ABSTRACT
Milkfat was fractionated at 40°C using supercritical carbon dioxide (CO2) at pressures of 10, 15, and 20 MPa. Fractions were collected for 6 h at each pressure and evaluated for extraction yield, fatty acid composition, thermal transition temperatures, and shear crystallization behavior. The mass extraction yields at 10, 15, and 20 MPa were 34%, 26%, and 33%, respectively, with the remaining raffinate representing ~7% of the starting milkfat. As a function of time within each successive pressure, there was a decrease in the proportion of short-chain (C4–C8), medium-chain (C10–C14), and total saturated fatty acids as well as an increase in long-chain (C18–C18:2) and total unsaturated fatty acids extracted. Shear showed little effect on the growth behavior of any fractions, but showed large effects on nucleation onset. This study showed that the composition and crystallization behavior of fractionated milkfat can be greatly tailored based on supercritical CO2 pressure and extraction time.
Introduction
As milkfat boasts a unique taste, melting behavior, and textural profile, it forms the basis of many dairy and processed food products. With its complex fatty acid and triacylglycerol (TAG) composition, it is liquid above 40°C but completely solid at −40°C.[Citation1] Animal source and feed, seasonal growth conditions, as well as geographical origin greatly affect its physicochemical properties.[Citation2] Milkfat’s wide melting range limits its use in applications where an appropriate solid fat content and texture are critical.[Citation3] Fractionation is a practical means to widen its range of applications in dairy (e.g., spreadable butters) and non-dairy (e.g., confectionery fillings) products.[Citation4,Citation5]
Separation into fractions with discrete melting ranges is possible using different means.[Citation6,Citation7] Physical separation approaches include dry and solvent fractionation, short path distillation, and supercritical fluid extraction (SFE). Dry and solvent fractionation are the simplest and involve two key steps: (i) crystallization of TAGs based on their melting points and (ii) ensuing separation,[Citation1,Citation5,Citation8] with the latter also requiring a solvent removal step. Short-path distillation permits separation of fats based on TAG boiling points under vacuum and can result in distillates enriched in volatile and low-molecular-weight components or higher-molecular-weight TAGs depending on the temperature and applied vacuum used.[Citation9]
SFE is a separation process where the properties of a solvent in a supercritical state can selectively extract specific compounds or to fractionate mixtures based on solvent temperature and pressure. Supercritical carbon dioxide (SC-CO2) is an attractive alternative to conventional extraction using organic solvents given increasing public awareness of the health, and environmental and safety hazards associated with organic solvents in food processing and possible solvent contamination. It has been used for the extraction and fractionation of lipid-bearing materials,[Citation10,Citation11] decaffeination,[Citation12] and the extraction of cholesterol,[Citation13] aromas,[Citation14] and antioxidants from fats and oils.[Citation15–Citation17]
Several studies on milkfat fractionation with SC-CO2 have been reported with the fractions obtained consisting of markedly different physical properties and compositions.[Citation5,Citation18,Citation19] For example, Chen et al.[Citation20] fractionated milkfat into low, intermediate, and high-melting fractions using different extraction pressures. Sequential fractionation can separate fats into fractions with more distinct compositions compared to standard SC-CO2 fractionation. This approach is straightforward and involves separation at different SC-CO2 pressures. Initially, a lower pressure is used, which is then increased to further separate a fat depleted in lower-melting TAGs (LMTs) and volatile compounds into higher-melting species. This approach permits the efficient separation of milkfat TAGs in multiple fractions with a wide melting range and distinctive compositional and sensory profiles.[Citation21]
The solidification of fat in processed foods often involves shear and mixing. Crystallization occurs via nucleation and crystal growth. When a molten fat is cooled to a temperature below the melting point of its highest-melting TAG, known as undercooling (usually at least 5–10°C), it will become supersaturated in the highest-melting TAG species and drive the system toward nucleation.[Citation22–Citation25] Shear may increase the rate of primary nucleation by providing enough energy to overcome activation energy barriers for nucleation, which may result in a larger number of smaller crystals. Additionally, shear may accelerate crystallization rates, resulting in higher initial solid fat content. Shear may also enhance secondary nucleation by fracturing newly formed crystals and distributing them throughout the melt, and providing an increased number of seeds/interfaces for nucleation, though these phenomena are shear rate-dependent. Finally, shear can greatly accelerate phase transitions to more stable polymorphs.[Citation26]
Though milkfat fractionation has been carried out in various forms for decades, there currently exists a dearth of information regarding its crystallization behavior, in particular under shear.[Citation27,Citation28] The objectives of the present study were twofold: (i) elucidate the effect of sequential SC-CO2 fractionation on the composition and crystallization/melting behavior of milkfat, where fatty acid composition as well as thermal and rheological properties were analyzed and compared against the original milkfat; and (ii) explore the flow-induced crystallization (FIC) of milkfat fractions, which we evaluated as a function of SC-CO2 pressure and extraction time.
