at room temperature (25 ± 1°C). PPO activity was assayed in triplicate. One enzyme activity unit (U) was defined as an increase of 0.001 units of absorbance per minute per mL of enzyme. Relative activity was expressed as (A/Amax) × 100, where A is the increment in optical density per minute. Residual activity was expressed as (At /A0) ×100, where At is the enzyme activity after thermal treatment and A0 is the enzyme activity before thermal treatment.
Effect of temperature and pH on PPO activity
To determine the optimum temperature for the enzyme reaction, reactivity of the partially PPO was observed over a period of 3 min through temperatures ranging from 25 to 70°C (in 5°C increments) at pH 6.8 by measuring specific activity as described above. The substrate solution was heated to the appropriate temperature, and the enzyme was added. PPO activity was calculated in the form of percent relative PPO activity at the optimum temperature.[Citation23] Analyses were performed in triplicate.
The optimum pH range of the PPO was investigated by measuring its activity at 40°C with pH values ranging from 4.4 to 9.2 and with buffer at 0.05 M concentration. Acetate buffer was used for pH 4.4–5.6, phosphate buffer for pH 6–8.4, and glycine-NaOH buffer solution for pH 8.8–9.2. The value corresponding to the highest enzyme activity was taken as the optimal pH. PPO activity was calculated in the form of percent relative PPO activity at the optimum pH.[Citation24,Citation25] Analyses were performed in triplicate.
Enzyme kinetics
The kinetic parameters were measured at pH 6.8 and 45°C. To determine Michaelis constant (Km) and maximum velocity (Vmax), PPO activities were measured using L-DOPA as substrate at various concentrations (2.5, 5, 7.5, 10, 12.5, and 15 mM). Km and Vmax were calculated from a plot of 1/V vs. 1/S by the method of Lineweaver–Burk.
Thermal treatment of PPO
Thermal treatment of PPO was performed in the range of 40–80°C (in intervals of 10°C) for 0, 10, 20, 30, 40, and 50 min, respectively. After heat treatment, the samples were cooled in an ice-bath to stop further thermal inactivation, and the residual enzyme activity was assayed. Determination of PPO activity was performed using the standard assay conditions as described above. All experiments and measurements were repeated in triplicate.
First order inactivation constant (k) was calculated from the slope of the natural logarithm (ln) of At /A0 vs. time graph, where At is the enzyme activity after thermal treatment and A0 is the enzyme activity before thermal treatment. The half-life of the enzyme (t1/2) was calculated by using the following equation: t1/2 = 0.693/k. The decimal reduction time (D value) is the time, at a given temperature and pressure, needed for 90% reduction of the initial activity. Decimal reduction time (D value) was determined from the relationship between k and D value: D = ln(10)/k. The Z value, which is the temperature increase required for a 1 log10 reduction in D value, was estimated from a plot of log10D vs. temperature. The slope of the graph is equal to 1/Z value.[Citation26,Citation27]
Differential scanning calorimetry (DSC) determination of PPO
The denatured temperature of purified PPO was determined using a Netzsch 204 F1 DSC (Netzsch, Selb, Germany). Five mg of lyophilised PPO were put into the sample pool. The measured conditions were as follows: the sweep temperature: 25–140°C; the heating rate: 2°C min−1; N2: 20 mL min−1. The denatured temperature is defined as the temperature for the peak.
Fluorescence spectroscopy experiments
Intrinsic fluorescence measurements of native and thermally treated enzyme were performed using a 970CRT Fluorescence spectrophotometer (Jingke Scientific Instrument Co., Ltd, Shanghai, China) with a quartz cell of 10 mm path length. Samples were scanned at ambient temperature (25 ± 1°C). Thermal treatment of PPO was performed at temperatures in the range of 40–80°C (intervals of 10°C) for 10 min. The samples were cooled in an ice-bath immediately after heat treatment to stop further thermal inactivation. The PPO (0.05 mg /mL) were prepared using a 0.067 M phosphate buffer (pH 7.2). PPO were measured at 350 nm emission wavelength to obtain the maximum excitation wavelength and then scanned at the maximum excitation wavelength to record the emission spectra. The excitation spectrum was λex: 287 nm and λem: 300–410 nm.
Fourier transform infrared (FTIR) spectroscopy
To determine the conformational change of PPO after the thermal inactivation, the secondary structure of the enzyme was analysed using a Scimitar 2000 Near FTIR Spectrometer (Agilent Technologies Inc., Palo Alto, CA, USA). Infrared spectra were collected using thermal treatment of PPO, performed at varying temperatures in the range of 40–80°C (intervals of 10°C) for 10 min. After the heat treatment, the samples were cooled in an ice-bath to stop further thermal inactivation; then they were lyophilised and ground into the enzyme powder. After tableting enzyme samples using FTIR at 16 cm−1 resolution in the 400 cm−1, 4000 cm−1 scans were taken. Analyses of spectra and data manipulations were carried out using Peak Fitv4.12 software. After analysing the wave spectrogram of amide III and making the curve fitting residual error r2 ≥ 0.99, the secondary structure of PPO was studied.
