ABSTRACT
Lycopus lucidus root, harvested from two sites at three different times, was analysed for nutritional profiles; phenolics by HPLC-MS and NMR; enzymatic activity of phenylalanine ammonia-lyase (PAL), tyrosine aminotransferase (TAT), cinnamic acid 4-hydroxylase (C4H), and polyphenol oxidase (PPO); and DNA damage protective effect. The results showed that the moisture first increased and then decreased, and the crude fat and crude fibre increased continuously during the harvesting period. Sucrose and fructose were the predominant sugars with different changing patterns. Linolelaidic, linoleic, palmitic, and stearic acids were the predominant fatty acids. No significant difference was observed of vitamin B2 accompany with an increase of vitamin C with the harvest time delayed among the three harvest times investigated. Caffeic, rosmarinic, and protocatechuic acid; rosmarinic acid methylester; protocatechualdehyde; Danshensu; 3, 4-dihydroxyphenyl caffeate; and N-trans-feruloyl tyramine were identified in L. lucidus root and their variations were discrepant in the period of harvest. There was no significant correlation between phenolics and enzymatic activity except for insoluble cell-wall-bound phenolics (ICP) and C4H, as well as protocatechualdehyde and TAT. The FP exhibited higher DNA damage protective activity than ICP.
Introduction
Lycopus lucidus Turcz. (L. lucidus) is an herbaceous perennial species of the Lamiaceae family that grows on lakesides and riversides of East Asia and North America.[Citation1] In China, it grows mainly in Yunnan, Sichuan, Hebei, Liaoning, Shandong, and Guizhou provinces. L. lucidus has been used as a traditional medicine in eastern Asian countries for the treatment of disorders of menstruation, amenorrhea, menstrual cramps, cardiovascular diseases, inflammation, oedema, bellyache, traumatic injury, rheumatic arthritis, and thyroid.[Citation2–Citation4]
A few pharmacological researches have demonstrated that L. lucidus has versatile biological activities, including antioxidant activity, antimicrobial activity, anticancer,[Citation3–Citation6] inhibition of high glucose induced vascular inflammation,[Citation7] hypoglycemic and hypolipidemic effect,[Citation8] immune activity,[Citation9,Citation10] anti-allergic effect,[Citation11] and acaricidal activity.[Citation12] More importantly, the aqueous extract of L. lucidus can ameliorate streptozotocin-induced diabetic renal damage.[Citation13]
Previous phytochemical investigations of L. lucidus mostly focused on the aerial parts or the whole plant, which have been resulted in the isolation of several phenolic compounds,[Citation1,Citation3,Citation4,Citation6] flavonoids,[Citation3,Citation14,Citation15] and the raffinose family oligosaccharides.[Citation16] However, there are only a few reports on the root of L. lucidus, especially about the variations in nutrients and phytochemicals of L. lucidus root at different harvest times showing a limited information. The root, as an edible and medicinal part of L. lucidus, is a rich source of nutrients such as carotenoids, carbohydrates, vitamins, and minerals. In recent years, it has become popular and has been widely consumed in China as a typical plateau food due to its high nutritional value and potential biological functions.
Numerous pre- and post-harvest factors including genotype, climatic conditions, cultural practices, harvesting time, storage conditions, and processing procedures influence and are responsible for the wide variations in nutritional composition and phytochemicals of plant foods.[Citation17] Due to its special biological characteristics, the L. lucidus root can be harvested from November of the first year to January of the next year, during which great changes in phytochemicals and biological activities might occur because of plant development and climate change.
In the present study, we focus on the sugars, fatty acid composition, main water soluble vitamins, DNA damage protective effect and the relation of phenolic compounds to enzymatic activities of the phenylalanine ammonia-lyase (PAL), tyrosine aminotransferase (TAT), cinnamic acid 4-hydroxylase (C4H), and polyphenol oxidase (PPO) in L. lucidus root during harvesting period. In addition, DNA damage protective effect of L. lucidus root collected at different times was also investigated. Thus, we expect to provide some parameters which can contribute to harvest L. lucidus root in time according to the needs of various foods, and which would greatly facilitate production of nutraceuticals that enable consumers to gain greater access to the health benefits of L. lucidus root.
Materials and methods
Chemicals
Thiamine, riboflavin, pyridoxine, pyridoxal, pyridoxiamine, ascorbic acid, rosmarinic acid, protocatechuic acid, caffeic acid, fatty acids, pBR322 plasmid DNA, and 2,2ʹ-Azobis(2-amidinopropanehydrochloride) (AAPH) were purchased from Sigma Chemical Co. (USA). A sample of 6-hydroxy-2,5,7,8-tetramethylchroman-2-carboxylic acid (Trolox) was obtained from Sigma-Aldrich Chemical Co. (USA). All other chemicals and reagents used in the experiments were of analytical grade.
Plant material
L. Lucidus roots at maturation stage were harvested from Silian village (S1) with the annual average temperature at 15°C, the average annual rainfall of 600 mm, and a yellow brown soil (pH 5–6) and Jiangwei village (S2) with the annual average temperature at 16°C, the average annual rainfall of 700 mm and a dark brown forest soil (pH 5–6) in Jianchuan County (Yunnan, China; latitude, 26° 53ʹ N; longitude, 99° 90ʹ E; altitude, 2200 m) on November 28, 2015 (T1); December 27, 2015 (T2);and January 27, 2016 (T3). The samples were freeze-dried for the determination of nutritional profiles. L. lucidus roots harvested from Silian (S1) and Jiangwei (S2) villages in Jianchuan County on November 16, 2016 (T1); December 15, 2016 (T2); and January 14, 2017 (T3) were immediately kept in liquid N2 for the determination of enzymatic activities and freeze-dried for the determination of phenolics.
Analysis of moisture content and crude fibre
Moisture content of freshly harvested L. lucidus root was determined using an oven-dry method. Crude fibre was determined according to the method of Chinese standard GB/T 5009.10–2003.[Citation18]
Analysis of total sugar, fructose, sucrose, and glucose
The content of total sugar was measured by Fehling reagent. Fructose, sucrose, and glucose were analysed as described previously[Citation19] with some minor modifications. Two grams of L. lucidus root powder was dissolved in 50 mL distilled water, and 5 ml 1.2 mol/L Zn(CH3COO)2 and 0.25 mol/L K4[Fe(CN)6] were slowly added, respectively. The total volume was then adjusted to 100 mL with distilled water. The supernatant was filtered through a Millipore membrane (0.45 μm) and used for HPLC analysis. A chromatographic separation of sugars involved acetonitrile: water (85: 15, v/v) as the mobile phase at a flow rate of 1.0 ml/min with Waters (c) Prevail Carbohydrate ES (5 μm, 250 mm × 4.6 mm) column. The injection volume was 20 μl and the column thermostat was set at 40°C. Eluted peaks were detected with a refractive index detector. Results for the sugars were expressed as gram per 100 g dry weight (DW).