Materials and methods
Milkfat
Cow milk obtained from a local farm in October was immediately pasteurized at 70°C for 5 min and cooled to 42°C. Milkfat was separated from butter according to a previous procedure.[Citation29]
Fractionation
Fractionation was carried out using a laboratory-scale SC-CO2 system (Model SFE-500F-2, Thar Technologies Inc., Pittsburgh, PA, USA) that consisted of a high-pressure CO2 pump, heat exchanger, automatic back pressure regulator, three 500 cm3 stainless steel vessels, and a thermostated circulating bath (Model K10, Thermo Scientific, Karlsruhe, Germany).
For the sequential fractionation, 100 g of melted anhydrous milkfat was placed in the first vessel where the pressure was maintained at 9 MPa for 30 min to allow sufficient contact time between the milkfat and SC-CO2. It was then adjusted to 10 MPa for the first fractionation run by using an automated back pressure regulator. The pressure in the second vessel was then adjusted to 5 MPa to allow transfer from the first vessel to the second vessel. A manual valve between the second and third vessels was opened along with a vent to keep the pressure and CO2 flow constant. Fractions were collected hourly from the bottom of the second vessel at the operational pressure. The procedure was repeated with pressures of 15 and 20 MPa. Each run was completed in 6 h at a 50 mL/min CO2 flow rate. In all, 16 fractions plus the raffinate (the remaining milkfat) were collected from the same starting batch sample. The procedure was duplicated.
Fatty acid profile
Gas chromatography was performed by converting the fractionated TAGs to their corresponding fatty acid methyl esters (FAME) by transesterification in a KOH/CH3OH solution at room temperature.[Citation30] A 7890A model gas chromatography system (Agilent Technologies, Santa Clara, CA, USA) equipped with a flame ionization detector, a split/splitless injector (operated with a split ratio of 1:40), and capillary column HP-88 (100 m × 0.25 mm ID × 0.20 μm film thickness, coated with 88% cyanopropyl-methylaryl polysiloxane) was used. Helium was used as the carrier gas. The injection temperature was 250°C. The oven temperature was kept at 120°C for 1 min, then heated from 120°C to 175°C at 10°C/min, then to 210°C at 5°C/min to 230°C and held for 5 min.
Fatty acids were identified by comparison of retention times against a known standard containing the FAME mix from Sigma-Aldrich (St. Louis, MO, USA). Fatty acids were calculated as the ratio of the partial area to the total peaks area. They were analyzed in duplicate for each fraction and expressed as g/100 g of fat.
Thermal behavior
Melting and crystallization thermograms were obtained with a Perkin-Elmer Model DSC4000 differential scanning calorimeter (DSC), equipped with a mechanical cooler. The DSC was calibrated with an indium standard. Samples of ~10 mg were weighed in aluminum measuring pans, which were sealed and placed in the calorimeter. An empty sealed pan was used as reference. Thermograms were determined by holding the samples for 5 min at 70°C to completely melt the fat and eliminate all crystal nuclei, after which the samples were cooled to −50°C at a cooling rate of 5°C/min. The samples kept 5 min at −50°C were than heated to 70°C at 5°C/min. All experiments were performed in duplicate. Thermograms were analyzed to determine the crystallization/melting temperatures observed.