Atomic force microscopy analysis
Samples were imaged with an atomic force microscope (AFM)(XE-70 AFM Park Systems, Suwan, Korea) according to the description previously mrentioned with some modifications.[Citation28,Citation29] The protein solutions (0.06 mg /mL) were prepared using a 0.067 M phosphate buffer (pH 7.2). Then 5 μL PPO solutions were uniformly dropped on a freshly cleaved flat mica surface and subsequently dried in a desiccator. The scan size was 5 μm. Images were processed and analysed with XEI software.
Statistical analysis
The experiments were performed in triplicate. The values are expressed as means ± standard deviations. One-way ANOVA (at the level of significance P < 0.05) was performed to ascertain the significance of the means. Statistical analysis was performed using the SPSS 19.0 programme.
Results and discussion
Purification of PPO
Enzyme activity of precipitates in the gradient saturated ammonium sulphate solutions was determined (). The highest PPO activity was observed in the fraction (50%), which was chosen for further purification. The above fraction was dialysed using a 10 kDa dialysis membrane and applied to DEAE-Sepharose Fast Flow ion-exchange column chromatography. Six protein peaks were obtained after the elution of PPO from the DEAE column (). Fractions 43–56 and 57–65 with higher PPO enzyme activity were eluted with 0.3 and 0.4 M NaCl. The PPO was not collected after elution by 0.3 M NaCl due to the high protein content and low specific activity. The most active fractions 57–65 were combined, pooled, concentrated, and applied to Sephadex G-100 gel filtration chromatography. Two protein peaks were eluted (). The first peak represented a protein with high molecular weight and enzyme activity, while the second one had low molecular weight and no enzyme activity. Thus, fractions 18–26 were collected with higher enzymatic activity on L-DOPA.
Figure 1. Purification and profiles of PPO from Penaeus vannamei. (A) Ammonium sulphate precipitation. (B) DEAE-Sepharose fast flow anion exchange chromatography. (C) Gel filtration chromatography on Sephadex G-100. (D) SDS–PAGE, M: Standard protein markers; lane 1: Purified PPO. (E) Native-PAGE, M: Native-markers; lane 1: Purified PPO stained with Coomassie brilliant R-250; lane 2: Purified PPO actively stained with L-DOPA.
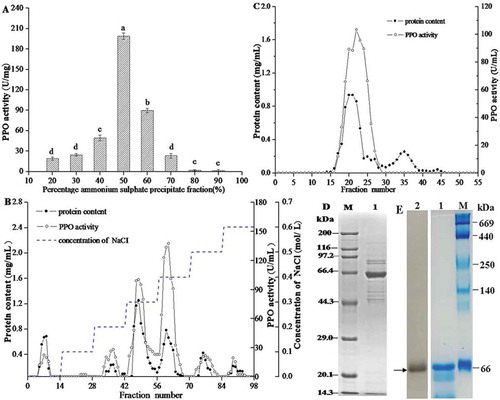
With the purification steps, the PPO mass decreased, but the specific activity increased. The characteristics of the PPO purified were given in . There was a 2.83-fold purification rate after ammonium sulphate precipitation. Following DEAE-Sepharose chromatography, the specific activity of PPO increased to 8.55 fold with a specific activity of 517.24 U/mg protein. The specific activity of PPO increased to 9.51 fold with a specific activity of 575.03 U/mg protein after a gel filtration chromatography. This significant increase in purity is attributed to the removal for most of the other proteins with low molecular weight after gel filtration chromatography from the crude extract. For example, the betalain pigments affecting the measurement of PPO activity were completely removed. The obtained enzyme was used for the further study.
Table 1. Purification of polyphenoloxidase from Penaeus vannamei using L-DOPA as the substrate
The purified PPO enzyme had one protein subunit band at 66 kDa on SDS-PAGE (). A single band was detected by in-gel enzymatic activity staining with L-DOPA (-lane2) on Native-PAGE, and the protein size was determined to be about 66 kDa by Coomassie R-250 staining (-lane1). It can be seen by the SDS-PAGE and Native-PAGE that the obtained PPO was composed by one subunit and the purified PPO was a homogeneous protein, which could meet the request for studying the PPO activity and its structure.
