ABSTRACT
Sol-gel and melt-mixing processes, which are used for the synthesis of low-density polyethylene/silver/titanium dioxide nanocomposites, can inactivate pathogens. In the sol-gel method, the nanocomposites were used to treat four selected microorganisms using the disk-diffusion method. In the melt-mixing method, fish coverage films were used to examine the shelf life of fish. TiO2 along with different concentrations of Ag (0%, 1%, 3%, and 5%) generated the nanocomposites. The maximum inhibition zone diameters (mm) for Staphylococcus aureus, Escherichia coli, Candida albicans, and Aspergillus niger in 3% Ag were measured. It was concluded that applying the LDPE/Ag/TiO2 nanocomposites produced could be beneficial for inactivation of pathogens.
Introduction
The food packaging industry has recently introduced innovations including food packaging in response to changes in food preparation and consumer demands for healthy foods [3]. Packaging is one of the largest industries in the world, comprising about 2% of the Gross National Product, especially in developed countries.[Citation1] Nanotechnology is an interdisciplinary science utilizing particles with dimensions less than 100 nm. Nanopackaging can protect food products during storage and transportation from undesirable conditions, such as bacteria growth.[Citation2] Nanopackaging along with bactericidal characteristics can be effective in increasing the shelf life of food products, ensuring food safety and consumer protection and satisfaction.[Citation3]
Inorganic compounds that restrict pathogens are usually supplemented to the polymers by melt mixing or other processes in the ultra-fine phase dimension of dispersion behavior.[Citation4] of nanoscale filler in the polymer medium. Transmission electron microscopy has been used to observe this process at high magnification.[Citation5] This approach can produce a nanocomposite that prevents the growth of pathogens and microbial colonization of polymers.[Citation6]
Among the nanocomposites, the photocatalyst, titanium dioxide (TiO2), has been effectively applied to tap water to inactivate bacteria. TiO2 as distinctive semiconductor photocatalyst can play a role as an anti-pathogenic agent. Nanoparticle (NP) compounds prepared from inorganic oxides such as TiO2 produced by the sol-gel as well as melting method have been confirmed as being bactericidal and suitable for a wide variety of uses including food packaging.[Citation7]
Low-density polyethylene (LDPE)-TiO2 nanocomposite films can be efficiently degraded under ultraviolet (UV) or sunlight radiance.[Citation8] Numerous electron-sized holes will be made on TiO2, and the OH− reactive oxygen species (ROS) and absorbed water on the exterior of TiO2 will be radicalized, while TiO2 is introduced to photons with an energy larger than the band gap of TiO2. The OH− attaches to the pathogens and inactivates them[Citation5] by degrading the organic components through the oxidization of the C–H bonds in the polyunsaturated phospholipid constituent of the bacterial cell wall [Citation9,Citation10] These three forms of ROS are released by the reaction of TiO2. TiO2-covered thin layers can reduce microbial contamination on the surface of food products.[Citation11] Mihaly Cozmuta et al.[Citation12] studied the ability of the packaging of a silver (Ag)/TiO2 nanocomposite prepared by the solvent method to increase the durability of bread. Ahari et al.[Citation13] examined the microbiological properties of TiO2 and Ag (1%, 3%, and 5%) nanocomposite coatings on dry sausages. NPs display different characteristics against microbes. CuONPs are more effective against Escherichia coli and Staphylococcus aureus, but are less effective against Bacillus subtilis.[Citation14] NPs containing TiO2 incorporates with Ag were studied using a variety of Gram-negative bacteria, Gram-positive bacteria, and fungi.[Citation15] Few studies [Citation16,Citation17] showed that the ability of Ag-NPs to discharge Ag+ is essential to inactivate bacterial activity. Yamanaka et al.[Citation18] showed Ag+ infiltrates into E. coli and inactivates crucial enzymes by attaching with the thiol groups, resulting in inhibition of DNA replication and death of the bacteria.
Pikeperch (Sander lucioperca) is a species of fish isolated from freshwater and brackish habitats in Eurasia, particularly in the Caspian Sea.[Citation19] This species is one of the top-ranked commercial species in north of Iran. In this study, LDPE/Ag/TiO2 nanocomposite films were prepared through the solvent and melt-mixing methods with percentages of 1%, 3%, and 5% (sum of the whole used NPs). The antibacterial activity of the produced films was assessed against S. aureus, E. coli, Candida albicans, and Aspergillus niger using the agar disk-diffusion test. The shelf life of S. lucioperca enclosed in all the films was evaluated for up to 20 days.