Rheological measurement
Molten milkfat and fractions were deposited between the parallel plates (PP25, 25 mm diameter, 0.5 mm gap) of a controlled strain rheometer (Physica MCR301, Anton Paar, Ville St-Laurent, QC, Canada). Samples were maintained at 70°C for 5 min and cooled at 1.0°C/min while being sheared at 10, 25, 50, 100, or 300 s−1. The apparent viscosity of the samples was measured using the rheometer, and measurements were recorded as samples were sheared and cooled. All experiments were performed in duplicate.
Statistical analysis
Analysis of variance was performed using Statgraphics Plus (Statistical Graphics Corp., Washington, USA). Duncan’s multiple-range test was used to compare sample means. Evaluations were based on a 5% significance level (p < 0.05).
Results and discussion
The fractionation yields at 10, 15, and 20 MPa were 34, 26, and 33 g/100 g milkfat, respectively (). The weight of the raffinate (the high-molecular weight remnant) was 7 g/100 g milkfat. At 10 MPa, the extent of fractionation initially increased with time and then decreased after 3 h as the lower-melting species soluble became depleted.[Citation31] The extraction rate at 15 MPa was lower than at 10 MPa, given the apparent lack of soluble components at this pressure. Fractionation at 20 MPa was linear over 6 h and showed a yield similar to 10 MPa. At room temperature, all 10 MPa fractions were liquid, whereas those collected at 15 MPa were semi-solid and those at 20 MPa were solid. All fractions were colorless, except for the yellowish raffinate and 20 MPa-6 h fraction, which we ascribed to the presence of β-carotene.[Citation32,Citation33]
Fatty acid composition
The major fatty acids in milkfat were myristic, palmitic, stearic, and oleic acids (). With increased pressure, the concentration of short-chain (C4:0–C8:0) and medium-chain (C10:0–C14:0) fatty acids decreased, whereas the long-chain (C16:0–C18:2) and total unsaturated fatty acids increased. Significant differences (p > 0.05) were found between fatty acid composition of the fractions. This trend is similar to those found for the fractionation of milkfat by other researchers.[Citation1,Citation21,Citation34]
Table 1. Fatty acid composition of milkfat and its fractions (g/100 g).
The percent fatty acid extracted was calculated using the weight of fatty acid in each fraction and weight of fatty acid in original milkfat at the end of each fractionation step. shows the distribution of the percent fatty acids extracted at each pressure and in the raffinate. The fractions collected at 10 MPa contained TAGs enriched in short-chain fatty acids, whereas at 15 MPa, there was no preference in fatty acid type extracted. [Citation1,Citation11,Citation20,Citation34] The 20 MPa fractions and raffinate were richest in long-chain fatty acids, namely stearic, oleic, and linoleic acids.
The ratio of unsaturated to saturated fatty acids increased from 10 to 20 MPa, ranging from 0.3 to 0.8 as compared to a value of 0.5 for milkfat. Unsaturation of the fractions increases due to an increase in long-chain unsaturated fatty acids (C18:1–C18:2) and a decrease in low- and medium-chain saturated fatty acids with increased pressure. This suggested that SC-CO2 affected a high degree of molecular weight separation. Fractionation of milkfat TAGs by SC-CO2 depends on solute–solvent interactions and affinity leading to TAG miscibility in the SC-CO2 phase. As the density of SC-CO2 increases with pressure, the mutual affinity of the supercritical fluid and TAG molecules correspondingly increases. Thus, low-density SC-CO2 was able to extract short-chain fatty acids with subsequent increases in SC-CO2 pressure increasing SC-CO2 density, and consequently its solvating power.[Citation34] Thus, changes in pressure yielded fractions markedly different composition.
Thermal behavior
Melting and crystallization thermograms of milkfat and fractions ( and , respectively) agreed with previous studies.[Citation1,Citation35] The melting thermogram of milkfat exhibited three endotherms representing the low- (LMT), medium- (MMT), and high-melting TAGs (HMT) (). At 10 MPa, only the LMT and MMT were initially extracted with the HMT appearing after 3 h (). At 15 MPa, the HMT became very pronounced such that by 6 h of fractionation it was nearly equal in intensity to the MMT (). At 20 MPa, the HMT dominated all melting thermograms (). The raffinate consisted of mostly HMTs along with small amounts of LMTs and middle-melting TAGs ().