Qian et al. reported that PPO from the cephalothorax extracts of Pacific white shrimp had molecular weight about 160 kDa as determined by activity staining using catechol as substrate.[Citation30] However, Nirmal et al. reported that PPO from the cephalothorax extracts of Pacific white shrimp had molecular weight about 210 kDa as determined by activity staining using L-DOPA as substrate.[Citation2] Prophenoloxidase have been isolated and characterised from several crustaceans, the monomer has a mass of about 70–80 kDa, and after proteolytic activation, the active enzyme, PPO, has a mass of 60–70 kDa.[Citation31] We found the molecular mass of PPO was 66 kDa, which indicated the existence of a variety of isozymes or enzyme molecules were polymerised in varying degrees.
Optimum pH and temperature
The pH can affect the enzyme stability and may induce the irreversible destruction. It can change the charge state of the substrate and enzyme molecules, thus affecting the combination of the enzyme and substrate. The activity of purified PPO from Prawns (Penaeus vannamei) was determined at different pH values ranging from 4.4 to 9.2 using L-DOPA as substrates (). The optimal pH for L-DOPA was found to have a broad range of 6.4 to 7.6, with more than 90% of the highest activity occurring between these, and exhibiting the highest activity at pH 6.8. The results agreed with the previous work that studied purified PPO from the heads of white shrimp and found that the enzyme activity was highest between pH 6.5 and 7.5.[Citation21] Most researchers have found that PPO of most crustaceans showed only an optimal pH within the pH 6–8 range.[Citation32,Citation33]
Temperature affects the velocity of enzymatic reactions and the stability of the enzyme.[Citation34] The optimum temperature of purified PPO activity was determined at different temperatures using L-DOPA as substrates. As shown in , the enzyme was active in a wide range of temperatures (between 25°C and 55°C), and the optimum temperature of reaction was 45°C. This observation was in agreement with most research that found that the highest activity of crustacean PPO occurred in the range of 40–45°C.[Citation32,Citation33,Citation35]
Kinetic parameters
The Km for PPO of Penaeus vannamei was 2.5 mM using L-DOPA as substrate. The maximum reaction velocity (Vmax) was found to be 0.062ΔA/min (). Km is a measure of affinity of the enzyme for the substrate, with smaller values representing greater affinity. The Km value in this study was comparable to Penaeus californiensis, of which the Km is 2.5 mM assay with L-DOPA.[Citation36] Our results are in line with many studies reported Km for shrimps PPO ranging from 1.85 mM to 3.57 mM.[Citation33,Citation37,Citation38]
Thermal treatment of PPO
PPO is considered to be a thermo-instable enzyme, and the rate of enzyme activity loss increased as the temperature increased. The effects of heat treatments on PPO stability were investigated from 40°C to 80°C. As shown in , the enzyme activities decreased with increasing temperature and treatment time. At 40°C, enzyme activity of PPO decreased slightly. At 60°C, enzyme activity of PPO decreased significantly. After treating for 10 min and 30 min, the residual activity was 57.13% and 18.26%. When the temperature was raised to 80°C, the enzyme activity quickly decreased, and it retained 9.4% of its original activity after 10 min. Moreover, it was completely inactivated after 20 min (). As shown in , the denatured temperature for PPO was 65°C, which is consistent with the result for the rapid decrease of PPO activity at 60°C. Huang reported that the activity of PPO from Litopenaeus vannamei treated for 30 min at 60°C was only 38.89%.[Citation26] Giménez also reported that PPO from Nephrops norvegicus remained 40% activity after treating at 60°C for 30 min.[Citation39] These results suggested that PPO from shrimp was hard to be completely inactivated below 60°C.
Figure 4. Effects of heat treatments on residual activity (A) and DSC curve (B) of purified PPO from Penaeus vannamei.
