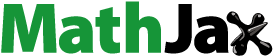
ABSTRACT
In this study, the effects of the disulfide bond cleavage induced by peracetic acid oxidation on the surface properties and surface hydrophobicity of soy proteins isolate (SPI) were investigated. The surface hydrophobicity, foaming capacity and emulsifying capacity of oxidized-SPI increased gradually at the initial stage with the increase of peracetic acid concentration. When the concentration of peracetic acid was increased up to 0.4%, compared with that of native SPI, the surface hydrophobicity, foaming capacity, emulsifying capacity and emulsifying stability of oxidized-SPI increased by 114.0, 81.4, 65.2, 49.8%, respectively, and achieved optimal results. However, excessive oxidation led to a decrease in surface hydrophobicity, foaming capacity and emulsifying stability of SPI, but it had no obvious effect on the foaming stability and emulsifying capacity of SPI. The foaming capacity, emulsifying capacity and emulsifying stability of SPI were positively related to the changes of surface hydrophobicity which caused by disulfide bond cleavage. The results of fluorescence spectroscopy, CD spectroscopy, and particle size analysis showed that the disulfide bond cleavage did cause great changes in the molecular structure of SPI, but there was no clear correlation between the molecular structural change and the surface activity of SPI. These suggested that the improvement of foaming capacity, emulsifying capacity and emulsifying stability of SPI could be achieved by changing its surface hydrophobicity via peracetic acid oxidation.
Introduction
Similar to traditional surfactant molecules, a protein molecule has both hydrophobic and hydrophilic parts. Therefore, protein molecules may be adsorbed to the oil/air/water interface, resulting in a decrease in interfacial tension, which has a potential to act as a surfactant or surface-active material in theory because of their amphiphilic nature. However, many studies have shown that most native proteins cannot be used as surfactants because their surface activity is too weak. Enhancing the surface activity of proteins by changing the molecular structure of proteins, such as ultrasound treatment[Citation1], heating and chemical modification[Citation2,Citation3], is a necessary technical way to expand and utilize their surface activity. In addition, it is the amphiphilic nature of the protein that makes the polypeptide chains automatically fold into spherical molecules in the aqueous environment because the non-polar solutes have a tendency to adhere to one another in an aqueous environment (so-called hydrophobicity).[Citation4] The automatic folding of protein polypeptide chains leads to a situation that most of the non-polar amino acid residues are embedded within the protein molecules to form a hydrophobic core, while the polar amino acid residues are on the surface of the protein molecules in hydrophilic environments. Therefore, the lack of sufficient hydrophobic groups on the surface protein can result in a poor surface activity of most natural spherical proteins. For most natural proteins, the formation of the hydrophobic core is very important for stabilizing the tertiary structure of proteins. In some proteins, disulfide bonds provide additional stability due to their limitation and locking of protein spatial structures.[Citation5]
Because of its renewable resources, good biocompatibility, biodegradability, and processability, soy protein isolate (SPI) has great potential applications in food industry, agriculture and biomaterials.[Citation6] SPI is a mixture of proteins, and is divided into 2S, 7S, 11S, and 15S according to their sedimentation coefficients. 7S and 11S account for 80% of all SPI. Each 7S globulin molecule has 0-SH radical and two disulfide bonds, whereas each 11S globulin molecule has 2-SH radical and 20 disulfide bonds. Native 11S is known to have a compact structure stabilized by disulfide bonds and thus its emulsifying and foaming ability is lower than that of 7S.[Citation7] Therefore, partially cleaving disulfide bonds may change the molecular structure and let more hydrophobic residues to be exposed to the surface of SPI, which in turn affects the properties such as gelation, foaming, and emulsifying. Thus, it is an effective way to improve the protein surface activity by partially cleaving disulfide bonds to change the molecular structure and surface hydrophobicity of SPI.
It is reported that sulfur-containing amino acids are the main targets of single electron oxidants (e.g. HO., RO., ROO) and double electron oxidants (e.g. H2O2, ONOOH, O2, O3)[Citation8,Citation9], the thiosulfinates (R-S-S(= O)-R and disulfide S-oxides) are the major initial oxidation products. However, the further change of these initial oxidation products will lead to-S-S- bond cleavage and sulfonic acid (RSO3H) formation.[Citation10] Compared with hydrogen peroxide, peracetic acid has stronger oxidation capacity and more moderate reaction.[Citation11] In addition, peracetic acid is usually used as an antimicrobial agent in dairy and cheese processing plants, on food processing equipment, and in pasteurizers in breweries, wineries, and beverage plants.[Citation12] Therefore, the objective of this work is to study the effects of peracetic acid oxidation on the surface hydrophobicity and surface properties of SPI, and to evaluate the relationship between changes in structure, surface hydrophobicity, and surface properties of SPI ().