Figure 3. Melting thermograms of fractions produced at (a) 10, (b) 15, (c) 20 MPa, and (d) milkfat and raffinate.
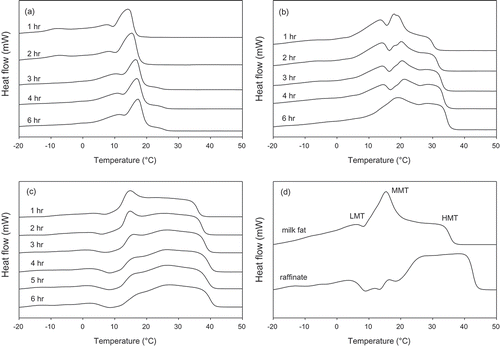
The 10 MPa fractions were composed largely of the liquid portion of the milkfat with clear points between 16.9°C and 28.2°C. There was almost equal distribution of fatty acids in 15 MPa fractions (). The 15 MPa fractions had clear points between 31.6°C and 35.8°C. The 20 MPa fractions and raffinate were composed largely of the solid portion with clear points between 37.5°C and 41.8°C and 44.7°C, respectively. The clear points of the fractions progressively increased from 10 MPa to raffinate. The crystallization thermograms of the 10 and 15 MPa fractions were nearly identical and consisted of peaks at 6–8°C and 9–10°C, respectively (). At 20 MPa, two crystallization peaks at 5–6°C and 12–15°C were joined by a third peak after 5 and 6 h ~20°C. The raffinate showed a high-melting fraction at 20°C. The crystallization thermogram of milkfat was similar to the 20 MPa-1 h fraction.
Rheology
shows the temperature-dependent viscosity profile of milkfat and its fractions sheared-cooled at 10–300 s−1 and at 1°C/min. A putative three-region viscosity profile (I−III) was found for most fractions at the different shear rates ().[Citation36] The increase in viscosity (region I) was attributed to the increased molten state interactions through van der Waals attraction between neighboring TAGs as the temperature is reduced. Region II, visible as the sharp increase in viscosity within a relatively narrow temperature range, resulted from the nucleation of HMTs. The region III viscosity profile was attributed to three concurrent events: de novo nucleation and growth of LMTs, as well as the possible growth and polymorphic transitions of pre-existing TAGs.[Citation37] Clear differences in shear viscosity profile were apparent among the different milkfat fractions.
Figure 5. Viscosity profiles of milkfat and its fractions at 1.0°C/min cooling rate as a function shear rate: (a) viscosity–temperature profile, (b) 10, (c) 25, (d) 50, (e) 100, and (f) 300 s−1.
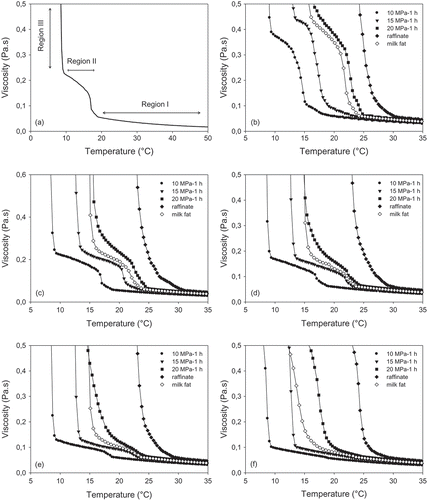
The three-region viscosity profile of milkfat was readily apparent at 10–50 s−1 but not so at 100–300 s−1, where the viscosity increase followed an exponential growth profile, with region II no longer apparent. Shear rate did not affect significantly crystal growth onset (p > 0.05), with all shear rates resulting in a milkfat TAG growth onsets of ~15°C (). However, the rate of increase in viscosity for phase II significantly differed with shear rate and composition, implying that the interaction and ordering of HMTs into crystals capable of increasing viscosity was significantly retarded by shear. Similar observations also apply to the FIC profiles of the various milkfat fractions.