Analysis of crude fat and fatty acid composition
Crude fat content was determined by Soxhlet extractor method. L. lucidus root oil was extracted three times (each extraction for 30 min) with mineral ether assisted by ultrasonic at room temperature, and methyl esters of oil samples were prepared according to the method of Sarin, Sharma, and Khan.[Citation20] The fatty acid composition was analysed using an Agilent 7890A-5977A (Agilent, California, USA) gas chromatography–mass spectrometry (GC–MS) system equipped with a MDS detector and a fused silica capillary Agilent Technology HP-5ms column (30 m × 250 μm × 0.25 μm). Peaks of fatty acid methyl esters were identified by comparing their retention time and mass spectra with that of the known standards, and then the quantitative data were calculated from their linear calibration curves of authentic standards under analysis conditions. Results for the fatty acids were expressed as milligram per gram oil.
Analysis of main water soluble vitamins
Vitamin B1, B2, B6, and C were determined by reference to the method of Chinese standard GB 5413.11–2010, GB 5413.12–2010, GB 5413.13–2010, and SN/T 0744–1999,[Citation21–Citation24] respectively. The vitamin contents were expressed as milligram per 100 gram DW.
Analysis of phenolics
Phenolic Content: Free (FP) and insoluble cell-wall-bound phenolics (ICP) were extracted from the L. lucidus roots. Extraction was based on a procedure as described previously.[Citation25] The phenolic content was determined according to the Folin–Ciocalteu colorimetric method as described by Lu et al.[Citation6] The analysis of phenolic composition was carried out using an Agilent1200 HPLC chromatograph system (Agilent, California, USA) and interfaced with an Agilent 1100 LC/MSD Ion Trap (Agilent Technologies) mass spectrometry (MS) with an electrospray interface (ESI). Separation was performed using a reversed-phase C18 column (Agilent, ZORBAX SB-C18, 5 μm, 4.6 mm × 250 mm). The prepared phenolic extracts were filtered through a Millipore membrane (0.45 μm) before injection. The mobile phase flow was split before the MS in order to give an effective flow of 0.3 mL/min to the MS. The MS was performed to operate in mode scanning from m/z 100 to 850. Mass spectra were acquired in negative or positive mode with source voltage of 4.0 kV, capillary temperature of 350°C, and capillary voltage of −85.5 V. Nitrogen was used as the drying gas at 8 L/min and 365°C.
NMR experiment
Compounds 2 and 3 were obtained by collecting the gradient elution according to the retention time and dissolved in CD3COCD3 and CD3OD, respectively, for the determination of 1H and 13C NMR. NMR spectra were recorded on a Bruker Avance III-400 spectrometer (Bruker BioSpin GmbH, Beijing, China) and tetramethylsilane (TMS) was used as an internal standard. The chemical shifts in the NMR spectra were recorded as δ values. All of the NMR data were reported in the article were derived from 1H NMR, 13C NMR, HMQC, COSY, and HMBC experiments.
Determination of PAL, TAT, C4H, and PPO activity
The enzymatic activity of phenylalanine ammonia-lyase (PAL), tyrosine aminotransferase (TAT), and cinnamic acid 4-hydroxylase (C4H) was measured as described by Zhang et al.[Citation26] with slight modifications. For the determination of PAL, fresh L. lucidus root (0.3 g) were homogenized with 5 mL of cold extraction buffer [0.05 mol/L sodium borate buffer (pH 8.8), containing 5 mmol/L of β-mercaptoethanol, 1 mmol/L of EDTA-Na2, 5% of glycerol (v/v) and 5% of polyvinylpyrrolidone (PVP) (w/v)]. The homogenate was filtrated and the supernatant was used as a crude enzyme. The reaction mixture consisted of 0.2 mL of the crude enzyme, 2 mL of sodium borate buffer (pH 8.8), 1 mL of L-phenylalanine (0.02 mol/L), and 1 mL of distilled water. After incubation at 37°C for 60 min, 0.2 mL of HCl (6 mol/L) was added to the mixture to stop the reaction. Then the absorbance value of the sample was measured at 290 nm.
For the determination of TAT, the reaction mixture consisted of 0.3 mL of enzyme extract, 3 mL of potassium phosphate buffer (0.2 mol/L, pH 7.2), 0.2 mL of α-ketoglutarate (10 mmol/L), 0.2 mL of L-tyrosine (88 mmol/L), and 0.1 mL of pyridoxal phosphate (0.2 mmol/L). After incubation at 37°C for 30 min, 1 ml of NaOH (10 mol/L) was added to the reaction mixture to stop the reaction. Then the absorbance value of the sample was measured at 331 nm.
For the determination of C4H, the reaction mixture consisted of 0.3 mL of enzyme extract, 0.2 mL of cinnamic acid (50 mmol/L), 0.2 mL of NADPH (0.25 mg/mL), and 3 mL of potassium phosphate buffer (0.1 mol/L, pH 7.2). Then the reaction mixture was incubated for 30 min at 30°C. The reaction was stopped with 0.2 mL of HCl (6 mol/L) and readjusted to pH 11 with NaOH (6 mol/L). Absorbance value of the sample was measured at 340 nm.
For the determination of PPO, L. lucidus root (0.3 g) were homogenized with 5 mL of cold phosphate buffer (0.2 mol/L, pH 6.0), and then centrifuged at 3000 rpm for 10 min at 4°C. The supernatant was used as a crude enzyme extract. The reaction mixture consisted of 0.2 mL of enzyme extract, 2 mL of phosphate buffer (0.2 mol/L, pH 6.0), and 1 mL of pyrocatechol (10 mmol/L). The mixture was incubated at 30°C for 30 min. Absorbance value of the sample was measured at 410 nm. The enzymatic activity was expressed as unit per gram fresh weight (FW) per hour.
Determination of DNA damage protective effect
Peroxyl radical-induced plasmid DNA relaxation assay was performed according to the method of Chang et al.[Citation27] with some modifications. Trolox (100 μg/mL) was used as positive control.
Statistical analysis
All the experiments were performed in triplicates and the results were expressed as mean ± standard deviation (SD). Analysis of variance (ANOVA), Tukey’s multiple range test and t-test were carried out to determine significant differences (p < 0.05) between the means by SPSS (version 17.0). Correlation coefficient and regression analysis were determined by SPSS (version 17.0) Pearson correlation program.