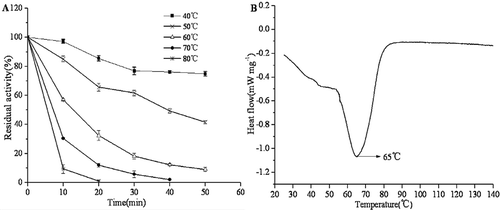
Thermal inactivation of Penaeus vannamei PPO was studied in the range 40–80°C, and the inactivation parameters are summarised in . The heat inactivation of the PPO followed first order kinetics. The inactivation constants (k values) increased with temperature increasing, indicating that PPO is less thermostable at higher temperatures. The half-life (t1/2) is another important parameter used in the characterisation of enzyme stability. When the temperature increased from 40 to 80°C, there was a significant decrease of half-life values from 105 to 3.1 min. Similar changes were observed in D values of shrimp PPO, which decreased from 348.9 to 10.2 min at the temperatures studied (). Z value for Penaeus vannamei PPO was calculated to be 27.03°C (r2 = 0.9806). These results suggested that the PPO was more susceptible at higher temperature. This is in agreement with the previous study by Huang et al., which reported the D values of PPO from Litopenaeus vannamei were 70.19 min and 0.46 min at 60°C and 100°C, respectively.[Citation26]
Table 2. Inactivation parameters of Penaeus vannamei PPO
The effects of thermal treatment on the tertiary structure of PPO
The changes in intrinsic fluorescence emission corresponded to the changes in the tertiary structure of PPO.[Citation40] When the protein was excited at 280 nm, where both tryptophan and tyrosine residues were affected, the tertiary structure of the protein was reflected.[Citation41] Intrinsic fluorescence spectra were used to monitor the tertiary conformation of PPO. The effects of thermal treatments (10 min at 40–80°C) on the fluorescence spectra of PPO are shown in . As the temperature increased, all samples subjected to thermal denaturation exhibited significant declines (P < 0.05) in fluorescence intensity. After treating at 80°C for 10 min, the fluorescence intensity declined from 226.5 to 101.5. After treating at 40, 50, 60, 70, and 80°C for 10 min, the wavelength of the fluorescence emission peak red-shifted from 338.1 nm to 338.2, 339.1, 342, 344.5, and 345.2 nm, respectively (). Red shifts of λmax were observed after the thermal treatment. Similarly, Liu et al. found a decrease of fluorescence intensity and a red shift in maximum emission wavelength, indicating that the activity of PPO in mushroom gradually decreased till an inactive state.[Citation16] These results indicated that the tertiary structural of PPO gradually changed as the temperature increases. The red shift could indicate the variations in the three-dimensional structures (secondary, tertiary, and quaternary structures) or the conformation of the enzymes.[Citation42]
Figure 5. Effects of thermal treatments on the tertiary structure of purified PPO. (A) Changes in the intrinsic fluorescence spectra of PPO by thermal treatments. Labels 1–6 indicate native, 40, 50, 60, 70, and 80°C, respectively. (B) The maximum wavelength of PPO intrinsic fluorescence at different temperatures.
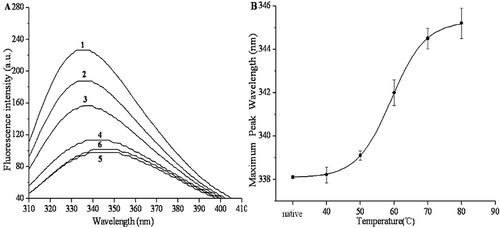
Effects of thermal treatment on the secondary structural of PPO
Fourier transform infrared spectroscopy is becoming an increasingly important method to determine the secondary structures of peptides and proteins. Among the spectral regions rising out of coupled and uncoupled stretching and bending modes of amide bonds, amide I and amide III spectral bands were found to be the most sensitive to the variations in secondary structure folding.[Citation43] Amide I was the most commonly used spectral band, primarily because of its strong signal, although it suffers from several limitations, including a strong interference from water vibrational band and overlap of revolved bands corresponding to various secondary structures. Because of the interference of water, α-helix and random coil are not far away from each other in amide I and, therefore, cannot be separated sometimes. In contrast, amide III’s spectral region (1330–1220 cm−1), albeit relatively weak in signals, does not have the above limitations. Easily resolved and better defined amide III bands are quite suitable for quantitative analysis of protein secondary structure.[Citation44] To determine the secondary structural changes during thermal treatment, curve-fitting analysis was applied to the Amide III region. Fourier self-deconvolution analysis revealed that the band located at 1330–1290 cm−1 was assigned to an α-helix structure. The peak located at 1295–1265 cm−1 was assigned to a β-turn structure. The band at 1250–1220 cm−1 was assigned to a β-sheet structure. The peak at 1270–1245 cm−1 was assigned to a random coil structure.[Citation44]
The FTIR patterns of the PPO enzymes treated at different temperature were shown in . The peak intensity of amide III band in treated PPO enzymes obviously increased compared to control. The intensity of amide III band was mainly caused by C-N stretching vibration and N-H bending vibration. The peak of PPO enzyme in this region was also significantly enhanced, but the peak position did not shift.
According to the curve-fitting analysis (r2 ≥ 0.99), untreated PPO sample had 18.18% α-helix, 29.88% β-sheet, 21.15% β-turn, and 30.79% random coil. After heated at 40°C for 10 min, secondary structure did not change much, the α-helix content decreased to 16.22%, the β-turn content decreased to 20.81%, and β-sheet content decreased to 25.77%, whereas random coil increased up to 37.20% (). These minor changes in the structure of PPO did not influence the interaction between PPO and substrate (). When thermal treatment was increased to 60°C, the α-helix content decreased to 14.05% and β-sheet content decreased to 12.18%, whereas β-turn and random coil increased to 33.06% and 40.71%, respectively. These structure changes inhibited the binding between PPO and substrate, resulted in a rapid decrease of PPO activity, and the residual enzyme activity was 57.13%. When PPO was treated at 80°C for 10 min, the α-helix and β-sheet content decreased to 11.82% and 13.54%, and the residual enzyme activity was 9.4% ().