Materials and methods
Nanoparticles
Silver and titanium dioxide NPs were purchased from Merck (Germany). Dynamic light scattering (DLS) test and Fourier infrared spectroscopy (FTIR) (Shimadzu, Japan) were conducted to determine the average size and chemical compounds of NPs. Also, LDPE was purchased from Bandar-Emam Petro Chemical Company (Bandar Abbas, Iran).
Nanocomposite preparation: melt mixing
The melt-mixing experiment was carried out at the Polymer Research Institute of Iran. The manufacturing method was based on the studies of Oliani et al.[Citation20] The percentage and amount of materials were calculated according to and weighed by a digital scale with an accuracy of 0.1. The LDPE pellets were mixed with Irganox 1010 in a rotary mixer and the mixture was maintained for 24 h. AgNPs were added using a twin-screw extruder (Clextral, France) based on the volumes presented in .
Table 1. Percentage and weight of film ingredients of the four treatments used in the meltmixing method.
In the method, the bayonet was switched on the twin screw and five thermal regions were set (150–165–170–175–180°C, respectively). The film was produced at a speed of 20 rpm and a thickness of 0.05 mm. After the film () was taken out of the extruder, the aluminium foil was drawn around the film to avoid direct contact with the light ().
Nanocomposite preparation: Melt mixing
TiO2-Ag nanocomposite films were prepared using different volumes of compounds () according to the slightly modified method of Rashedi et al.[Citation21] Xylene (180 ml) was added to an Erlenmeyer flask and heated to its boiling point (145°C). After 30 min of boiling, the LDPE was then weighed, slowly added, and stirred with a magnet at a 250 rpm for 1 h. Nano-Ag and TiO2 were weighed in the dark as quickly as possible and mixed using an ultrasonic probe (UP200S with sonotrode S14; Hielscher, Germany) with a maximum output power of 400 W for proper mixing of the NPs. To prevent the sample from warming, the ultrasonic device was set at 25% of the maximum range, and the time interval between wave pulses was set at 0.5 s. Also, a mixture of water and ice around the sample vessel was used to control the sample temperature and prevent solvent evaporation. The amount of 400 cc of methanol as an anti-solvent solution was added to the mixture, and finally a rigid polymer was formed. Methanol was separated from the polymer using a filter paper and 90 manganese (). After 16 h, to make 4 g of pressurized polymer, 4 g of the final product was weighed using a filter paper. The weighed polymer of each treatment was placed on the central portion of each paper. For the initial heat treatment, a temperature of 160°C with 3000 psi for 4 min was considered, and the main pressure was set to 160°C and 4000 psi for 3 min. The resulting film had no reaction or signs of burns ( and ).
Table 2. Percentage and weight of the film ingredients of the four treatments used in the sol-gel method.
Treatments
The susceptibility of the pathogens was examined against seven groups of LDPEs incorporating different concentrations of Ag+/TiO2. The first group was assigned as control (S1), in which the film comprised LDPE, three films (S2, S3, and S4) produced through the melt-mixing method with defined concentrations () and three films (S5, S6, and S7) produced through the sol-gel method (). These treatments were assessed through two methods. In the first method, they were exposed to S. aureus, E. coli, C. albicans, and A. niger using the disk-diffusion assay. In the second method, the LDPE films were used to package S. lucioperca for the shelf life experiment.
Survey of features of the produced films
In order to observe the morphological characteristics of the produced films, the samples were observed directly by Field Emission Scanning Electron Microscopy using a model S-4160 microscope (Hitachi, Japan) with a precision of 5 nm, a magnification of 20–30,000, and a maximum working voltage of 30 kV. According to the method of Wang and colleagues,[Citation5] the samples were completely cleaned and dried to obtain a high energy electron beam for imaging and chemical analysis. The samples were placed on stubs, and electrical contact between the stubs and samples was generated.