Results and discussion
Nutritional profiles at different harvest times
Nutritional profiles in L. lucidus root at different harvest times are presented in . The moisture content first increased and then decreased regardless of the harvest site and a significant difference was observed between T2 and T3 roots collected in S2. Additionally, the moisture content in S1 root was lower than that in S2 root. This difference could be attributed to the divergence in water content in the soil. The crude fibre content increased continuously to the highest concentration by the end of harvest regardless of the harvest site. Crude fibre mainly occurs in cell wall of plants. Therefore, the variation of crude fibre is probably related to the synthesis of cell wall during the development of L. lucidus root growing processes. Contrary to moisture content, the crude fibre content in S1 root was higher than that in S2 root, which could be due to the divergence in field management, irrigation, and fertilization.
Table 1. Nutritional profiles and phenolic content in L. lucidus root at different harvest times.
The changes of total sugar content were different between S1 and S2 L. lucidus roots at different harvest times. No significant change was found in S1 L. lucidus root with the harvested time delayed, whereas it first decreased by 13.69% and then increased by 2.30% for S2 L. lucidus root. Meanwhile, the data indicated that the content of total sugar in S1 L. lucidus root was higher than that in S2 L. lucidus root. Fructose, sucrose, and glucose were also analysed for L. lucidus root at different harvest times. The results revealed that sucrose was the most abundant sugar, followed by fructose and glucose was not detected. The sucrose and fructose contents followed different changing patterns during the harvesting period. For S1 L. lucidus root, no significant variation of sucrose content was observed with the harvested time delayed, whereas fructose content increased significantly to 2.54-fold in T3 root compared with that in T1 root. For S2 L. lucidus root, the contents of sucrose and fructose decreased significantly by 14.26% and 47.89% from T1 to T2, respectively, and then no obvious changes were observed by the end of harvest. Additionally, the sucrose content of S1 root was higher than that of S2 root.
Sugars play an important role by providing energy for metabolic changes and the accumulation of which in the plant is determined by the balance of biosynthesis, transportation, and storage. It has been reported that various factors such as soil moisture, combined drought and heat stress, origin, harvesting date, cultivar, ripening stages, photoperiod, growth temperature, cold acclimatization, and hot air treatment could influence the sugars in plant materials.[Citation28–Citation32] Variations of total sugar, fructose, and sucrose in L. lucidus root affirmed the influence of harvest time, growing conditions, cultivation techniques, and origin on production and release of sugars. It was worth mentioning that germination occurred in L. lucidus root during the later period of harvest. It is known that germination could consume the nutritional components, especially of sugars in the rhizome of plant materials for the growth and development of sprouts due to a series of chemical and enzymatic alterations. The significant decline of total sugar, fructose and sucrose from T1 to T2 in L. lucidus root collected in S2 might be closely related to more germination.
The content of crude fat increased continuously by 16.84% and 10.56% to the highest concentration by the end of harvest for L. lucidus root collected in S1 and S2, respectively (), which was in consistent with the previous studies that as the fruit and vegetable developed, the lipid content increases.[Citation33,Citation34] Besides, it was found that the crude fat content in L. lucidus root collected in S1 was significantly higher than that in L. lucidus root collected in S2.
The fatty acid compositions of L. lucidus root oil at different harvest times are presented in . A total of 33 different fatty acids including 15 saturated fatty acids, 8 monounsaturated fatty acids, and 10 polyunsaturated fatty acids were investigated in L. lucidus root oil. The changes of total fatty acids are different between S1 and S2 L. lucidus roots during the period of harvest. It decreased continuously by 14.25% to the lowest concentration by the end of harvest for S1 L. lucidus root. In contrast, it increased continuously by 50.80% to the highest concentration by the end of harvest for S2 L. lucidus root. Thirteen saturated fatty acids were detected and palmitic and stearic acids were the major saturated fatty acids. Six monounsaturated fatty acids were detected and tetracosenoic, heptadecenoic, and 11-Eicosenoic acids were the major monounsaturated fatty acids. Nine polyunsaturated fatty acids were detected and linolelaidic and linoleic acid were the major polyunsaturated fatty acids. As a whole, the variation tendency of the mostly fatty acids paralleled the changes in total fatty acids. Differences in individual fatty acid content between S1 and S2 root were also found. Generally speaking, the content of individual saturated and monounsaturated fatty acid in S2 root was found to be significantly higher than that in S1 root collected at T3, and the content of individual polyunsaturated fatty acid in S2 root was significantly higher than that in S1 root during the harvesting period. Fatty acid composition is the characteristic feature by which the quality and possible uses of a given oil can be directly delivered.[Citation33] Shifts in membrane fatty acid composition are a way for plants to protect membrane stability, integrity, and function by increasing the proportion of unsaturated fatty acids.[Citation35] Lipid biosynthesis can be affected by environmental factors. The general categories of environmental factors affecting lipid levels and metabolism are light; temperature; water stress; soil constituents; atmospheric constituents; and other factors, such as physical damage and pest attack.[Citation36] In view of this, the significant variations in the crude fat content and fatty acid compositions in L. lucidus root collected from different sites during the harvesting period were the result of interaction of various factors.
Table 2. Fatty acid composition (mg/g oil) of L. lucidus root oil at different harvest times.
It was also interesting to note that the changing pattern of the total fatty acids was contrary to the crude fat in L. lucidus root collected in S1 during the harvesting period, indicating that unidentified compounds including triglycerides and phytosterols has made a significant contribution to the variation of crude fat. In addition, higher amounts of linoleic acid were detected in L. lucidus root oil, which makes the oil with high nutritive value.
Four water soluble vitamins including vitamin B1, B2, B6, and C were determined in L. lucidus root at different harvest times (). The obtained results revealed that vitamin B1 and B6 were not detected in L. lucidus root. However, L. lucidus root contained high concentrations of vitamin B2 and vitamin C. No significant difference of vitamin B2 content was observed during the harvesting period in both S1 and S2 L. lucidus roots. The vitamin C content increased by 23.08% to the highest concentration at T2 and no obvious changes were observed from T2 to T3 for S1 L. lucidus root, and it increased continuously to 2.34-fold to the highest concentration by the end of harvest for S2 L. lucidus root. Additionally, no significant differences of vitamin B2 contents were observed between S1 and S2 roots. However, there were significant differences of vitamin C contents between S1 and S2 roots at each harvest time. That is, in S1 root it was significantly higher than that in S2 at both T1 and T2, while at T3 it was significantly lower than that in S2.
Unapparent variation of vitamin B2 content in L. lucidus root at different harvest times indicated that the biosynthesis of this vitamin was slightly affected by the growing conditions and developmental stage. The ideal low temperature acclimatization period increases the ascorbic acid content in plant foods.[Citation37] It has been reported that low temperature and cold stress increased the vitamin C content in broccoli and spinach.[Citation19,Citation38] The significant increase of vitamin C content in L. lucidus root with the harvest time delayed could be attributed to the decrease of atmospheric temperature. L. lucidus root can well tolerate low temperature through a profound modification of its root metabolism, specifically related to its capacity to scavenge oxygen relative species (antioxidant activities), which results in a relevant rise in its ascorbic acid content in the root.