Materials and methods
Materials
Defatted soybean meal (Kunhua Biotechnology Co., Ltd., Anyang, Henan, China); peracetic acid (AR, Xilong Chemical Co., Ltd, Guangzhou, Guangdong, China); 5,5‘-Dithiobis-(2-nitrobenzoic acid) (DTNB), 8-Anilino-1-naphthalenesulfonic acid (ANS), Ortho-phthaldaldehyde (OPA) and Glutathione (Reduced) (Aladdin Biotechnology Co. Ltd., Shanghai, China); . The other chemicals were purchased from the Xilong Chemical Co. Ltd., Guangzhou, Guangdong, China.
Preparation of samples
Preparation of SPI
The preparation of the SPI was adopted from a method developed by Samoto with some slight modifications.[Citation13] Defatted soybean meal was ground through an 80-mesh sieve and mixed with water at a 1:15 mass-to-volume ratio. The pH was adjusted to 8.0 with 2.0 M NaOH solution. After stirring for 2 h with an electric driving stirrer, the resulting suspension was centrifuged at 8000 g for 20 min at 4°C with a centrifuge (Avanti J-26 XPI, the United States) to remove the insoluble material. Then, the pH of the supernatant was adjusted to pH 4.5 with 2.0 M HCl, and the precipitate was collected by centrifugation at 5000 g, for10 min at 4°C. The precipitate was then redissolved in 5-fold deionized water, and the pH was adjusted to 7.0 with 2.0 M NaOH. The neutral SPI solution was frozen at −20°C, and then freeze-dried using a freezing dryer (Christ Alpha 1-4LDplus, Germany). The freeze-dried samples were then stored until use.
Preparation of oxidized SPI with different concentrations of peracetic acid
The SPI was dissolved in a phosphate buffer solution, and different volumes of peracetic acid were, respectively, added into SPI solution to obtain 0, 0.2%, 0.4%, 1%, 1.4%, and 1.8% (v/v) peracetic acid concentration, and the obtained samples were sequentially numbered 1–6. The obtained sample was subjected to a reaction in a dark environment at 4 °C for 12 h, and then they were freeze-dried and stored until use.
Disulfide bond content
A slight modification to the method developed by Ellman et al.[Citation14] was used for the spectrophotometric analysis. We first prepared 0.1% DTNB solution, 0.1% NTSB solution, 2 mg/ml glutathione standard solution, respectively. The oxidized-SPI (0.4 mL, 25 mg/mL) and DTNB solution (0.4 mL) was mixed about 20 min at room temperature (25 °C), and then the mixture was diluted to 10 mL with a phosphate buffer (pH 8.0) for spectrometric measure. The absorbance at 412 nm was obtained from an ultraviolet visible spectrophotometer (Cary-60, the United States). In the similar way, the mixed solution containing 0.4 mL oxidized-SPI and 0.4 mL NTSB solution was prepared to measure the total disulfide and sulfhydryl content. Different concentrations of glutathione solution were used to generate a standard curve which is described as formula (1):
Where: y =absorbance at 412nm, and x = mass concentration of reductive glutathione concentration (mg/mL). Thiol concentration in oxidized SPI was calculated according to EquationEquation (2)(2)
(2) .
Where: The molecular mass of reductive glutathione is 307.32 g/mol
Disulfide opening rate of oxidized SPI was calculated according to EquationEquation (3)(3)
(3) .
Where: = disulfide bond cleaving rate,
= disulfide bond content before modification, and
= disulfide bond content after modification
Surface hydrophobicity
A slight modification to the method developed by Hayakawa and Nakai[Citation15] was used to measure surface hydrophobicity. The surface hydrophobicity of SPI before and after oxidation was determined using 8-anilino-1-naphthalenesulfonic acid (ANS) as fluorescent probe. Twenty microliters of ANS (8 mM/L) were added into different concentrations samples, respectively. The fluorescence spectra of these samples were obtained from a fluorescent spectrophotometer (F-320, Shimadzu, Japan). The slope of the initial phase of the curve (S0) was the surface hydrophobicity index of the samples.