Microorganism preparation and antibacterial disk design
E. coli (ATCC 25922), S. aureus (ATCC 6538) as representative gram-negative and positive bacteria, respectively, A. niger (ATCC 9142) as the representative mold representative and C. albicans (ATCC 10231) as the representative fungus were cultured on a gradient nutrient agar tubes and kept at 4oC. During the preparation of liquid culture from the bacteria, a loop of each bacterial specimen was removed, cultured in sterilized conditions in 50 ml of sterilized BHI medium and then incubated at 37°C for 24 h in a shaking incubator. Each microbial culture was diluted in sterile distilled water to a suitable viable microbial population for testing, which was determined to be 107 colony-forming units (CFU)/ml. Antimicrobial activity was determined using the disk-diffusion method in an agar medium. Nanofilms were prepared in different concentrations, and cut to 0.5 cm in diameter, placed in layers of filter papers, and sterilized in the incubator at 121°C for 15 min.[Citation22] Under strictly sterile conditions, the prepared antibiogram discs were transferred to Mueller-Hinton agar on which the particular microorganism had been inoculated to create a lawn of growth. The susceptibility of microbial strains to each treatment was determined by measuring the diameters of the zones of growth inhibition as an antimicrobial index of each film.[Citation23]
Fish sample preparation
S. lucioperca with an average weight of 2–4 kg were freshly purchased commercially in Mazandaran Province, located in the northern part of Iran. The fish were transferred to the Health Laboratory within 6 h in a closed and insulated container containing a sufficient amount of ice. Upon arrival, the fish were immediately peeled, evacuated, gutted, and fillets with dimensions of 5 × 4 × 5 cm were prepared. The fillets were completely cleaned of blood clots and other waste by washing with cold water. The prepared nanocomposite films were wrapped around the fish pieces and stored in a refrigerator for 20 days at 4°C. Fish were randomly assigned to microbiological tests every 5 days[Citation24]
Microbial tests for fish samples
Samples were taken for microbiological tests as described previously by Sallam.[Citation25] After preparing serial dilutions, 1 cc of each dilution was inoculated to plate count agar. Plates were incubated for 48 h at 37°C for aerobic mesophilic bacteria and for 10 days at 7°C for psychrophilic bacteria. Enterobacteriaceae were cultured on Violet Red Bile Glucose Agar for 2 days at 30°C. Colony count was done and recorded as log CFU/g.
Statistical analyses
In order to determine the differences of inhibition zone diameters (mm), one-way ANOVA and Tukey’s Bonferroni multiple comparisons test for pairwise differences were used (p < 0.05). Colony count data as log CFU/g for Enterobacteriaceae, mesophilic, and psychrophilic bacteria were analysed using one-way ANOVA with repeated measures through the general linear model procedure of SPSS version 16 (SPSS Inc., Chicago, IL, USA) within 20 days while the fish samples were taken from the fillets every fifth day (n = 3).
Results
Analysis of NPs
DLS analysis showed that the average size of silver NPs was approximately 68 nm. Also, FTIR analysis showed that no confounding factors were mixed in the mixture of NPs. (). A peak wave number of approximately 1,748 cm−1 is related to the C=O (carbonyl functional group) and a peak in the range of 1,000–1,300 cm−1 showed C–O (alcohol-end groups). Evidence of the presence of other numbers as 2,898, 1,022, and 767 cm−1 indicating C–H stretching, C–O stretching, and =C–H bending, respectively. Additionally, the wave number of 559 and 400 cm−1 refers to the fingerprint region of LDPE.
Effect of film type on growth inhibition
Inhibition zone diameters produced by the different nanocomposite LDPE/Ag/TiO2 films against E. coli are listed in and depicted in . The maximum diameter (mm) was observed for S4 (16.76 ± .10). The inhibition zone was significantly larger (p < 0.05) than those of S7 and S3 (12.64 ± .06 and 10.04 ± .10, respectively). The minimum inhibition zone diameter was produced by S5 (4.28 ± .05). The control displayed no inhibition.
Table 3. Effect of different films on the inhibition zone diameters (mm) of E. coli.
Inhibition zone diameters produced by the different nanocomposite LDPE/Ag/TiO2 films against S. aureus are listed in and depicted in . The maximum diameter (mm) was observed for S4 (18.45 ± 0.15), which was significantly larger (p < 0.05) than the diameters produced by S7 and S3 (15.41 ± .05 and 11.33 ± 0.15, respectively). The minimum value was S5 (4.39 ± .00). The control displayed no inhibition.
Table 4. Inhibition zone diameters (mm) of inoculated S. aureus.
Inhibition zone diameters produced by the different nanocomposite LDPE/Ag/TiO2 films against A. niger are listed in and depicted in . The maximum diameter (mm) was observed for S4 (13.83 ± .11), which was significantly larger (p < 0.05) than the diameters produced by S7 and S3 (11.73 ± .13 and 8.69 ± .08, respectively). The values for S2 and S5 were markedly less (3.61 ± .00 and 2.74 ± .00, respectively) and were significantly different (p < 0.05). The control displayed not growth inhibition.