NMR data
N-trans-Feruloyl tyramine: 1H-NMR (CD3COCD3, 400 MHz) δ:7.51 (1H, d, J = 15.8 Hz, H-7), 7.44 (1H, t, J = 5.5 Hz, CONH), 7.14 (1H, d, J = 1.7 Hz, H-2), 7.06 (2H, d, J = 8.3 Hz, H-2ʹ, 6ʹ), 7.04 (1H, dd, J = 8.4, 1.7 Hz, H-6), 6.85 (1H, d, J = 8.4 Hz, H-5), 6.78 (1H, dd, J = 7.4 Hz, J = 1.8 Hz, H-5ʹ), 6.55 (1H, d, J = 15.8 Hz, H-8), 3.52 (2H, m, H-8ʹ), 2.77 (2H, t, J = 7.5 Hz, H-7ʹ), 3.85 (3H, s, OCH3); 13C-NMR (CD3COCD3, 100 MHz) δ:127.8 (C-1), 111.1 (C-2), 148.3 (C-3), 148.9 (C-4), 115.9 (C-5), 122.3 (C-6), 140.5 (C-7), 119.4 (C-8), 166.7 (C-9),130.6 (C-1ʹ), 130.2 (C-2ʹ, 6ʹ), 115.8 (C-3ʹ, 5ʹ), 156.5 (C-4ʹ), 35.4 (C-7ʹ), 47.8 (C-8ʹ), 55.9 (OCH3).
3,4-Dihydroxyphenyl caffeate: 1H-NMR (CD3OD, 400 MHz) δ:7.06 (1H, d, s, H-2), 6.79 (1H, d, J = 7.8 Hz, H-5), 6.93 (1H, d, J = 7.7 Hz, H-6), 7.54 (1H, d, J = 15.3 Hz, H-7), 6.29 (1H, d, J = 15.3 Hz, H-8), 6.80 (1H, s, H-2ʹ), 6.71 (1H, d, J = 7.8 Hz, H-5ʹ), 6.65 (1H, m, H-6ʹ); 13C-NMR (CD3OD, 100 MHz) δ:127.8 (C-1), 115.2 (C-2), 146.6 (C-3), 149.4 (C-4), 116.5 (C-5), 123.0 (C-6), 147.1 (C-7), 115.1 (C-8), 168.9 (C-9), 129.4 (C-1ʹ), 117.5 (C-2ʹ), 144.9 (C-3ʹ), 145.9 (C-4ʹ), 116.2 (C-5ʹ), 121.8 (C-6ʹ).
Phenolics at different harvest times
The levels of FP, ICP, and total phenolic content (TPC) in L. lucidus root at different harvest times are presented in . The FP content first decreased by 9.81% and 16.30% to the lowest level at T2 and then increased by 2.31% and 15.41% for S1 and S2 L. lucidus root, respectively. The ICP content first increased by 1.63-fold to the highest level at T2 and then decreased by 39.24% for the S1 L. lucidus root. However, it decreased continuously by 48.41% and reached a minimum at T3 in S2 L. lucidus root. No significant changes were observed of the TPC in S1 L. lucidus root during the harvesting period, while it first decreased by 17.12% and then increased by 10.42% in S2 L. lucidus root. In addition, the TPC of S1 L. lucidus root was higher than that in S2 L. lucidus root.
In the present study, the phenolic compounds were identified by comparing peak retention time, ESI-MS and NMR spectroscopic data with standard compounds or published data in the literature and the results are shown in and . Comparing with the retention time, UV and mass data of the authentic standard compounds, peak 1, 4 and 6 were identified as caffeic, rosmarinic, and protocatechuic acid, respectively. Comparing with the mass data in the literature,[Citation39–Citation41] peak 5, 7, and 8 were identified as rosmarinic acid methylester, protocatechualdehyde and Danshensu. The molecular formula of peak 2 was determined to be C18H19NO4 based on the 1H and 13C NMR and ESI-MS data. The 13C NMR spectrum and DEPT revealed the presence of 18 carbon signal including one CH3, two CH2, nine CH and six C. The 1H NMR spectrum revealed the presence of two olefinic protons at δ 7.51 (1H, d, J = 15.8 Hz) and 6.55 (1H, d, J = 15.8 Hz), one tri-substituted aromatic ring with signals at δ 7.14 (1H, d, J = 1.7 Hz), 7.04 (1H, dd, J = 8.4, 1.7 Hz), and 6.85 (1H, d, J = 8.4 Hz), and one di-substituted aromatic ring with signals at δ 7.06 (2H, d, J = 8.3 Hz) and 6.78 (2H, d, J = 8.5 Hz). The coupling constants between H-7 and H-8 (J = 15.8 Hz) clearly indicated the trans-conFiguretion of the double bonds. Key correlations from H-7 (δ 7.51, 1H, d, 15.8 Hz) to C-2 (δ 111.1), C-6 (δ 122.3), and C-9 (δ 166.7), from H-7ʹ (δ 2.77, 2H, t, 7.5 Hz) to C-2ʹ (δ 130.2) and C-6ʹ (δ 130.2), from H-8ʹ (δ 3.52, 2H, m) to C-9 (δ 166.7), from CONH (δ 7.44, 1H, t, 5.5 Hz) to C-8 (δ 119.4) and C-7ʹ (δ 35.4), from OCH3 (δ 3.85, 3H, s) to OCH3 (δ 3.85) were observed in the HMBC experiments. Additionally, key correlations from H-8ʹ (δ 3.52, 2H, m) to CONH (δ 7.44, 1H, t, 5.5 Hz) and H-7ʹ (δ 2.77, 2H, t, 7.5 Hz) were observed in the 1H-1HCOSY experiments. With a comparison of the spectroscopic data available in the literature,[Citation42] peak 2 was consequently identified as N-trans-feruloyl tyramine. The molecular formula of compound 3 was determined to be C15H12O6 based on the 1H and 13C NMR and ESI–MS data. The NMR spectra of compound 3 exhibited 15 carbons and 8 aromatic protons, of which 9 carbons and 5 protons were assigned to the caffeic acid unit and the remaining 6 carbons and 3 protons to an oxygenation-aromatic ring. HMQC and HMBC correlations established the 1ʹ, 3ʹ, 4ʹ- oxygenation patterns of the ring. The linkage between the caffeic acid unit and the ring was deduced from the downfield shifts of δ 168.9 (C-9) and δ 129.4 (C-1ʹ). The full assignments of the protons and carbons were achieved by a combination of 1H, 13C, COSY, HMQC, and HMBC experiments. The structure of peak 3 was determined as 3, 4-dihydroxyphenyl caffeate.[Citation43]
Table 3. Mass spectrum data of the identified phenolic compounds in the phenolic extracts from L. lucidus root at different harvest times.