Table 2. Nucleation and crystallization onset temperatures (°C) of milkfat and its fractions based on shear crystallization.
At 10–100 s−1, the three-region pattern was readily apparent with the 10 and 15 MPa fractions, but was less defined with the 20 MPa fractions, which exhibited a less-defined region II. The raffinate exhibited an exponential growth in viscosity with no region II visible similar to most shear crystallization curves at 50–300 s−1 (–). The region III crystal growth onset temperatures of all fractions are shown in . Shear rate did not impact the growth onset temperatures of the milkfat and fractions produced at 10 and 15 MPa. Increases of 1–2°C for fractions produced at 20 MPa were seen with increasing shear rate. By contrast, the raffinate showed a slight decrease in nucleation and growth onset temperatures with increasing shear rate.
A clear effect of shear rate on nucleation temperature was evident. Within each fraction, these temperatures increased with shear rate, though this depended on extraction pressure. For example, the nucleation onset temperature following 6 h of extraction at 10 MPa increased from ~16°C to 22°C upon increasing shear rate from 10 to 300 s−1. Such an increase was ascribed to the increased collision frequency and ordering of TAG and promotion of shear-induced mass transfer. At 20 MPa-6 h, however, the 1°C increase was ascribed to the gradually higher proportion of oleic acid-based TAGs in these fractions. Finally, the raffinate exhibited a slight decrease in nucleation induction temperature with increasing shear.
Shear rate showed little effect on growth rate temperatures, with increases of only up to 1°C at higher shear rates. The effect of shear on crystallization has been studied in fat; especially cocoa butter and palm oil.[Citation37,Citation38] Increasing shear rates have been shown to enhance crystallization rate and polymorphic transition in all these fats.[Citation39–Citation41] de Graef et al.[Citation25] and Mazzanti et al.[Citation40] showed that shear did not influence the onset of formation of α-crystals in palm oil, while the onset of β’ formation was enhanced with increasing shear rate. In our case, high shear rates did not greatly affect nucleation. This was surprising as it was assumed that it would increase due to flow-induced collisions, resulting in a decrease in melt entropy and the associated barrier to nucleation. Under quiescent conditions, with insufficient supercooling, nuclei will be unstable due to the free energy cost of their interface with the liquid. This free energy nucleation barrier can be overcome by sufficient supercooling. As the nucleation rate depends on the barrier height, any change in this barrier will alter nucleation rate and nucleus density.
There was a slight decrease in nucleation onset in the raffinate, suggesting that HMTs behaved differently from their lower-melting counterparts under shear. That is, melts with a range of HMTs contributed more strongly to FIC as these TAGs associated more readily under flow. Thus measurements from polydisperse materials contain simultaneous contributions from many different molecular lengths, each executing widely differing dynamics.
Certain TAG families were more sensitive to shear than others, in particular those that form at higher temperature, which suggested a link between composition and shear on crystallization. For example, based on the fatty acid profile (), there was a correlation (r2 = 0.84) between palmitic acid content and the change in crystal growth onset within each fraction as a function of shear rate (). The fraction with the highest palmitic acid content (10 MPa, 6 h) saw its crystal growth onset temperature increase by 6°C upon increasing shear rate from 10 to 300 s−1. By contrast, the fractions with the lowest palmitic acid content (the raffinate and 20 MPa fractions at 1 and 6 h) had the smallest change in onset temperature. Though we cannot directly correlate fatty acid and TAG content, these results demonstrate that specific components with the milkfat TAGs played a dominant role of nucleation and growth.
Figure 6. Correlation of palmitic acid content and the change in nucleation onset temperature with shear rate from 10 to 300 s−1.