Surface tension and critical micelle concentration (CMC)
The surface tension of the protein solutions of different concentrations was determined by an automatic interface tensiometer (DT-102, Shandong Zibo Huakun Electronics Co. Ltd. China). The CMC and the minimum surface tension (γCMC) of protein solution were determined by plotting the surface tension as a function of the logarithm of the concentration.[Citation16]
Foaming capacity and stability
According to the methods mentioned by Wang[Citation17], foaming capacity and stability can be measured, using the following steps. The oxidized SPI solutions (10 mL) were diluted to 50 mL with deionized water and were subjected to high-speed shear mixing at 10000 r/min for 1 min by a high-speed shear mixed emulsifier (100LX, China). The above solutions were rapidly transferred into a 100 mL graduated cylinder, and the initial bubble volume (V0) was recorded. After standing at room temperature (30°C), the bubble volume was recorded again at 30 min (V30). FC and FS were calculated using formula (4) and (5).
Emulsifying capacity and stability
The oxidized SPI solutions (15 mL) were mixed with 5 mL of soybean oil. The mixture was emulsified with a high-speed shear mixed emulsifier (100LX, China) at 10000r/min for 1 min. A 20μL aliquot of the solution was taken at 0 min and at 30 min after the emulsification. Then, 20 μL of the SPI was mixed with 5 mL of 0.1% SDS (m/v) dissolved in a tube. An ultraviolet visible spectrophotometer (Cary-60, the United States) was used to measure the absorbance of the resulting solutions at 500 nm. The EC and ES were calculated using formula (6) and (7).
Where: T = 2.303; N is the dilution factor of 250; c is the protein concentration before mixing (g/mL); φ is the volume fraction of the oil phase in the emulsion (0.25); A0 is the absorbance value at 0 min; and A30 is the absorbance value at 30 min.
Fluorescence spectrum
The intrinsic fluorescence of different samples was obtained from a fluorescent spectrophotometer (F-320, Shimadzu, Japan) at an excitation wavelength of 290 nm. The excitation slit was 5 nm and the emission slit was 3 nm. Scanning range was 300–420 nm and scanning speed was middle.
Particle size distribution
A malvern zetasizer (Nano ZS90, UK) was used to measure the particle size distribution of each sample at room temperature using a wavelength of 623 nm and a scattering angle of 90°.
Circular dichroism (CD) spectra
The CD spectrum of the sample solution was performed at 25°C in a 1 mm colorimetric dish using a circular dichroism spectrometer (Chirascan, UK). The following conditions were used: wavelength scanning range was 180–260 nm, scanning speed was 100 nm/min, response time was 0.25 s, slit width was 0.1 nm, and sensitivity was 10mdeg/cm.
Statistical analysis
Analysis and graphic presentations were prepared using Origin software (version 8.0, OriginLab, USA). Correlation analysis among functional properties and the surface hydrophobicity was determined with Pearson correlation coefficients by using SPSS (version 22.0, USA).Statistical analysis was performed using SPSS using analysis of variance (ANOVA) with significant differences determined by the least significant difference (LSD) test. Significance was assumed at p< 0.05.
Results and discussion
Controlled oxidation by using peracetic acid to cleave disulfide bonds
The content of free sulfhydryl group in SPI before or after peracetic acid oxidation treatment was determined. The results showed that peracetic acid could oxidize the disulfide bonds to sulfonic acid and disconnect the disulfide bonds in SPI as shown in . It was clear that the rate of disulfide bond cleavage increased with the increase of the peracetic acid concentration. When the peracetic acid concentration was up to 1.8%, the disulfide bond cleavage was 96.27%. Previously, a similar result has been gotten by using performic acid to oxidize SPI. Performic acid also could cleave disulfide bonds and loosen the structure of peptide chains, causing the grafting reaction between chitosan and SPI to be easy.[Citation18] Early in the middle of the last century, Sanger and Hirs[Citation19,Citation20] reported that the disulfide bonds could be cleaved by performic acid to form cysteic acid. Liu et al.[Citation21] also found that the disulfide bonds could be cleaved by hydrogen peroxide to form cysteic acid. Moreover, dithiothreotol or mercaptoethanol is used to reduce the disulfide bond to free mercapto group, which is one of the traditional methods of disulfide bond cleavage.[Citation22] However, when the concentration of dithiothreitol or mercaptoethanol decreases, the free mercapto groups may be oxidized again to form a disulfide bond, which will cause the unfolded polypeptide chain to fold again. In addition, dithiothreotol and mercaptoethanol themselves are toxic compounds. On the contrary, the reaction of peracetic acid is mild and easy to decompose into acetic acid and oxygen without any harmful components after reaction. In addition, the advantage of peracetic acid oxidation is that the sulfonic groups formed by peracetic acid oxidation cannot be further reduced or re-oxidized, thus preventing the recovery of disulfide bonds. Therefore, peracetic acid is an ideal cleaving agent for the disulfide bond cleavage.