Figure 1. Typical HPLC chromatogram of the FP (A) and ICP (B) from T2 L. lucidus root collected in S1. The peaks are (1) caffeic acid, (2) 3,4-Dihydroxyphenyl caffeate (3) N-feruloyl tyramine, (4) rosmarinic acid, (5) rosmarinic acid methylester, (6) protocatechuic acid, (7) protocatechualdehyde, and (8) Danshensu.
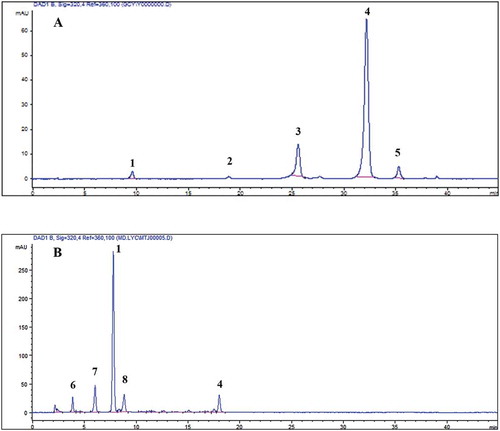
The changes in phenolic compounds in L. lucidus root during the harvesting period were investigated and the results are presented in . Rosmarinic acid, rosmarinic acid methylester, and N-trans-feruloyl tyramine were the major phenolic compounds in FP. Caffeic and rosmarinic acids were the major phenolic compounds in ICP. As can be seen from the table, the variations of the phenolic compounds in L. lucidus root during the harvesting period are different. For example, protocatechuic acid were decomposed, N-trans-feruloyl tyramine and rosmarinic acid methylester were synthesized, and caffeic acid, protocatechualdehyde and Danshensu remained stable.
Table 4. Content of the phenolic compounds (mg/g DW) in L. lucidus root at different harvest times.
The key enzyme for phenolic metabolism is PAL; it catalyses the reductive deamination of L-phenylalanine to form cinnamic acid.[Citation44] Further enzymes including C4H, 4-coumaric acid CoA –ligase (4CL), chalcone synthase (CHS), or chalcone isomerase of the starting phase of the major phenylpropanoid pathway were also involved in phenolic biosynthesis. The other key enzyme for phenolic metabolism is TAT; it catalyses the reductive deamination of L-tyrosine to form 4-hydroxyphenylpyruvic acid (pHPP), which is then reduced to 4-hydroxyphenyllactic acid (pHPL) by hydroxyphenylpyruvate reductase (HPPR).[Citation45] The two intermediate precursors of 4-coumaroyl-CoA produced in phenylpropanoid pathway and 4-hydroxyphenyllactic acid are coupled by ester formation with release of coenzyme A and 4-coumaroyl-4ʹ-hydroxyphenyllactic acid (4C-pHPL) is formed.[Citation45] The reaction is catalysed by rosmarinic acid synthase (RAS) and 4C-pHPL further transforms to rosmarinic acid by cytochrome P450-dependent monooxygenase reactions.[Citation45]
The enzymatic activity of PAL, TAT, C4H, and PPO in L. lucidus root at different harvest times is presented in . The PAL activity increased continuously by 1.05-fold and 51.86% to the maximum at T3 in S1 and S2 L. lucidus root, respectively. The TAT activity first increased by 57.88% to the highest level at T2 and then decreased by 25.99% in S1 L. lucidus root, while it increased continuously by 13.90% at T3 in S2 L. lucidus root. The C4H activity first decreased by 71.69% and 29.03% to the lowest level at T2 and then increased by 69.16% and 48.94% in S1 and S2 L. lucidus root, respectively. In contrast, the PPO activity first increased by 9.69% and 4.49-fold to the highest level at T2 and then decreased by 39.94% and 52.21% in S1 and S2 L. lucidus root, respectively. As a whole, the enzymatic activity of PAL, TAT, and C4H in L. lucidus root collected in S2 was higher than that in L. lucidus root collected in S1, whereas the PPO activity in L. lucidus root collected in S2 was lower than that in L. lucidus root collected in S1.
Figure 2. PAL, TAT, C4H, and PPO activities of L. lucidus root. Values with different lowercase letters for the same harvest site at different harvest times are statistically different at p < 0.05. Values with different uppercase letters for different harvest sites at same harvest time are statistically different at p < 0.05.
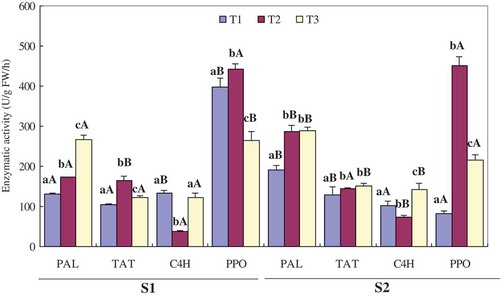
It has been reported that various factors such as irradiation, nutrient deficiencies, wounding, lower temperature stress, herbicide, and regulator treatment along with viral, fungal and insect attacks can increase PAL synthesis or PAL activity, or induce secondary metabolites through the phenylpropanoid pathway in various plants.[Citation44,Citation46] The TAT activity can also be influenced by ultraviolet irradiation, heavy metal salts, methyl jasmonate, and fungi extracts.[Citation26] Therefore, the complex changes in the enzymatic activity in L. lucidus root at different harvest times were closely related to the soil, fertilization, cultural practices, herbicide and regulator treatment, climate, growing season, insect pest and the degree of maturity at harvest. Variation in enzymatic activity further resulted in the changes of phenolic compounds in L. lucidus root during the harvesting period.
The correlation analysis between the phenolics and PAL, TAT, C4H, and PPO activity was performed and the correlation coefficients (R) are shown in . There was no significant correlation between the phenolics and the enzymatic activity except for ICP and C4H, as well as protocatechualdehyde and TAT. The enzymes of the general phenylpropanoid pathway (PAL, C4H, 4CL) are ubiquitous in land plant and represent branch points in formation of various secondary metabolites, such as flavonoids, lignin, chlorogenic acid and anthocyanin. Flux of metabolites through this pathway is diverted to numerous products. TAT is a primary enzyme as well because it forms pHPP that is needed for the biosynthesis of tocopherols and plastoquinones. PPO can oxidize phenolics to quinines. Due to their multiple roles in the secondary metabolic network, the levels of different phenolic compounds in plant materials are the result of combined action of various enzymes. Some authors reported that rosmarinic acid accumulation was correlated to the TAT activity but not PAL activity.[Citation47] While others found that rosmarinic acid accumulation was correlated to PAL activity but not TAT activity.[Citation26] In the present study, rosmarinic acid was more correlated to PAL activity. This inconsistency could be attributed to the divergence of plant materials. Additionally, no significant correlations were observed among PAL, TAT, C4H, and PPO activity ().