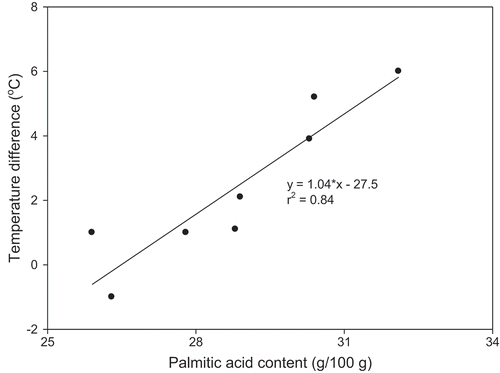
The effect of shear on fat crystallization consists of two competing factors whereby below a critical shear rate nucleation and/or growth will be enhanced due to flow-mediated transport of TAG molecules toward existing crystal nuclei. Above a critical shear value, deposition of TAG molecules onto nuclei will be hindered by the presence of an energy barrier that lowers nucleation. Comparing the extreme cases of the 10 MPa-1 h and 20 MPa-6 h fractions, the key differentiator in the shear-induced crystallization of milkfat was composition. At 10 MPa, the greater compositional variety, in particular the presence of LMTs, resulted in a more gradual series of crystallization events as evidenced by the clear three-region shear profiles (). Such gradual crystallization was more sensitive to shear-induced TAG–TAG, TAG–nuclei, and/or nuclei–nuclei collisions. Hence, greater shear accelerated these events, resulting in crystallization onsets at higher temperatures. Conversely, the lack of evolution in onset temperatures for the 20 MPa-6 h fraction implied that shear rate had little effect on nucleation and growth. This was the result of its more limited composition, which resulted in more rapid crystallization and thus a reduced effect of shear once nucleation and growth had been initiated.
Conclusion
Sequential SC-CO2 is a viable tool to extract fractions from milkfat with diverse compositions and solidification behaviors. In general terms, fractions extracted at lower pressures (i.e., 10 MPa) and for shorter times (i.e., 1–2 h) were enriched in shorter fatty acids, whereas those extracted at higher pressures (i.e., 20 MPa) and longer times (i.e., ~6 h) contained larger amounts of long-chained fatty acids and unsaturated fatty acids. The correlation between composition and crystallization behavior certainly warrants further attention as it may lead to more precise means to control the rate of fat crystallization in complex mixtures.
Conflict of interest
The authors have declared no conflict of interest.
Acknowledgment
The Scientific and Technical Council of Turkey (TÜBİTAK) is gratefully acknowledged for funding support.
References
- Bhaskar, A. R.; Rizvi, S. S. H.; Bertoli, C.; Fay, L. B.; Hug, B. A. Comparison of Physical and Chemical Properties of Milkfat Fractions Obtained by Two Processing Technologies. J. Am. Oil Chem. Soc. 1998, 75, 1249–1264.
- Hinrichs, J.; Heineman, U.; Kessler, H. G. Differences in the Composition of Triglycerides in Summer and Winter Milkfat. Milchwissenschaft 1992, 47, 495–498.
- Wright, A. J.; Batte, H. D.; Marangoni, A. G. Effects of Canola Oil Dilution on Anhydrous Milkfat Crystallization and Fractionation Behavior. J. Dairy Sci. 2005, 88, 1955–1965.
- Ten Grotenhuis, E.; Van Aken, G. A.; Van Malssen, K. F.; Schenk, H. Polymorphism of Milkfat Studied by Differential Scanning Calorimetry and Real-Time X-Ray Powder Diffraction. J. Am. Oil Chem. Soc. 1999, 76, 1031–1039.
- Van Aken, G. A.; Ten Grotenhuis, E.; Van Langevelde, A. J.; Schenk, H. Composition and Crystallization of Milkfat Fractions. J. Am. Oil Chem. Soc. 1999, 76, 1323–1331.
- Kaylegian, K. E. The Production of Specialty Milkfat Fractions. J. Dairy Sci. 1999, 82, 1433–1439.
- Dewettinck, K.; Vanhoutte, B.; Foubert, I.; Huyghebaert, A. Effect of Process Parameters on Milkfat Fractionation. Idf 2004, 389, 97–103.
- Lopez, C.; Bourgaux, C.; Lesieur, P.; Riaublanc, A.; Ollivon, M. Milkfat and Primary Fractions Obtained by Dry Fractionation 1. Chemical Composition and Crystallisation Properties. Chem. Phys. Lipids 2006, 144, 17–33.