Table 3. Secondary structure change (%) of PPO obtained by curve-fitting during thermal treatment
Decker et al.[Citation45] found that the activity centres of polyphenol oxidase in mushrooms have four α-helices and that these α-helices played a very important role in the enzymes activity.[Citation16,Citation46] In this study, we found that the α-helix of PPO decreased as the temperature increased; meanwhile, the enzyme activity was decreased, which indicated that the activity of the PPO was related to the α- helix. The increase of random coil may be mainly due to the β-sheet changes, because the secondary structure of proteins β-sheet is maintained by the hydrogen bond. Hydrogen bonds broke up as the temperature increased, peptides chains were constantly open, and PPO changed from an ordered structure to a disordered structure, resulting in the reduced β-sheet and the increase of random coil. However, the β-sheet structures are mainly located on the protein surface [Citation47], indicating that changes in the activity and thermodynamics might correlate to the unfolding of conformation in PPO.
Atomic force microscopy observation of PPO
AFM images of PPO during the heat treatment from 40°C to 80°C were shown in . The molecular groups of the untreated PPO had a mean diameter of 8.6 nm and an average height of 12 nm in the form of irregular, small flakes. PPO may take the form of a monomer, dimer, tetramer, or even connected together to be some large molecular groups in PPO solution.[Citation28,Citation48] Accordingly, we observed the enzyme molecules aggregated partially to form some small particles of aggregates at 40°C. PPO maintained most of its activity, and the residual activity was 97.21% under this condition. After treating at 50°C for 10 min, molecular groups became deformed, and some small aggregates approached together. The obvious changes were detected when the temperature increased to 60°C. The molecular groups looked like ellipsoids and had a wide diameter range (21–75.58 nm), suggesting that PPO began to denature and aggregate, which was in agreement with the results of the denatured temperature in . In , the microstructures of PPO underwent enormous changes when the temperature increased to 70°C, and most of the small molecular groups were replaced by aggregates. PPO was denatured and tended to form aggregates, resulting in a strong inactivation effect. When the temperature increased to 80°C, the shape of the aggregates did not change, but the size of the globular aggregates increased and the activity decreased. These results indicated that thermal treatment induced the great changes in microstructure of PPO, and the aggregation of PPO might influence the contact between active site and the substrate.
Conclusion
PPO was extracted from prawns (Penaeus vannamei) and purified to obtain a 9.51-fold purification rate. The optimal condition for enzyme activity was determined using L-DOPA as the substrate, showing that its optimal temperature and pH were around 45°C and 6.8, respectively. Km and Vmax values were found to be 2.5 mM and 0.062ΔA/min, respectively. When the thermal treatment was less than 10 min at 40°C, the enzyme remained fully active. When the temperature increased to 60°C, PPO activity decreased remarkably. When the temperature was raised to 80°C, the enzyme retained less than 9.4% of its original activity after 10 min. The D values of heat-treated PPO from Penaeus vannamei were 131.6 min and 44.6 min at 50°C and 60°C, respectively, and the D value decreased to 10.2 min at 80°C. Z value for the PPO was calculated to be 27.03°C. Thermal treatment resulted in the rearrangement of secondary structure and disruption of tertiary structure. A significant decrease in fluorescence intensity was recorded, indicating that thermal denaturation directly induced the tertiary structural changes of PPO. FTIR results revealed that the secondary structural changes in PPO resulted from thermal treatment. In addition, PPO molecules were deformed and inactivated at 60°C, while higher temperature induced the formation of large aggregates and the inactivation of PPO. Further studies could be carried out to clarify the inactivation mechanism of the PPO in food systems.
Acknowledgements
This work was supported by the Research Project from Science & Technology Department of Liaoning Province of China (No. 2015103020).
References
- Wang, J. J.; Lin, T.; Li, J. B.; Liao, C.; Pan, Y. J.; Zhao, Y. Effect of Acidic Electrolyzed Water Ice on Quality of Shrimp in Dark Condition. Food Control. 2014, 35, 207–212. DOI: 10.1016/j.foodcont.2013.07.005.
- Nirmal, N. P.; Benjakul, S. Effect of Ferulic Acid on Inhibition of Polyphenoloxidase and Quality Changes of Pacific White Shrimp (Litopenaeus Vannamei) during Iced Storage. Food Chem. 2009, 116, 323–331. DOI: 10.1016/j.foodchem.2009.02.054.
- Encarnacion, A. B.; Fagutao, F.; Shozen, K. I.; Hirono, I.; Ohshima, T. Biochemical Intervention of Ergothioneine-Rich Edible Mushroom (Flammulina Velutipes) Extract Inhibits Melanosis in Crab (Chionoecetes Japonicus). Food Chem. 2011, 127, 1594–1599. DOI: 10.1016/j.foodchem.2011.02.023.