Surface hydrophobicity
As showed in , peracetic acid oxidization exhibited a notable influence on surface hydrophobicity of SPI. The surface hydrophobicity of oxidized-SPI increased gradually at the initial stage with a lower disulfide bond cleavage. However, the surface hydrophobicity of oxidized-SPI did not continue to increase after the peracetic acid concentration exceeded 0.4%. An appropriate disulfide bond cleavage was more important for SPI to cause more hydrophobic groups to be exposed to the molecular surface while an excessive oxidation would lead to a higher disulfide bond cleavage and a decrease in surface hydrophobicity of SPI due to leading to the unfolded polypeptide chains refold again. It was found that an over-oxidization could induce the unfolded polypeptide chain to refold again and form insoluble aggregates.[Citation23,Citation24] Thus, when the disulfide bond cleavage was up to 53.29%, the surface hydrophobicity of oxidized-SPI reached the maximum and increased by 114.0% compared with that of native SPI. In addition, it is worth noting that although many hydrophobic residues are buried within native proteins, there are still some hydrophobic groups which may also be retained on the molecular surface or in crevices and contribute to the surface hydrophobicity of native protein.
Figure 3. Changes of the surface hydrophobicity of SPI (0, 0.2%, 0.4%, 1%, 1.4%, 1.8% of peracetic acid, v/v). Different letters on top of a symbol of the line indicate significant (p < 0.05) differences among samples treated under different conditions
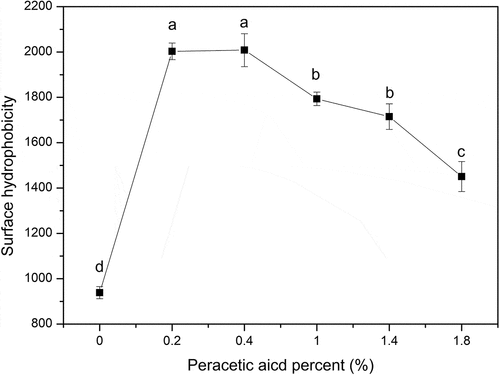
Due to hydrophobicity, the non-polar residues of proteins have a tendency to adhere to one another in an aqueous environment, which leads the polypeptide chains to fold to form a globular molecule. In addition, the molecular structure of globular proteins is usually further stabilized by the intra-chain and/or inter-chain disulfide bonds. Therefore, the structures and properties of globular proteins are obviously affected and restricted by disulfide bonds and hydrophobicity. On one hand, hydrophobic interaction is the driving force for the formation and evolution of globular protein structures. Most of the hydrophobic residues of globular proteins are embedded within the protein molecules to form hydrophobic core, while the polar amino acid residues are distributed on the surface of the protein molecules in hydrophilic environments due to the hydrophobic interaction, which results in that most native protein has poor surface activity due to the lack of sufficient hydrophobicity on the molecular surface. On the other hand, disulfide bonds also play an important role in maintaining the structure and properties of the protein. It is the restriction of the disulfide bond that prevents the hydrophobic groups embedded in the molecule from being exposed to the surface of the molecule and prevents most natural proteins from exhibiting their surface activity. Thus, partially cleaving disulfide bonds may be a good way to change the structure and surface hydrophobicity, which in turn will change proteins’ properties such as gelation, foaming, and emulsifying.