Table 5. Inhibition zone diameters (mm) of inoculated A. niger.
Inhibition zone diameters produced by the different nanocomposite LDPE/Ag/TiO2 films against C. albicans are listed in and depicted in . The maximum diameter (mm) was observed for S4 (14.65 ± .07), which was significantly larger (p < 0.05) than the diameters produced by S7 and S3 (12.72 ± .09 and 8.28 ± .00, respectively). The minimum diameters were produced by S2 (3.97 ± .06) and S5 (2.73 ± .14), which were significantly different (p < 0.05). The control displayed no growth inhibition.
Table 6. Inhibition zone diameters (mm) of inoculated C. albicans.
Effect of film type on fillet shelf life
The minimum marginal mean value of the mesophilic bacteria (2.67 ± 0.08 log CFU/g) was produced by S4 on day 5 of the experiment. This value was significantly (p < 0.05) less than those of other treatments and control. The S4 value was followed by those of the S2 and S7 groups (3.03 ± 0.08, 3.06 ± 0.08, respectively), which were not significantly different from each other (p > 0.05). This pattern continued throughout the experiment (). At day 10, the value for S4 (3.26 ± 0.06) remained the minimum value, followed by S7 (3.52 ± 0.06). At day 15, the minimum marginal mean value of the mesophilic bacteria was 5.20 ± 0.12, which was significantly different (p < 0.05) compared with other groups (next in descending order were 6.23 ± 0.12 for S7 and 6.27 ± 0.12 for S3). On day 20, S4 again displayed the minimum value (6.25 ± 0.15) with a significant difference (p < 0.05) compared to the other groups.
displays the growth results (all log CFU/g) for the Enterobacteriaceae with the different film types measured over 20 days. The minimum marginal mean value was observed at day 5 for S7 (2.07 ± 0.06), followed by S6, S3, and S4 (2.14 ± 0.06, 2.14 ± 0.06, and 2.17 ± 0.06, respectively). The latter three values did not differ significantly (p > 0.05). All the treatment values were significantly less (p < 0.05) compared with the control. At day 10, the minimum marginal mean value was observed for S5 (2.26 ± 0.10), followed by S4 (2.31 ± 0.10), although the difference was not significant (p > 0.05). S6 and S7 values (2.54 ± 0.10 and 2.54 ± 0.10, respectively) were significantly different (p < 0.05) from those of S5 and S4. This pattern was different on day 15. At that time, the minimum marginal mean value was displayed by S4 (2.59 ± 0.08), followed by S7 and S3 (2.89 ± 0.08 and 2.95 ± 0.08, respectively). On day 20, the minimum value was again displayed by S4 (3.83 ± 0.06), with a remarkable difference compared to S7 (4.31 ± 0.08). These values were significantly different (p < 0.05) compared to the other groups.
Concerning the psychrophilic bacteria (), the minimum marginal mean value (log CFU/g) was observed on day 5 for S4 (3.79 ± 0.03), which was significantly different (p < 0.05) from the next value of S3 (4.11 ± 0.15). All the values were significantly different (p < 0.05) than the control. At day 10, the minimum marginal mean value of the Enterobacteriaceae was observed for S4 (4.00 ± 0.03), followed by S7 (4.52 ± 0.03). The values differed significantly (p < 0.05). The pattern observed on day 15 () revealed the minimum marginal mean value for S4 (4.87 ± 0.15), followed by S7 (5.11 ± 0.1). On day 20, the minimum value was again displayed by S4 (5.54 ± 0.15) and was significantly different from the other values (p < 0.05).
Figure 10. Marginal means (log CFU/g) of the psychrophilic bacteria for the different film types with time.