- Nirmal, N. P.; Benjakul, S. Effect of Catechin and Ferulic Acid on Melanosis and Quality of Pacific White Shrimp Subjected to Prior Freeze–Thawing during Refrigerated Storage. Food Control. 2010, 21, 1263–1271. DOI: 10.1016/j.foodcont.2010.02.015.
- Cabanes, J.; García-Cánovas, F.; García-Carmona, F. Chemical and Enzymatic Oxidation of 4-Methylcatechol in the Presence and Absence of L-Serine. Spectrophotometric Determination of Intermediates. Biochim. Biophys. Acta. 1987, 914, 190–197. DOI: 10.1016/0167-4838(87)90063-X.
- Fulcrand, H.; Cheminat, A.; Brouillard, R.; Cheynier, V. Characterization of Compounds Obtained by Chemical Oxidation of Caffeic Acid in Acidic Conditions. Phytochemistry. 1994, 35, 499–505. DOI: 10.1016/S0031-9422(00)94790-3.
- Ludikhuyze, L.; Van Loey, A.; Indrawati,; Smout, C.; Hendrickx, M. Effects of Combined Pressure and Temperature on Enzymes Related to Quality of Fruits and Vegetables: From Kinetic Information to Process Engineering Aspects. Crit. Rev. Food Sci. Nutr. 2003, 43, 527–586. DOI: 10.1080/10408690390246350.
- López-Caballero, M. E.; Martínez-Álvarez, Ó.; Gómez-Guillén, M. C.; Montero, P. Effect of Natural Compounds Alternative to Commercial Antimelanosics on Polyphenol Oxidase Activity and Microbial Growth in Cultured Prawns (Marsupenaeus Tiger) during Chilled Storage. Eur. Food Res. Technol. 2006, 223, 7–15. DOI: 10.1007/s00217-005-0049-3.
- Basiri, S.; Shekarforoush, S. S.; Aminlari, M.; Akbari, S. The Effect of Pomegranate Peel Extract (PPE) on the Polyphenol Oxidase (PPO) and Quality of Pacific White Shrimp (Litopenaeus Vannamei) during Refrigerated Storage. LWT-Food Sci. Technol. 2015, 60, 1025–1033. DOI: 10.1016/j.lwt.2014.10.043.
- Nirmal, N. P.; Benjakul, S. Inhibition Kinetics of Catechin and Ferulic Acid on Polyphenoloxidase from Cephalothorax of Pacific White Shrimp (Litopenaeus Vannamei). Food Chem. 2012, 131, 569–573. DOI: 10.1016/j.foodchem.2011.09.025.
- Manheem, K.; Benjakul, S.; Kijroongrojana, K.; Visessanguan, W. The Effect of Heating Conditions on Polyphenol Oxidase, Proteases and Melanosis in Pre-Cooked Pacific White Shrimp during Refrigerated Storage. Food Chem. 2012, 131, 1370–1375. DOI: 10.1016/j.foodchem.2011.10.001.
- Ndiaye, C.; Xu, S. Y.; Wang, Z. Steam Blanching Effect on Polyphenoloxidase, Peroxidase and Colour of Mango (Mangifera Indica L.) Slices. Food Chemistry. 2009, 113, 92–95. DOI: 10.1016/j.foodchem.2008.07.027.
- Schweiggert, U.; Schieber, A.; Carle, R. Inactivation of Peroxidase, Polyphenoloxidase, and Lipoxygenase in Paprika and Chili Powder after Immediate Thermal Treatment of the Plant Material. Innov. Food Sci. Emer. Tech. 2005, 6, 403–411. DOI: 10.1016/j.ifset.2005.05.001.
- Chutintrasri, B.; Noomhorm, A. Thermal Inactivation of Polyphenoloxidase in Pineapple Puree. LWT-Food Sci. Technol. 2006, 39, 492–495. DOI: 10.1016/j.lwt.2005.04.006.
- Gouzi, H.; Depagne, C.; Coradin, T. Kinetics and Thermodynamics of the Thermal Inactivation of Polyphenol Oxidase in an Aqueous Extract from Agaricus Bisporus. J. Agric. Food Chem. 2012, 60, 500–506. DOI: 10.1021/jf204104g.
- Liu, W.; Zou, L. Q.; Liu, J. P.; Zhang, Z. Q.; Liu, C. M.; Liang, R. H. The Effect of Citric Acid on the Activity, Thermodynamics and Conformation of Mushroom Polyphenoloxidase. Food Chem. 2013, 140, 289–295. DOI: 10.1016/j.foodchem.2013.02.028.
- Aydin, B.; Gulcin, I.; Alwasel, S. H. Purification and Characterization of Polyphenol Oxidase from Hemşin Apple (Malus Communis L.). Int. J. Food Properties. 2015, 18, 2735–2745. DOI: 10.1080/10942912.2015.1012725.