- Campos, R. J.; Litwinenko, J. W.; Marangoni, A. G. Fractionation of Milkfat by Short-Path Distillation. J. Dairy Sci. 2003, 86, 735–745.
- Zaidul, I. S. M.; Norulaini, N. N. A.; Omar, A. K. M.; Smith, R. L., Jr. Blending of Supercritical Carbon Dioxide (SC–CO2) Extracted Palm Kernel Oil Fractions and Palm Oil to Obtain Cocoa Butter Replacers. J. Food Eng. 2007, 78, 1397–1409.
- Sahena, F.; Zaidul, I. S. M.; Jinap, S.; Karim, A. A.; Abbas, K. A.; Norulaini, N. A. N.; Omar, A. K. M. Application of Supercritical CO2 in Lipid Extraction: A Review. J. Food Eng. 2009, 95, 240–253.
- Saldana, M. D. A.; Mohamed, R. S.; Baer, M. G. G.; Mazzafera, P. Extraction of Purine Alkaloids from Mate (Ilex Paraguariensis) Using Supercritical CO2. J. Agric. Food Chem. 1999, 47, 3804–3808.
- Froning, G. W.; Wehling, R. L.; Cuppett, S. L.; Niemann, L. Moisture Content and Particle Size of Dehydrated Egg Yolk Affect Lipid and Cholesterol Extraction Using Supercritical Carbon Dioxide. Poult. Sci. 1998, 77, 1718–1722.
- Ollanketo, M.; Hartonen, K.; Riekkola, M. L.; Holm, Y.; Hiltunen, R. Supercritical Carbon Dioxide Extraction of Lycopene in Tomato Skins. Eur. Food Res. Technol. 2001, 21, 561–565.
- Choksi, P. M.; Joshi, V. Y. A Review on Lycopene - Extraction, Purification, Stability and Applications. Int. J. Food Prop. 2007, 10, 289–298.
- Delfanian, M.; Esmaeilzadeh, K. R.; Sahari, M. A. Utilization of Jujube Fruit (Ziziphus Mauritiana Lam.) Extracts as Natural Antioxidants in Stability of Frying Oil. Int. J. Food Prop. 2016, 19, 789–801.
- Delfanian, M.; Esmaeilzadeh, K. R.; Sahari, M. A. Effect of Natural Extracted Antioxidants from Eriobotrya Japonica (Lindl.) Fruit Skin on Thermo Oxidative Stability of Soybean Oil during Deep Frying. Int. J. Food Prop. 2016, 19, 958–973.
- Mohamed, R. S.; Neves, G. B. M.; Kieckbusch, T. G. Reduction in Cholesterol and Fractionation of Butter Oil Using Supercritical CO2 with Adsorption on Alumina. Int. J. Food Sci. Technol. 1998, 33, 445–454.
- Hartel, R. W.; Kaylegian, K. E. Advances in Milkfat Fraction. In Crystallization Processes in Fats and Lipids Systems; Garti, N., Sato, K., Eds.; Marcel Dekker: New York, NY, 2001.
- Chen, H.; Schwartz, S. J.; Spanos, G. A. Fractionation of Butter Oil by Supercritical Carbon Dioxide. J. Dairy Sci. 1992, 75, 2659–2669.
- Fatouh, A. E.; Mahrana, G. A.; El-Ghandoura, M. A.; Singh, R. K. Fractionation of Buffalo Butter Oil by Supercritical Carbon Dioxide. LWT-Food Sci. Technol. 2007, 40, 1687–1693.
- Walstra, P.; Kloek, W.; Van Vliet, T. Fat Crystals Networks. In Crystallization Processes in Fats and Lipids Systems; Garti, N., Sato, K., Eds; Marcel Dekker: New York, NY, 2001.
- Dibildox-Alvarado, E.; Laredo, T.; Toro-Vasquez, J. F.; Marangoni, A. G. Pre-Nucleation Structuring of TAG Melts Revealed by Fluorescence Polarization Spectroscopy and Molecular Mechanics Simulations. J. Am. Oil Chem. Soc. 2010, 87, 1115−1125.