Table 5. Correlation analysis of phenolics and enzymatic activities.
DNA damage protective activity at different harvest times
The efficiency of phenolic extracts (100 μg GAE/mL) from L. lucidus root in preventing oxidative damage of DNA induced by peroxyl radical was also evaluated. The plasmid DNA was mainly of the supercoiled form in the absence of AAPH) (A, lane 1). During the addition of AAPH, the supercoiled form of DNA converted into the open circular form (A, lane 2) indicating that ROO· radical generated from thermal decomposition of AAPH caused single-strand DNA breakage.
Figure 3. DNA damage protective activity of phenolic extracts from L. lucidus root harvested at different times. (A) Protective activity of phenolic extracts against ROO· radical-induced DNA damage. Lane 1, the native DNA; Lane 2, the DNA treated with AAPH; Lanes 3–5, the DNA treated with FP from T1, T2, and T3 L. lucidus root collected in S1, respectively; Lanes 6–8, the DNA treated with FP from T1, T2, and T3 L. lucidus root collected in S2, respectively; Lanes 9–11, the DNA treated with ICP from T1, T2 and T3 L. lucidus root collected in S1, respectively; Lanes 12–14, the DNA treated with ICP from T1, T2 and T3 L. lucidus root collected in S2, respectively; Lane 15, the DNA treated with Trolox. (B) Densitometric quantification of the protective activity of phenolic extracts and Trolox against ROO· radical-induced DNA damage. Data represent mean ± SD for n = 3. Different lowercase letters indicate statistically significant differences between the means (p < 0.05) for % supercoiled form.
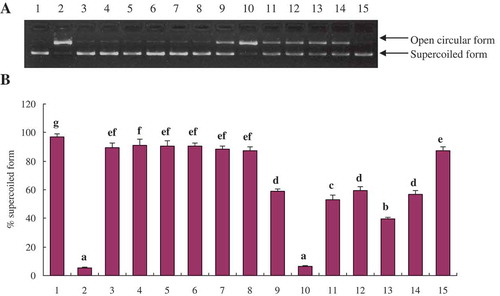
The protective activity assayed herein on ROO· radical-induced DNA damage was expressed as % supercoiled form for comparison. Differences in DNA damage protective activity among the phenolic extracts were observed (A, lanes 3–14). The % supercoiled form values for FP and ICP ranged from 87.47 to 91.00 and 6.47 to 59.30, respectively (B). These data indicated that the FP exhibited higher protective activity against ROO· radical-induced DNA strand breakage than that of ICP, which could be due to the presence of different phenolic compounds in FP and ICP. The correlation analysis between the DNA damage protective effect and the content of individual phenolic compound was performed. In FP fraction, significant positive correlation was found between caffeic acid and DNA damage protective effect (R = 0.854, p < 0.05). In ICP fraction, significant negative correlation was observed between protocatechuic acid, protocatechualdehyde, and Danshensu (R = −0.837, p < 0.05; R = −0.920, p < 0.01; and R = −0.865, p < 0.05, respectively). Phenolic compounds might contribute to DNA damage protective activity individually or through synergic effects. Besides, the unidentified phenolics and non-phenolic constituents present in the phenolic extracts may also be responsible for the DNA damage protective activity. Furthermore, the DNA damage protective activity of FP was higher than that of Trolox (% supercoiled form value 87.11).
Conclusion
In summary, the nutritional profiles, phenolics, and DNA damage protective effect of L. lucidus root were found to vary greatly with the collecting site and harvest time. Different changing patterns were observed of moisture, crude fat, crude fibre, sugars, vitamins, fatty acid composition, and phenolics during the harvesting period. The effect of harvest site on these parameters was also different. Eight phenolic compounds were identified in L. lucidus root and rosmarinic acid was the most abundant one. There was no significant correlation between phenolics and PAL, TAT, C4H, and PPO activity except for ICP and C4H, and protocatechualdehyde and TAT. The FP exhibited higher protective activity against ROO· radical-induced DNA strand breakage than that of ICP. The variations of these parameters may significantly affect the nutritional quality and processing characteristics of L. lucidus root. As a result, the L. lucidus root should be harvested at appropriate time according to the requirement of nutrition and processing while consuming L. lucidus root.
Additional information
Funding
References
- Murata, T.; Watahiki, M.; Tanaka, Y.; Miyase, T.; Yoshizaki, F. Hyaluronidase Inhibitors from Takuran, Lycopus Lucidus. Chem. Pharm. Bull. 2010, 58, 394–397. doi:10.1248/cpb.58.394.
- The State Commission of Chinese Pharmacopoeia. Pharmacopoeia of People’s Republic of China, Part Ӏ; China Medical Science Press: Beijing, 2015; 228.
- Woo, E. R.; Piao, M. S. Antioxidative Constituents from Lycopus Lucidus. Arch. Pharm. Res. 2004, 27, 173–176. doi:10.1007/BF02980102.
- Ślusarczyk, S.; Hajnos, M.; Skalicka-Woźniak, K.; Matkowski, A. Antioxidant Activity of Polyphenols from Lycopus Lucidus Turcz. Food Chem. 2009, 113, 134–138. doi:10.1016/j.foodchem.2008.07.037.
- Yu, J. Q.; Lei, J. C.; Zhang, X. Q.; Yu, H. D.; Tian, D. Z.; Liao, Z. X.; Zou, G. L. Anticancer, Antioxidant and Antimicrobial Activities of the Essential Oil of Lycopus Lucidus Turcz. Var. Hirtus Regel. Food Chem. 2011, 126, 1593–1598. doi:10.1016/j.foodchem.2010.12.027.
- Lu, Y. H.; Huang, J. H.; Li, Y. C.; Ma, T. T.; Sang, P.; Wang, W. J.; Gao, C. Y. Variation in Nutritional Compositions, Antioxidant Activity and Microstructure of Lycopus Lucidus Turcz. Root at Different Harvest Times. Food Chem. 2015, 183, 91–100. doi:10.1016/j.foodchem.2015.03.033.
- Lee, Y. J.; Kang, D. G.; Kim, J. S.; Lee, H. S. Lycopus Lucidus Inhibits High Glucose-Induced Vascular Inflammation in Human Umbilical Vein Endothelial Cells. Vascul. Pharmacol. 2008, 48, 38–46. doi:10.1016/j.vph.2007.11.004.