- Davis, B. J.;. Disc electrophoresis-II Method and Application to Human Serum Proteins. Ann. New York Acad. Sci. 1964, 121, 404–427. DOI: 10.1111/j.1749-6632.1964.tb14213.x.
- Laemmli, U. K.;. Cleavage of Structural Proteins during the Assembly of the Head of Bacteriophage T4. Nature. 1970, 227, 680–685. DOI: 10.1038/227680a0.
- Bradford, M. M.;. A Rapid Sensitive Method for the Quantification of Microgram Quantities of Protein Utilizing the Principle of Protein-Dye Binding. Anal. Biochemistr. 1976, 72, 248–254. DOI: 10.1016/0003-2697(76)90527-3.
- Simpson, B. K.; Marshall, M. R.; Otwell, W. S. Phenoloxidase from Shrimp (Penaues Setiferus): Purification and Some Properties. J. Agric. Food Chem. 1987, 35, 918–921. DOI: 10.1021/jf00078a017.
- Gülçin, İ.; Küfrevioğlu, Ö. İ.; Oktay, M. Purification and Characterization of Polyphenol Oxidase from Nettle (Urtica Dioica L.) and Inhibitory Effects of Some Chemicals on Enzyme Activity. J. Enzyme Inhib. Med. Chem. 2005, 20, 297–302. DOI: 10.1080/1475636032000141890.
- Ünal, M. Ü.; Şener, A. Two-Year Comparison of the Biochemical Properties of Polyphenol Oxidase from Turkish Alyanak Apricot (Prunus Armenica L.). Food Chem. 2016, 190, 741–747. DOI: 10.1016/j.foodchem.2015.06.025.
- Gülçin, İ.; Yildirim, A. Purification and Characterization of Peroxidase from Brassica Oleracea Var. Acephala. Asian J. Chem. 2005, 17, 2175–2183.
- Köksal, E.; Gülçin, İ. Purification and Characterization of Peroxidase from Cauliflower (Brassica Oleracea L.Var. Botrytis) Buds. Protein Pept. Lett. 2008, 15, 320–326. DOI: 10.2174/092986608784246506.
- Huang, W. Y.; Ji, H. W.; Liu, S. C.; Zhang, C. H.; Chen, Y. L.; Guo, M. H.; Hao, J. M. Inactivation Effects and Kinetics of Polyphenol Oxidase from Litopenaeus Vannamei by Ultra-High Pressure and Heat. Innov. Food Sci. Emerging Tech. 2014, 26, 108–115. DOI: 10.1016/j.ifset.2014.10.005.
- Ünal, M. Ü.; Şener, A.; Şen, K. Characterization of Sultaniye Grape (Vitis Vinifera L. Cv. Sultana) Polyphenol Oxidase. Int. J. Food Sci. Technol. 2007, 42, 1123–1127. DOI: 10.1111/j.1365-2621.2006.01406.x.
- Yu, Z. L.; Zeng, W. C.; Lu, X. L. Influence of Ultrasound to the Activity of Tyrosinase. Ultrason. Sonochem. 2013, 20, 805–809. DOI: 10.1016/j.ultsonch.2012.11.006.
- Zhou, L.; Liu, W.; Zou, L. Q.; Xiong, Z. Q.; Hu, X. T.; Chen, J. Aggregation and Conformational Change of Mushroom (Agaricus Bisporus) Polyphenoloxidase Subjected to Thermal Treatment. Food Chem. 2017, 214, 423–431. DOI: 10.1016/j.foodchem.2016.07.041.
- Qian, Y. F.; Xie, J.; Yang, S. P.; Wu, W. H.; Xiong, Q.; Gao, Z. L. Vivo Study of Spoilage Bacteria on Polyphenoloxidase Activity and Melanosis of Modified Atmosphere Packaged Pacific White Shrimp. Food Chem. 2014, 155, 126–131. DOI: 10.1016/j.foodchem.2014.01.031.
- Söderhäll, K.; Cerenius, L. Role of the Prophenoloxidase-Activating System in Invertebrate Immunity. Curr. Opin. Immunol. 1998, 10, 23–28. DOI: 10.1016/S0952-7915(98)80026-5.
- Liu, G. X.; Yang, L. L.; Fan, T. J.; Cong, R. S.; Tang, Z. H.; Sun, W. J.; Meng, X. H.; Zhu, L. Y. Purification and Characterization of Phenoloxidase from Crab Charybdis Japonica. Fish. Shellfish Immunol. 2006, 20, 47–57. DOI: 10.1016/j.fsi.2005.03.012.
- Zamorano, J. P.; Martínez-Álvarez, O.; Montero, P.; Gómez-Guillén, M. D. C. Characterisation and Tissue Distribution of Polyphenol Oxidase of Deepwater Pink Shrimp (Parapenaeus Longirostris). Food Chem. 2009, 112, 104–111. DOI: 10.1016/j.foodchem.2008.05.061.