- Sonwai, S.; Mackley, M. R. The Effect of Shear on the Crystallization of Cocoa Butter. J. Am. Oil Chem. Soc. 2006, 83, 583–596.
- De Graef, V.; Goderis, B.; Van Puyvelde, P.; Foubert, I.; Dewettinck, K. Development of a Rheological Method to Characterize Palm Oil Crystallizing under Shear. Eur. J. Lipid Sci. Technol. 2008, 110, 521–529.
- MacMillan, S. D.; Roberts, K. J. In Situ Small Angle X-Ray Scattering (SAXS) Studies of Polymorphism with the Associated Crystallization of Cocoa Butter Fat Using Shearing Conditions. Cryst. Growth Des. 2002, 2, 221−226.
- Wright, A. J.; Scanlon, M. G.; Hartel, R. W.; Marangoni, A. G. Rheological Properties of Milkfat and Butter. J. Food Sci. 2001, 66, 1056–1071.
- Rønholt, S.; Mortensen, K.; Knudsen, J. C. The Effective Factors on the Structure of Butter and Other Milk Fat-Based Products. Compr. Rev. Food Sci. Food Saf. 2013, 12, 468–482.
- Kaya, A. Properties and Stability of Butter Oil Obtained from Milk and Yogurt. Nahrung-Food 2000, 44, 126–129.
- Anonymous. Column Selection for the Analysis of Fatty Acid Methyl Esters. Agilent Application Catalogue 2008, 5989-3760 EN. www.agilent.com
- Yoon, J.; Hartel, R. W.; Wang, Y. C. Analysis of Butterfat Extraction and Fractionation Using Supercritical Carbon Dioxide. J. Food Process. Pres. 1993, 17, 471–484.
- Saldana, M. D. A.; Sun, L.; Guigard, S. E.; Temelli, F. Comparison of the Solubility of β-carotene in Supercritical CO2 Based on a Binary and a Multicomponent Complex System. J. Supercrit. Fluids 2006, 37, 342–349.
- Uquiche, E.; Del Valle, J. M.; Ortiz, J. Supercritical Carbon Dioxide Extraction of Red Pepper (Capsicum Annuum L.) Oleoresin. J. Food Eng. 2004, 65, 55–66.
- Arul, J.; Tardif, R.; Boudreau, A.; McGinnis, D. S.; Lencki, R. W. Solubility of Milkfat Triglycerides in Supercritical Carbon Dioxide. Food Res. Int. 1994, 27, 459–467.
- Shi, Y.; Smith, C. M.; Hartel, R. W. Compositional Effects on Milkfat Crystallization. J. Dairy Sci. 2001, 84, 2392–2401.
- Tran, T.; Ghosh, S.; Rousseau, D. Spheroidal Fat Crystal Microstructures Formed with Confined Gap Shearing. Cryst. Growth Des. 2014, 14, 6383−6390.
- De Graef, V.; Van Puyvelde, P.; Goderis, B.; Dewettinck, K. Influence of Shear Flow on Polymorphic Behavior and Microstructural Development during Palm Oil Crystallization. Eur. J. Lipid Sci. Technol. 2009, 111, 290−302.
- Maleky, F.; Marangoni, A. Thermal and Mechanical Properties of Cocoa Butter Crystallized under an External Laminar Shear Field. Cryst. Growth Des. 2011, 11, 2429–2437.
- Mazzanti, G.; Guthrie, S. E.; Sirota, E. B.; Marangoni, A. G.; Idziak, S. H. J. Orientation and Phase Transitions of Fat Crystals under Shear. Cryst. Growth Des. 2003, 3, 721–725.
- Mazzanti, G.; Marangoni, A. G.; Idziak, S. H. J. Modeling Phase Transitions during the Crystallization of a Multicomponent Fat under Shear. Phys. Rev. E 2005, 71, 041607 (1–12).
- Mudge, E.; Mazzanti, G. Rheo-NMR Measurements of Cocoa Butter Crystallized under Shear Flow. Cryst. Growth Des. 2009, 3111–3118.