- Xiong, W.; Chen, G. Y.; Tan, D. Y.; Zuo, S. Y. Hypoglycemic and Hypolipidemic Effect of Polysaccharide from Wild Plant Lycopus Lucidus Turcz. On Alloxan-Induced Diabetic Mice. Chinese. J. Bioprocess Eng. 2011, 9, 45–48.
- Yang, X. B.;. Lv Y. L.; Tian, L. M.; Zhao, Y. Composition and Systemic Immune Activity of the Polysaccharides from an Herbal Tea (Lycopus Lucidus Turcz). J. Agric. Food Chem. 2010, 58, 6075–6080. doi:10.1021/jf101061y.
- Yang, X. B.; Zhao, Y.; He, N. W. Isolation, Characterization, and Immunological Effects of α-galacto-oligosaccharides from a New Source, the Herb Lycopus Lucidus Turcz. J. Agric. Food Chem. 2010, 58, 8253–8258. doi:10.1021/jf101217f.
- Shin, T. Y.; Kim, S. H.; Suk, K.; Ha, J. H.; Kim, I. K.; Lee, M. G.; Jun, C. D.; Kim, S. Y.; Lim, J. P.; Eun, J. S.; Shin, H. Y.; Kim, H. M. Anti-Allergic Effects of Lycopus Lucidus, on Mast Cell-Mediated Allergy Model. Toxicol. Appl. Pharmacol. 2005, 209, 255–262. doi:10.1016/j.taap.2005.04.011.
- Yang, J. Y.; Lee, H. S. Acaricidal Activities of the Active Component of Lycopus Lucidus Oil and Its Derivatives against House Dust and Stored Food Mites (Arachnida: Acari). Pest Manag. Sci. 2012, 68, 564–572. doi:10.1002/ps.2295.
- Yao, Y.; Yang, J.; Wang, D.; Zhou, F.; Cai, X.; Lu, W.; Hu, C.; Gu, Z.; Qian, S.; Guan, X.; Cao, P. The Aqueous Extract of Lycopus Lucidus Turcz Ameliorates Streptozotocin-Induced Diabetic Renal Damage via Inhibiting TGF-β1 Signaling Pathway. Phytomedicine 2013, 20, 1160–1167. doi:10.1016/j.phymed.2013.06.004.
- Malik, A.; Yuldashev, M. P. Flavonoids of Lycopus Lucidus. Chem. Nat. Compounds 2002, 38, 104–105. doi:10.1023/A:1015762605965.
- Malik, A.; Yuldashev, M. P.; Obid, A.; Ismoil, T.; Ping, L. Y. Flavonoids of the Aerial Part of Lycopus Lucidus. Chem. Nat. Compounds 2002, 38, 612–613. doi:10.1023/A:1022667611501.
- Liang, T.; Fu, Q.; Li, F.; Zhou, W.; Xin, H.; Wang, H.; Jin, Y.; Liang, X. Hydrophilic Interaction Liquid Chromatography for the Separation, Purification, and Quantification of Raffinose Family Oligosaccharides from Lycopus Lucidus Turcz. J. Sep. Sci. 2015, 38, 2607–2613. doi:10.1002/jssc.v38.15.
- Lee, S. K.; Kader, A. A. Pre-Harvest and Post-Harvest Factors Influencing Vitamin C Content of Horticultural Crops. Postharvest Biol. Technol. 2000, 20, 207–220. doi:10.1016/S0925-5214(00)00133-2.
- Ministry of Health, People’s Republic of China; Standardization Administration of China. Determination of Crude Fiber in Vegetable Foods. GB/T 5009.10-2003; Beijing: Standards press of China, 2003.
- Yoon, Y.; Kuppusamy, S.; Cho, K. M.; Kim, P. J.; Kwack, Y.; Lee, Y. B. Influence of Cold Stress on Contents of Soluble Sugars, Vitamin C and Free Amino Acids Including Gamma-Aminobutyric Acid (GABA) in Spinach (Spinacia Oleracea). Food Chem. 2017, 215, 185–192. doi:10.1016/j.foodchem.2016.07.167.
- Sarin, R.; Sharma, M.; Khan, A. A. Studies on Guizotia Abyssinica L. Oil, Biodiesel Synthesis and Process Optimization. Bioresour. Technol. 2009, 100, 4187–4192. doi:10.1016/j.biortech.2009.03.072.
- Ministry of Health, People’s Republic of China. National Food Safety Standard. Determination of Vitamin B1 in Foods for Infants and Young Children, Milk and Milk Products. GB 5413.11-2010; Beijing: Standards press of China, 2010.
- Ministry of Health, People’s Republic of China. National Food Safety Standard. Determination of Vitamin B2 in Foods for Infants and Young Children, Milk and Milk Products. GB 5413.12-2010; Beijing: Standards press of China, 2010.
- Ministry of Health, People’s Republic of China. National Food Safety Standard. Determination of Vitamin B6 in Foods for Infants and Young Children, Milk and Milk Products. GB 5413.13-2010; Beijing: Standards press of China, 2010.
- China Entry-Exit Inspection and Quarantine. Method for Determination of Vitamin C and Caffeine in Beverages of Export; SN/T, 0744–1999; Beijing: Standards press of China, 1999.
- Santiago, R.; Reid, L. M.; Arnason, J. T.; Zhu, X. Y.; Martinez, N.; Malvar, R. A. Phenolics in Maize Genotypes Differing Insusceptibility to Gibberella Stalk Rot (Fusarium Graminearum Schwabe). J. Agric. Food Chem. 2007, 55, 5186–5193. doi:10.1021/jf070641e.
- Zhang, S. C.; Yan, Y.; Wang, B. Q.; Liang, Z. S.; Liu, Y.; Liu, F. H.; Qi, Z. H. Selective Responses of Enzymes in the Two Parallel Pathways of Rosmarinic Acid Biosynthetic Pathway to Elicitors in Salvia Miltiorrhiza Hairy Root Cultures. J. Biosci. Bioeng. 2014, 117, 645–651. doi:10.1016/j.jbiosc.2013.10.013.
- Chang, S. T.; Wu, J. H.; Wang, S. Y.; Kang, P. L.; Yang, N. S.; Shyur, L. F. Antioxidant Activity of Extracts from Acacia Confusa Bark and Heartwood. J. Agric. Food Chem. 2001, 49, 3420–3424. doi:10.1021/jf0100907.
- Wang, B. M.; Chen, J. J.; Chen, L. S.; Wang, X. N.; Wang, R.; Ma, L.; Peng, S. F.; Luo, J.; Chen, Y. Z. Combined Drought and Heat Stress in Camellia Oleifera Cultivars: Leaf Characteristics, Soluble Sugar and Protein Contents, and Rubisco Gene Expression. Trees 2015, 29, 1483–1492. doi:10.1007/s00468-015-1229-9.