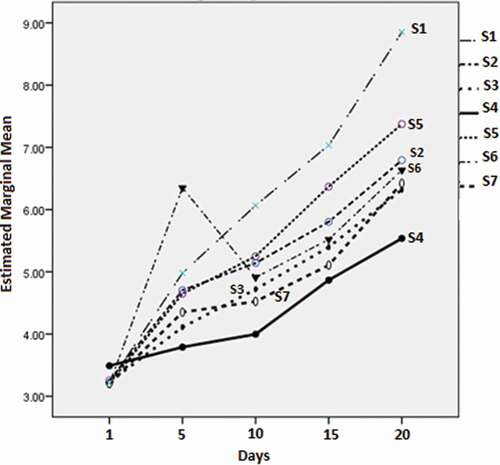
LDPE produced through the sol-gel process was not homogeneous ( and ); as a result, silver particles could be well-loaded on TiO2. This could be due to the complete insolubility of the NPs in the acetonitrile solvent. When processing was based on the melt-mixing method, perfect homogeneity of samples was observed (). In the control group (), LDPE was not covered by Ag NPs.
Figure 11. (a and b) LDPE produced using 0.5% Ag and 2.5% TiO2 as well as 1% of silver, which were produced by the sol-gel method. (c) Production using the melt-mixing method 0.5% Ag and 2.5% TiO2 as well as 1% of silver. (d) Control group with no Ag nanoparticles. Examination at a magnification of KX60 revealed a particle size of 68 nm.
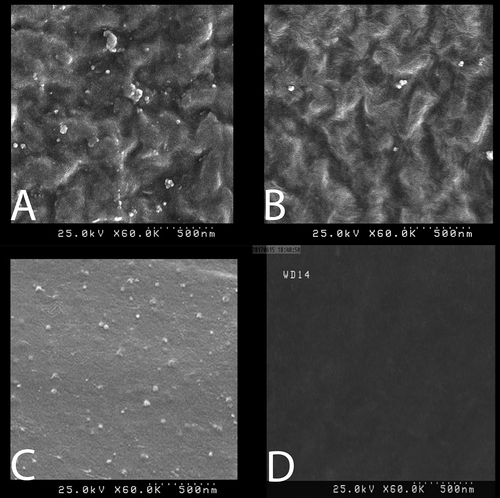
Discussion
Nanocomposites based on LDPE/Ag/TiO2 prepared by melt or sol-gel methods have modified properties that enable their efficient degradation under mild UV illumination or sunlight, due to the dispersion of TiO2 NPs. The attachment of Ag+ from the TiO2 NPs may bestow antimicrobial properties. [Citation5,Citation8] Gupta et al.[Citation26] reported that Ag-TiO2 nanocomposites having a synergistic antimicrobial property in cooperation with a photoactivity effect have increased anti-pathogenic activity than pure Ag and TiO2 NPs alone.[Citation27] Presently, inhibition zone diameters for the different nanocomposites of LDPE/Ag/TiO2 indicated that the nanocomposites used in this study are efficacious against the selected pathogens (). For E. coli, the inhibition zone diameters increased in an Ag/TiO2 concentration-dependent manner. Mirhoseini and Salabat[Citation28] showed that this result could be due to the accumulation of nanoparticles in the polymer matrix, which causes a decrease in the number of surface-active sites at which the nanocomposite can incorporate. The efficacy of the melt-mixing method was better than the sol-gel method concerning the inactivation of E. coli, qualitatively similar to the results of Yu et al.,[Citation29] who reported an increasing diameter of the inhibition zone from 3 to 7 mm with increasing silver NP in the composite films. However, the present values of 4.60 ± .10 to 16.76 ± .10 mm for the melt-mixing method, and from 4.28 ± .05 to 12.64 ± .06 mm for the sol-gel method differed from the values reported by Lopez Goerne et al.,[Citation30] who described that NPs containing Ag/TiO2 produced by the sol-gel process increased the zone of inhibition to approximately 10, 15.3, and 10.3 mm for E. coli, S. aureus and C. albicans, respectively. The values we obtained for S. aureus and C. albicans were different (18.45 ± 0.15 and 14.65 ± .07 in the melt-mixing method, respectively, and 15.41 ± .05 and 12.72 ± .09 in the sol-gel method).