Surface tension and critical micelle concentration (CMC) value
Because of their amphiphilic characteristic, proteins can be adsorbed to oil–water interface, causing a reduction of the interfacial tension. Typically, most native proteins decrease the tension by about 15 to 20mN/m at saturated monolayer coverage; in contrast, the ability of small traditional surfactant to reduce surface tension is stronger, about 30-40mN/m.[Citation25] Although hydrophilic and hydrophobic groups exist in the primary structure of proteins, there are no clear hydrophilic heads and hydrophobic tails in most native proteins. Due to the conformational constraints to properly orient the hydrophilic and hydrophobic groups at the interface and improper packing at the interface, most native proteins cannot significantly reduce the surface tension, which is closely related to their complex structural properties. Therefore, the structural properties of native protein in any case, theoretically, can result in native protein itself possess poor surface properties and it is hard to reduce the surface tension of water below 50mM/m due to their poor surface properties[Citation26], while the modified protein through S-S bond cleavage like the oxidized-SPI in this work exhibits good surface properties because it may reduce surface tension much more than the native SPI does. It could be seen from and that the surface tension of oxidized-SPI decreased more quickly than that of native SPI, and the CMC values of oxidized-SPI were also much smaller than those of native SPI. Among them, the CMC value of sample 2 was only a third of that of native SPI. The surface tensions of sample 2 and 3 also exhibited typical surfactant characteristics. These results suggest that an appropriate peracetic acid oxidation can actually decrease the surface tension of SPI and enhance the surface properties of SPI, which means that some of the oxidized SPI can be used as an ideal precursor for the preparation of protein-based surfactants. It also means that most native proteins can be further modified to improve their surface properties to be used as surfactants.
Table 1. γCMC and CMC values for each group of samples
Foaming capacity and stability
As shown in , similar to the surface hydrophobicity changes, the foaming capacity of oxidized-SPI also increased first and then decreased with the increase of oxidation degree. At a low oxidation level, the foaming capacity of oxidized-SPI increased with the increase of oxidation degree. When the concentration of peracetic acid was 0.4% and the disulfide bond cleavage was 53.29%, the foaming capacity of oxidized-SPI increased to the highest, the foaming capacity of oxidized-SPI reached the maximum, and increased by 81.4%, compared with that of native SPI. However, the foaming stability of SPI increased only slightly with the increase of oxidation degree. The peracetic acid oxidation did not significantly improve the foaming stability of SPI. Excessive oxidation could not effectively improve the foaming capacity and surface hydrophobicity of SPI, even led to the decrease of foaming capacity and surface hydrophobicity of SPI.
Figure 5. Foaming capacity and stability of the SPI with different concentrations of peracetic acid (0, 0.2%, 0.4%, 1%, 1.4%, 1.8%, v/v). Different letters on top of a column indicate significant (p < 0.05) differences among samples treated under different conditions
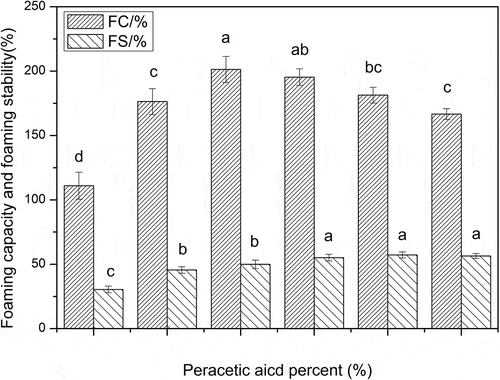
The first condition for a protein molecule to exhibit good surface activity is that it should be able to absorb rapidly at the interface of incompatible two phases; secondly, it should rapidly undergo conformational changes and distribution at the interface and rapidly reduce surface tension; third, it should be able to form a viscoelastic film through intermolecular interactions. According to this suggestion, the first two conditions are essential for better foaming capacity, whereas, the third condition is important for the foaming stability.[Citation27] Although native glycinin has a weak surface activity due to its low surface hydrophobicity, low molecular flexibility and larger molecular size, and the slowest adsorption rate at the interface[Citation28], the foaming capacity of SPI can be greatly improved by an appropriate disulfide bond cleavage. Peracetic acid oxidation did have a significant effect on the molecular structure of SPI, which provided a possibility for improving the surface activity of SPI. An appropriate disulfide bond cleavage can significantly improve the foaming capacity of oxidized SPI. However, excessive oxidation, which induces the unfolded polypeptide chain to refold again and form large aggregates[Citation23,Citation24], may lead to the decrease of foaming capacity in SPI. Furthermore, the peracetic acid oxidation did not significantly improve the foaming stability of SPI. This might also be due to the folding and the formation of large particles, which led to the failure of SPI to form viscoelastic films through intermolecular interactions. The Pearson correlation coefficients for the relationship between surface properties and the surface hydrophobicity of SPI were obtained and listed in , which also showed that surface hydrophobicity of SPI is positively related to its foaming capacity except for the foaming stability. These results mean that foaming stability was not been improved effectively and it may need further modification with other chemical methods on the basis of peracetic acid oxidation to enhance the surface activity of SPI truly.