- Karimi, M.; Ahmadi, A.; Hashemi, J.; Abbasi, A.; Tavarini, S.; Guglielminetti, L.; Angelini, L. G. The Effect of Soil Moisture Depletion on Stevia (Stevia Rebaudiana Bertoni) Grown in Greenhouse Conditions: Growth, Steviol Glycosides Content, Soluble Sugars and Total Antioxidant Capacity. Sci. Hortic. 2015, 183, 93–99. doi:10.1016/j.scienta.2014.11.001.
- Hykkerud Steindal, A. L.; Rødven, R.; Hansen, E.; Mølmann, J. Effects of Photoperiod, Growth Temperature and Cold Acclimatization on Glucosinolates, Sugars and Fatty Acids in Kale. Food Chem. 2015, 174, 44–51. doi:10.1016/j.foodchem.2014.10.129.
- Yu, L. N.; Liu, H. X.; Shao, X. F.; Yu, F.; Wei, Y. Z.; Ni, Z. M.; Xu, F.; Wang, H. F. Effects of Hot Air and Methyl Jasmonate Treatment on the Metabolism of Soluble Sugars in Peach Fruit during Cold Storage. Postharvest Biol. Technol. 2016, 113, 8–16. doi:10.1016/j.postharvbio.2015.10.013.
- Yang, B. R. Sugars, Acids, Ethyl β-D-glucopyranose and a Methyl Inositol in Sea Buckthorn (Hippophaë Rhamnoides) Berries. Food Chem. 2009, 112, 89–97. doi:10.1016/j.foodchem.2008.05.042.
- Gaydou, E. M.; Lozano, Y.; Ratovohery, J. Triglyceride and Fatty Acid Compositions in the Mesocarp of Persea Americana during Fruit Development. Phytochemistry 1987, 26, 1595–1597. doi:10.1016/S0031-9422(00)82251-7.
- Ozdemir, F.; Topuz, A. Changes in Dry Matter, Oil Content and Fatty Acids Composition of Avocado during Harvesting Time and Post-Harvesting Ripening Period. Food Chem. 2004, 86, 79–83. doi:10.1016/j.foodchem.2003.08.012.
- Steponkus, P. L. Role of the Plasma Membrane in Freezing Injury and Cold Acclimation. Annu. Rev. Plant Physiol. Plant Mol. Biol. 1984, 35, 543–584. doi:10.1146/annurev.pp.35.060184.002551.
- Salas, J. J.; Sanchez, J.; Ramli, U. S.; Manaf, A. M.; Williams, M.; Harwood, J. L. Biochemistry of Lipid Metabolism in Olive and Other Oil Fruits. Prog. Lipid Res. 2000, 39, 151–180. doi:10.1016/S0163-7827(00)00003-5.
- Ito, A.; Shimizu, H.; Hiroki, R.; Nakashima, H.; Miyasaka, J.; Ohdoi, K. Effect of Different Durations of Root Area Chilling on the Nutritional Quality of Spinach. Environ. Control Biol. 2014, 51, 187–191. doi:10.2525/ecb.51.187.
- Steindal, A. L. H.; Mølmann, J.; Bengtsson, G. B.; Johansen, T. J. Influence of Day Length and Temperature on the Content of Health-Related Compounds in Broccoli (Brassica Oleracea L. Var. Italica). J. Agric. Food Chem. 2013, 61, 10779–10786. doi:10.1021/jf403466r.
- Kang, N. S.; Lee, J. H. Characterisation of Phenolic Phytochemicals and Quality Changes Related to the Harvest Times from the Leaves of Korean Purple Perilla (Perilla Frutescens). Food Chem. 2011, 124, 556–562. doi:10.1016/j.foodchem.2010.06.071.
- Song, C. Z.; Wang, Y. H.; Hua, Y.; Wu, Z. K.; Du, Z. Z. Chemical Constituents of Clematis Montana. Chin. J. Nat. Med. 2008, 6, 116–119. doi:10.3724/SP.J.1009.2008.00116.
- Zhou, W. T.; Xie, H. H.; Xu, X. Y.; Liang, Y. G.; Wei, X. Y. Phenolic Constituents from Isodon Lophanthoides Var. Graciliflorus and Their Antioxidant and Antibacterial Activities. J. Funct. Foods 2014, 6, 492–498. doi:10.1016/j.jff.2013.11.015.
- Forino, M.; Tartaglione, L.; Dell’Aversano, C.; Ciminiello, P. NMR-based Identification of the Phenolic Profile of Fruits of Lycium Barbarum (Goji Berries). Isolation and Structural Determination of a Novel N-Feruloyl Tyramine Dimer as the Most Abundant Antioxidant Polyphenol of Goji Berries. Food Chem. 2016, 194, 1254–1259. doi:10.1016/j.foodchem.2015.08.129.
- Zhang, Z. Z.; Li, S. Y.; Li Ownby, S.; Wang, P.; Yuan, W.; Zhang, W. L.; Scott Beasley, R. Phenolic Compounds and Rare Polyhydroxylated Triterpenoid Saponins from Eryngium Yuccifolium. Phytochemistry 2008, 69, 2070–2080. doi:10.1016/j.phytochem.2008.03.020.
- Morello, J. R.; Romero, M. P.; Ramo, T.; Motilva, M. J. Evaluation of L-Phenylalanine Ammonia-Lyase Activity and Phenolic Profile in Olive Drupe (Olea Europaea L.) From Fruit Setting Period to Harvesting Time. Plant Sci. 2005, 168, 65–72. doi:10.1016/j.plantsci.2004.07.013.
- Petersen, M.; Abdullah, Y.; Benner, J.; Eberle, D.; Gehlen, K.; Hücherig, S.; Janiak, V.; Kim, K. H.; Sander, M.; Weitzel, C.; Wolters, S. Evolution of Rosmarinic Acid Biosynthesis. Phytochemistry 2009, 70, 1663–1679. doi:10.1016/j.phytochem.2009.05.010.
- Hudec, J.; Kochanová, R.; Burdová, M.; Kobida, L.; Kogan, G.; Turianica, I.; Chlebo, P.; Hanáčková, E.; Slamka, P. Regulation of the Phenolic Profile of Berries Can Increase Their Antioxidant Activity. J. Agric. Food Chem. 2009, 57, 2022–2029. doi:10.1021/jf803185g.
- Yan, Q.; Shi, M.; Ng, J.; Wu, J. Y. Elicitor-Induced Rosmarinic Acid Accumulation and Secondary Metabolism Enzyme Activities in Salvia Miltiorrhiza Hairy Roots. Plant Sci. 2006, 170, 853–858. doi:10.1016/j.plantsci.2005.12.004.