Cheng et al.[Citation31] reported that the use of an Ag/TiO2 composite containing 0.5 M AgNO3 in the presence of sunlight inhibited 99.99% of the growth of E. coli and S. aureus (15.2 and 14.9 mm, respectively, through the hierarchical substrate of Ag). These values were close to the present findings that the best inhibition zone for E. coli and S. aureus was 16.76 ± .10 and 18.45 ± 0.15 mm, respectively, with a significantly higher value attained using the melt-mixing method compared to the sol-gel process with the increased dispersion of Ag/TiO2 gained with 3% Ag. Similarly, Gupta and colleagues[Citation26] documented that an Ag/TiO2 nanocomposite produced through the sol-gel process completely inactivated bacteria including E. coli and S. aureus when the TiO2 NPs were doped with 3% Ag. In another study, the efficiency of Ag-TiO2 NPs to completely kill E. coli populations was increased at an Ag concentration of 2.5%.[Citation32] With respect to the effect of TiO2, no significant difference was found with LDPE used in sunlight or in darkness.[Citation33]
Similar to this study, LDPE nanocomposites with customized Ag/TiO2 NPs were confirmed to be more effective against staphylococci than E. coli.[Citation34] This result was similar to those of Threepopnatkul et al.,[Citation35] who showed that TiO2 was two times more effective on S. aureus than E. coli. Based on the results of Rahmawati et al.,[Citation36] the deposition of Ag on the TiO2 improves the produces performance in E. coli disinfection compared with Cu–TiO2 after 30 min. Similar to this study, Zhang and Chen[Citation37] applied Ag (7.4 wt%)/TiO2 NPs as the anti-bacterial compound for cultures of E. coli (105 CFU/mL) and confirmed that treatment with TiO2 (200 µg/mL) produced only weak growth inhibition, while growth was remarkably prohibited when Ag was added.[Citation38] Mihaly Cozmuta and colleagues[Citation12] documented that fresh lettuce packaging composed of one polypropylene/TiO2 decreased E. coli contamination from 6.4 to 4.9 log CFU/g after 1 day, while that of the control group decreased from 6.4 to 6.1 log CFU/g.
It was concluded that applying LDPE/Ag/TiO2 nanocomposite films produced through the melt-mixing and sol-gel methods is beneficial for fish packaging in terms of inactivate representative pathogens including E. coli, S. aureus, C. albicans, and A. niger. The efficiency of the melt-mixing method was much better than the sol-gel process. Comparison of efficacy was done using measurement of diameter of inhibition zone and evaluation of the results using Tukey's statistical method. There were significant (p < 0.05) differences between inhibition zone caused by films produced by the melt-mixing method. Therefore, application of low percentages of NPs in food packaging which are produced by melt-mixing method is highly suggested for expensive food products since their remarkable effects on increasing shelf life.
References
- Han, J.H. Innovations in Food Packaging; Elsevier Science & Technology Books 2005. ISBN: 0123116325.
- Mihindukulasuriya, S.; Lim, L.-T. Nanotechnology Development in Food Packaging: A Review. Trends in Food Science & Technology 2014, 40(2), 149–167.
- Tharanathan, R. Biodegradable Films and Composite Coatings: Past, Present and Future. Trends in Food Science & Technology 2003, 14(3), 71–78.
- Zhang, J.; Wang, X.; Lu, L.; Li, D.; Yang, X. Preparation and Performance of High‐Impact Polystyrene (HIPS)/Nano‐TiO2 Nanocomposites. Journal of Applied Polymer Science 2003, 87(3), 381–385.
- Wang, Z.; Li, G.; Xie, G.; Zhang, Z. Dispersion Behavior of TiO2 Nanoparticles in LLDPE/LDPE/TiO2 Nanocomposites. Macromolecular Chemistry and Physics 2005, 206(2), 258–262.
- Park, J.S.; Kim, J.H.; Nho, Y.C.; Kwon, O.H. Antibacterial Activities of Acrylic Acid‐Grafted Polypropylene Fabric and Its Metallic Salt. Journal of Applied Polymer Science 1998, 69(11), 2213–2220.
- Guzman, M.; Dille, J.; Godet, S. Synthesis and Antibacterial Activity of Silver Nanoparticles against Gram-Positive and Gram-Negative Bacteria. Nanomedicine: Nanotechnology, Biology and Medicine 2012, 8(1), 37–45.
- Zan, L.; Fa, W.; Wang, S. Novel Photodegradable Low-Density Polyethylene− TiO2 Nanocomposite Film. Environmental Science & Technology 2006, 40(5), 1681–1685.
- Yu, J.-G.; Yu, H.-G.; Cheng, B.; Zhao, X.-J.; Yu, J.C.; Ho, W.-K. The Effect of Calcination Temperature on the Surface Microstructure and Photocatalytic Activity of TiO2 Thin Films Prepared by Liquid Phase Deposition. The Journal of Physical Chemistry B 2003, 107(50), 13871–13879.