Table 2. Pearson correlation coefficients among surface properties and the surface hydrophobicity of oxidized-SPI
Emulsification capacity and emulsification stability
shows that the change of emulsifying capacity and emulsifying stability of oxidized SPI is somewhat different from that of foaming ability and stability of oxidized SPI. Due to peracetic acid oxidation, the emulsifying capacity of oxidized SPI was much higher than that of native SPI, while the emulsifying capacity of oxidized SPI samples with different oxidation degrees showed no significant difference. On the contrary, the emulsifying stability of oxidized SPI increased first and then decreased with the increase of oxidation degree, which was similar to the change of foaming capacity of oxidized SPI. At a low oxidation level, the emulsifying stability of oxidized-SPI increased with the increase of oxidation degree. When the concentration of peracetic acid was 0.4% and the disulfide bond cleavage was 53.29%, the emulsifying stability of oxidized-SPI increased to the highest, the emulsifying stability of oxidized-SPI reached the maximum, and increased by 49.8%, compared with that of native SPI. The results of correlation analysis also showed that the surface hydrophobicity of SPI was positively correlated with its emulsifying capacity and emulsifying stability (). The above results indicated that although peracetic acid oxidation could significantly change the emulsifying properties of SPI, the SPI structural changes caused by different peracetic acid concentrations only showed differences in the emulsifying stability of oxidized SPI, but not in the emulsifying capacity of oxidized SPI. This is consistent with the works of Tang et al.[Citation29,Citation30] They found that the conformational flexibility plays a crucial role in different aspects of emulsifying properties; however, the progressive improvement of the emulsifying ability is not related to the initial adsorption rate and amount of adsorbed proteins, but highly dependent on the ease of structural rearrangement of adsorbed proteins at the interface. Because the emulsifying capacity of SPI was measured at the initial stage of emulsification and the emulsifying stability of SPI was determined after 30 min of emulsification, the changes of protein structure caused by different peracetic acid concentration only showed differences in emulsifying stability, but not in emulsifying ability. According to Damodaran’s suggestion, owing to an appropriate peracetic acid oxidation of, some oxidized SPI can not only meet the requirement of forming good emulsifying capacity, but also meet the requirement of forming stable emulsifier.[Citation27] Chen et al.[Citation24] also found that an appropriate oxidation had a positive effect on the emulsifying properties of SPI while over-oxidation can seriously damage its functionalities which are due to the different molecular structures. The emulsifying properties of proteins ultimately depend on a suitable balance between the hydrophile and lipophile, and not just the enhancement of lipophilicity.[Citation31]
Determination of fluorescence spectra
Aromatic amino acids like tryptophan (Trp) can cause proteins to possess some fluorescence characteristics. Since Trp is highly sensitive to the local environment, the conformational changes of protein can be indicated commonly by the fluorescence emission of Trp. Therefore, the average of exposed Trp residues in the aqueous phase affects the maximum emission of protein.[Citation32] shows that all samples had a maximum emission at 340nm and a similar spectral change. Under low levels of oxidization, due to more exposed tryptophan residues in molecules, sample 1–3 showed an increase of fluorescence intensity. It could be observed that the fluorescence intensity of sample 4–6 was weaker than that of native SPI, indicating that the molecular structure of SPI had been changed dramatically due to the oxidative destruction of disulfide bonds, which could be owing to the refolding of unfolded polypeptide chains or the formation of insoluble aggregation. Moreover, the exposed tryptophan residues were buried inside of molecule again, leading to the crease of fluorescence intensity for sample 4–6. Changes in fluorescence intensity of samples may depend on whether tryptophan in protein is exposed to the environment. Because tryptophan or tryptophan residues are easily destroyed by strong oxidants such as organic peroxides[Citation33,Citation34], and at the same time, the exposed tryptophan residues may be buried inside the molecules again due to the refolding of the unfolded polypeptide chains. Therefore, the fluorescence intensity of the sample can reach the maximum under an appropriate oxidation; otherwise, the excessive oxidation will lead to the decrease of the fluorescence intensity of the sample. The results of fluorescence spectroscopy could not show that there was a clear correlation between the changes in SPI molecular structure and surface activity.
Determination of circular dichroism (CD) spectra
The changes in the relative content of secondary structural conformation (α–helix, β-turn and random curling) in protein molecule can reflect changes in the tertiary structure of the protein molecule. Far-UV circular dichroism was used to determine the changes of secondary structure and correspondingly the changes of tertiary structure can be speculated theoretically through the conformational change in secondary structure.[Citation35] The CD spectra and the relative content of secondary structural conformation of native SPI and modified SPI are shown in and . It can be concluded that random curling content decreased all the way, and as the peracetic acid concentration was below 0.4%, the content of α–helix and β-turn for modified SPI increased with the increase of peracetic acid concentration, while excessive oxidation caused the content of α–helix and β-turn to decrease.