- Liu, N. N.; Liu, W.; Wang, D. J.; Zhou, Y. B.; Lin, X. J.; Wang, X.; Li, S. B. Purification and Partial Characterization of Polyphenol Oxidase from the Flower Buds of Lonicera Japonica Thunb. Food Chem. 2013, 138, 478–483. DOI: 10.1016/j.foodchem.2012.10.103.
- Ali, M. T.; Marshall, M. R.; Wei, C. I.; Gleeson, R. A. Monophenol Oxidase Activity from the Cuticle of Florida Spiny Lobster (Panulirus Argus). J. Agric. Food Chem. 1994, 42, 53–58. DOI: 10.1021/jf00037a008.
- Gollas-Galván, T.; Hernández-López, J.; Vargas-Albores, F. Prophenoloxidase from Brown Shrimp (Penaeus Californiensis) Hemocytes. Comp. Biochemistry Physiol. B. 1999, 122, 77–82. DOI: 10.1016/S0305-0491(98)10143-8.
- Chen, J. S.; Rolle, R. S.; Marshall, M. R.; Wei, C. I. Comparison of Phenoloxidase Activity from Florida Spiny Lobster and Western Australia Lobster. J. Food Sci. 1991, 56, 154–160. DOI: 10.1111/j.1365-2621.1991.tb07999.x.
- Fan, T. J.; Wang, X. F. Purification and Partial Biochemical Characterization of Phenoloxidase from Penaeus Chinensis. Acta Biochim. Biophys. Sin. 2002, 34, 589–594.
- Giménez, B.; Martínez-Alvarez, Ó.; Montero, P.; Gómez-Guillén, M. D. C. Characterization of Phenoloxidase Activity of Carapace and Viscera from Cephalothorax of Norway Lobster (Nephrops Norvegicus). LWT-Food Sci. Technol. 2010, 43, 1240–1245. DOI: 10.1016/j.lwt.2010.02.017.
- Zhou, L. Y.; Wu, J. H.; Hu, X. S.; Zhi, X.; Liao, X. J. Alterations in the Activity and Structure of Pectin Methylesterase Treated by High Pressure Carbon Dioxide. J. Agric. Food Chem. 2009, 57, 1890–1895. DOI: 10.1021/jf803501q.
- Sun, N.; Lee, S.; Song, K. B. Effect of High-Pressure Treatment on the Molecular Properties of Mushroom Polyphenoloxidase. LWT-Food Sci. Technol. 2002, 35, 315–318. DOI: 10.1006/fstl.2001.0871.
- Ho, S. Y.; Mittal, G. S.; Cross, J. D. Effects of High Field Electric Pulses on the Activity of Selected Enzymes. J Food Eng. 1997, 31, 69–84. DOI: 10.1016/S0260-8774(96)00052-0.
- Haris, P. I.; Severcan, F. FTIR Spectroscopic Characterization of Protein Structure in Aqueous and Non-Aqueous Media. J. Mol. Catalysis. B: Enzymatic. 1999, 7, 207–221. DOI: 10.1016/S1381-1177(99)00030-2.
- Cai, S. W.; Singh, B. R. Identification of Β-Turn and Random Coil Amide III Infrared Bands for Secondary Structure Estimation of Proteins. Biophys. Chem. 1999, 80, 7–20. DOI: 10.1016/S0301-4622(99)00060-5.
- Decker, H.; Schweikardt, T.; Tuczek, F. The First Crystal Structure of Tyrosinase: All Questions Answered? Angewandte Chemie Int. Edn. 2006, 45, 4546–4550. DOI: 10.1002/(ISSN)1521-3773.
- Yi, J. Y.; Jiang, B.; Zhang, Z.; Liao, X. J.; Zhang, Y.; Hu, X. S. Effect of Ultrahigh Hydrostatic Pressure on the Activity and Structure of Mushroom (Agaricus Bisporus) Polyphenoloxidase. J. Agric. Food Chem. 2012, 60, 593–599. DOI: 10.1021/jf203405u.
- Aprodu, I.; Stănciuc, N.; Dumitraşcu, L.; Râpeanu, G.; Stanciu, S. Investigations Towards Understanding the Thermal Denaturation of Lactoperoxidase. Int. Dairy J. 2014, 38, 47–54. DOI: 10.1016/j.idairyj.2014.03.013.
- Yi, J. J.; Yi, J. Y.; Dong, P.; Liao, X. J.; Hu, X. S.; Zhang, Y. Effect of High-Hydrostatic-Pressure on Molecular Microstructure of Mushroom (Agaricus Bisporus) Polyphenoloxidase. LWT-Food Sci. Technol. 2015, 60, 890–898. DOI: 10.1016/j.lwt.2014.10.027.