- Cho, M.; Chung, H.; Choi, W.; Yoon, J. Linear Correlation between Inactivation of E. coli and OH Radical Concentration in TiO2 Photocatalytic Disinfection. Water Research 2004, 38(4), 1069–1077.
- Chawengkijwanich, C.; Hayata, Y. Development of TiO 2 Powder-Coated Food Packaging Film and Its Ability to Inactivate Escherichia coli in Vitro and in Actual Tests. International Journal of Food Microbiology 2008, 123(3), 288–292.
- Mihaly Cozmuta, A.; Peter, A.; Mihaly Cozmuta, L.; Nicula, C.; Crisan, L.; Baia, L.; Turila, A. Active Packaging System Based on Ag/TiO2 Nanocomposite Used for Extending the Shelf Life of Bread. Chemical and Microbiological Investigations. Packaging Technology and Science 2015, 28(4), 271–284.
- Farrokhi,S.; Ahari, H.; Abedini, M. R. (2017) Comparative Effects Of Colloidal silver Nanoparticles Used in Packaging Film and Spray In Inactivating Bacteria Experimentally Added to Chicken Eggshells. International Journal of Food Properties, 20(10), 2314-2322, doi:10.1080/10942912.2016.1236274
- Ruparelia, J.P.; Chatterjee, A.K.; Duttagupta, S.P.; Mukherji, S. Strain Specificity in Antimicrobial Activity of Silver and Copper Nanoparticles. Acta biomaterialia 2008, 4(3), 707–716.
- Martinez-Gutierrez, F.; Olive, P.L.; Banuelos, A.; Orrantia, E.; Nino, N.; Sanchez, E.M.; Ruiz, F.; Bach, H.; Av-Gay, Y. Synthesis, Characterization, and Evaluation of Antimicrobial and Cytotoxic Effect of Silver and Titanium Nanoparticles. Nanomedicine: Nanotechnology, Biology and Medicine 2010, 6(5), 681–688.
- Stobie, N.; Duffy, B.; McCormack, D.E.; Colreavy, J.; Hidalgo, M.; McHale, P.; Hinder, S.J. Prevention of Staphylococcus epidermidis Biofilm Formation Using a Low-Temperature Processed Silver-Doped Phenyltriethoxysilane Sol–Gel Coating. Biomaterials 2008, 29(8), 963–969.
- Feng, Q.; Wu, J.; Chen, G.; Cui, F.; Kim, T.; Kim, J. A Mechanistic Study of the Antibacterial Effect of Silver Ions on Escherichia coli and Staphylococcus aureus. Journal of Biomedical Materials Research 2000, 52(4), 662–668.
- Yamanaka, M.; Hara, K.; Kudo, J. Bactericidal Actions of a Silver Ion Solution on Escherichia coli, Studied by Energy-Filtering Transmission Electron Microscopy and Proteomic Analysis. Applied and Environmental Microbiology 2005, 71(11), 7589–7593.
- Jones, S.; Ahonen, H.; Granlund, L.; Arsiola, T.; Taskinen, J. Two Novel Microsporidia in Skeletal Muscle of Pike-Perch Sander lucioperca and Burbot Lota Lota in Finland. Journal of Parasitology 2017, 103(1), 95–102.
- Oliani, W.L.; Fermino, D.M.; Komatsu, L.G.H.; Lugao, A.B.; Rangari, V.K.; Lincopan, N.; Parra, D.F. Preparation and Characterization of Polyethylene Nanocomposites with Clay and Silver Nanoparticles. In Characterization of Minerals, Metals, and Materials 2017; Springer, 2017; pp 709–718.
- Rashedi, H.; Partovi, A.; Fattah, R.; Khiabani, M. The Effect of Silver Nanofilms Based on Titaniom Dioxide on the Shelf-Life of Rainbow Trout Fillet (Oncorhynchus mykiss). Iranian Journal of Nutrition Sciences & Food Technology 2016, 11(3), 85–92.
- Mikiciuk, J.; Mikiciuk, E.; Szterk, A. Physico‐Chemical Properties and Inhibitory Effects of Commercial Colloidal Silver Nanoparticles as Potential Antimicrobial Agent in the Food Industry. Journal of Food Processing and Preservation 2017, 41(2).
- Saadatmand, M.; Yazdanshenas, M.; Rezaei Zarchi, S.; Yosefi Talori, B.; Negahdari, M. The Antimicrobial Activity of Chitosan nanocomposite-TiO2, and Its Application on the Gauze Hospital. Laboratory J 2012, 6(1), 57–59.