Table 3. The content of secondary structural conformation of modified SPI with different peracetic acid percent. The results were expressed as mean ±SD of at least three independent observations
Figure 8. CD spectra of the SPI with different concentrations of peracetic acid (0, 0.2%, 0.4%, 1%, 1.4%, 1.8%, v/v)
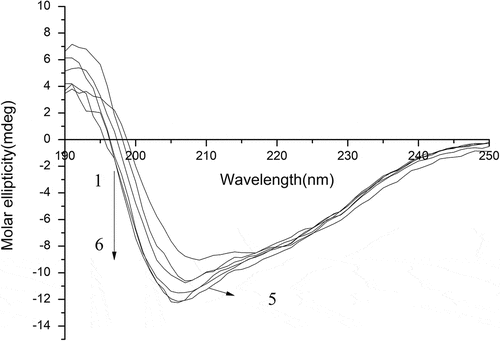
These results indicated that the tertiary structure of oxidation-SPI was gradually extended, and the overall molecular structure loosened and the flexibility increased.[Citation36] Therefore, the internal hydrophobic groups were exposed to the surface of the molecule so as to effectively improve the surface activity of the SPI, and however, excessive oxidation may contribute to the refolding of the unfolded molecular structure of SPI again. The results of CD spectra also could not show that there was a clear correlation between the changes in SPI molecular structure and surface activity.
Measurements of particle size distribution
The mode in measurements of particle size distribution in this paper is intensity. The experimental results () showed that the particle sizes of SPI were changed by peracetic acid oxidation. Native SPI particle sizes were distributed between 0 and 600 nm. With the addition of 0.2% and 0.4% peracetic acid, the particle sizes of SPI were mainly distributed between 90 and 230 nm, and the particle size became obviously smaller. This was due to the oxidation by peracetic acid that caused the breakage of the disulfide bonds in the protein molecule, resulting in the disaggregation of the protein molecules, which will produce small particles of soluble protein, and cause the subunit composition of the protein to change. The particle sizes seen in oxidized SPI with the addition of 1% and 1.4% of peracetic acid were mainly distributed between 100 and 550 nm, indicating that excessive oxidation broke part of the peptide chain and increased the formation of insoluble protein components. The particle size distribution of oxidized-SPI (1.8% of peracetic acid) was significantly broadened. These results indicated that in addition to small particles, large particles also increased due to excessive oxidation. These results above showed that in addition to making protein granules smaller, excessive oxidation also made protein granules become large particles. The results of particle size analysis also could not show that there was a clear correlation between the changes in SPI molecular structure and surface activity.
Conclusion
This study showed that peracetic acid oxidation was an effective method for improving the surface hydrophobicity and surface properties of proteins. The surface properties of SPI were markedly improved by an appropriate disulfide bond cleavage resulted from peracetic acid oxidation. The results of fluorescence spectra, CD spectra, and particle size indicated that the molecular structures of SPI did have been changed dramatically due to the oxidative destruction of disulfide bonds, but there was no clear correlation between the molecular structural change and the surface activity of SPI. Peracetic acid oxidization exhibited a notable influence on surface hydrophobicity of SPI. The surface hydrophobicity of oxidized-SPI increased gradually at the initial stage with a lower disulfide bond cleavage. However, an excessive oxidation would lead to a higher disulfide bond cleavage and a decrease in surface hydrophobicity of SPI. An appropriate peracetic acid oxidation could decrease the surface tension of SPI more quickly. Similar to the changes in surface hydrophobicity, the foaming capacity and emulsifying stability of oxidized SPI also increased first and then decreased with the increase of oxidation degree. Excessive oxidation led to a decrease in foaming capacity, emulsifying stability and surface hydrophobicity of SPI, but it had no obvious effect on the foaming stability and emulsifying capacity of SPI, and the foaming stability and emulsifying capacity of oxidized-SPI increased only slightly with the increase of oxidation degree. These results would be useful for better understanding the structure dependence of protein surface behaviors and expanding the utilization of SPI.
Acknowledgments
This research was financially supported by National Natural Science Foundation of China (grant number 21466006, grant number 31660473). The authors declare that there is no conflict of interest regarding the publication of this paper.