- Duun, A.S.; Rustad, T. Quality of Superchilled Vacuum Packed Atlantic Salmon (Salmo Salar) Fillets Stored At− 1.4 And− 3.6 C. Food Chemistry 2008, 106(1), 122–131.
- Sallam, K.I.;. Antimicrobial and Antioxidant Effects of Sodium Acetate, Sodium Lactate, and Sodium Citrate in Refrigerated Sliced Salmon. Food Control 2007, 18(5), 566–575.
- Gupta, K.; Singh, R.; Pandey, A.; Pandey, A. Photocatalytic Antibacterial Performance of TiO2 and Ag-Doped TiO2 against S. aureuS. P. aeruginosa and E. coli. Beilstein Journal of Nanotechnology 2013, 4, 345.
- Li, M.; Noriega-Trevino, M.E.; Nino-Martinez, N.; Marambio-Jones, C.; Wang, J.; Damoiseaux, R.; Ruiz, F.; Hoek, E.M. Synergistic Bactericidal Activity of Ag-TiO2 Nanoparticles in Both Light and Dark Conditions. Environmental Science & Technology 2011, 45(20), 8989–8995.
- Mirhoseini, F.; Salabat, A. Antibacterial Activity Based Poly (Methyl Methacrylate) Supported TiO2 Photocatalyst Film Nanocomposite, 2015.
- Yu, B.; Leung, K.M.; Guo, Q.; Lau, W.M.; Yang, J. Synthesis of Ag–TiO2 Composite Nano Thin Film for Antimicrobial Application. Nanotechnology 2011, 22(11), 115603.
- Lopez Goerne, T.; Alvarez Lemus, M.; Morales, V.; López, E.; Ocampo, P. Study of Bacterial Sensitivity to Ag-TiO2 Nanoparticles. Journal of Nanomedicine and Nanotechnology S 2012, 5, 2.
- Cheng, H.; Ye, J.; Sun, Y.; Yuan, W.; Tian, J.; Bogale, R.F.; Tian, P.; Ning, G. Template-Induced Synthesis and Superior Antibacterial Activity of Hierarchical Ag/TiO 2 Composites. RSC Advances 2015, 5(98), 80668–80676.
- Hamad, A.; Li, L.; Liu, Z.; Zhong, X.L.; Wang, T. Picosecond Laser Generation of Ag–TiO2 Nanoparticles with Reduced Energy Gap by Ablation in Ice Water and Their Antibacterial Activities. Applied Physics A 2015, 119(4), 1387–1396.
- Bodaghi, H.; Mostofi, Y.; Oromiehie, A.; Zamani, Z.; Ghanbarzadeh, B.; Costa, C.; Conte, A.; Del Nobile, M.A. Evaluation of the Photocatalytic Antimicrobial Effects of a TiO 2 Nanocomposite Food Packaging Film by in Vitro and in Vivo Tests. LWT-Food Science and Technology 2013, 50(2), 702–706.
- Cheng, Q.; Li, C.; Pavlinek, V.; Saha, P.; Wang, H. Surface-Modified Antibacterial TiO 2/Ag+ Nanoparticles: Preparation and Properties. Applied Surface Science 2006, 252(12), 4154–4160.
- Threepopnatkul, P.; Wongnarat, C.; Intolo, W.; Suato, S.; Kulsetthanchalee, C. Effect of TiO2 and ZnO on Thin Film Properties of PET/PBS Blend for Food Packaging Applications. Energy Procedia 2014, 56, 102–111.
- Rahmawati, F.; Kusumaningsih, T.; Hapsari, A.; Hastuti, A. Ag and Cu Loaded on TiO2/graphite as a Catalyst for Escherichia coli-Contaminated Water Disinfection. Chemical Papers 2010, 64(5), 557–565.
- Zhang, H.; Chen, G. Potent Antibacterial Activities of Ag/TiO2 Nanocomposite Powders Synthesized by a One-Pot Sol− Gel Method. Environmental Science & Technology 2009, 43(8), 2905–2910.
- Becaro, A.A.; Puti, F.C.; Correa, D.S.; Paris, E.C.; Marconcini, J.M.; Ferreira, M.D. Polyethylene Films Containing Silver Nanoparticles for Applications in Food Packaging: Characterization of Physico-Chemical and Anti-Microbial Properties. Journal of Nanoscience and Nanotechnology 2015, 15(3), 2148–2156.