Additional information
Funding
References
- O‘Sullivan, J.; Murray, B.; Flynn, C.; Norton, I. The Effect of Ultrasound Treatment on the Structural, Physical and Emulsifying Properties of Animal and Vegetable Proteins. Food Hydrocolloids. 2016, 53, 141–154. DOI: 10.1016/j.foodhyd.2015.02.009.
- Sung, H. Y.; Chen, H. J.; Liu, T. Y.; Jong-Ching, S. U. Improvement of the Functionalities of Soy Protein Isolate through Chemical Phosphorylation. J. Food Sci. 2010, 48, 716–721. DOI: 10.1111/j.1365-2621.1983.tb14882.x.
- Segat, A.; Misra, N. N.; Fabbro, A.; Buchini, F.; Lippe, G.; Cullen, P. J.; Innocente, N. Effects of Ozone Processing on Chemical, Structural and Functional Properties of Whey Protein Isolate. Food Res. Int. 2014, 66, 365–372. DOI: 10.1016/j.foodres.2014.10.002.
- Cardamone, M.; Puri, N. K. Spectrofluorimetric Assessment of the Surface Hydrophobicity of Proteins. Biochem. J. 1992, 282, 589–593. DOI: 10.1042/bj2820589.
- Banach, M.; Kalinowska, B.; Konieczny, L.; Roterman, I. Role of Disulfide Bonds in Stabilizing the Conformation of Selected Enzymes—An Approach Based on Divergence Entropy Applied to the Structure of Hydrophobic Core in Proteins. Entropy. 2016, 18, 1–21. DOI: 10.3390/e18030067.
- Song, F.; Tang, D. L.; Wang, X. L.; Wang, Y. Z. Biodegradable Soy Protein Isolate-Based Materials: A Review. Biomacromolecules. 2011, 12, 3369–3380. DOI: 10.1021/bm200904x.
- Nishinari, K.; Fang, Y.; Guo, S.; Phillips, G. O. Soy Proteins: A Review on Composition, Aggregation and Emulsification. Food Hydrocolloids. 2014, 39, 301–318. DOI: 10.1016/j.foodhyd.2014.01.013.
- Ferrersueta, G.; Manta, B.; Botti, H.; Radi, R.; Trujillo, M.; Denicola, A. Factors Affecting Protein Thiol Reactivity and Specificity in Peroxide Reduction. Chem. Res. Toxicol. 2011, 24, 434–450. DOI: 10.1021/tx100413v.
- Trujillo, M.; Alvarez, B.; Radi, R. One- and Two-Electron Oxidation of Thiols: Mechanisms, Kinetics and Biological Fates. Free Radical Res. 2015, 50, 150–171. DOI: 10.3109/10715762.2015.1089988.
- Karimi, M.; Ignasiak, M. T.; Chan, B.; Croft, A. K.; Radom, L.; Schiesser, C. H.; Pattison, D. I.; Davies, M. J. Reactivity of Disulfide Bonds is Markedly Affected by Structure and Environment: Implications for Protein Modification and Stability. Sci. Rep. 2016, 6, 1–12. DOI: 10.1038/srep38572.
- Pan, G. X.; Spencer, L.; Leary, G. J. Reactivity of Ferulic Acid and Its Derivatives toward Hydrogen Peroxide and Peracetic Acid. J. Agric. Food Chem. 1999, 47, 3325–3331. DOI: 10.1021/jf9902494.
- Wang, Y. W.; Liao, M. S.; Shu, C. M. Thermal Hazards of a Green Antimicrobial Peracetic Acid Combining DSC Calorimeter with Thermal Analysis Equations. J. Therm. Anal. Calorim. 2015, 119, 2257–2267. DOI: 10.1007/s10973-014-4369-z.
- Samoto, M.; Maebuchi, M.; Miyazaki, C.; Kugitani, H.; Kohno, M.; Hirotsuka, M.; Kito, M. Abundant Proteins Associated with Lecithin in Soy Protein Isolate. Food Chem. 2007, 102, 317–322. DOI: 10.1016/j.foodchem.2006.05.054.
- Ellman, G. L.;. Tissue Sulfhydryl Groups. Arch. Biochem. Biophys. 1959, 82, 70–77. DOI: 10.1016/0003-9861(59)90090-6.
- Hayakawa, S.; Nakai, S. Relationships of Hydrophobicity and Net Charge to the Solubility of Milk and Soy Proteins. J. Food Sci. 1985, 50, 486–491. DOI: 10.1111/j.1365-2621.1985.tb13433.x.
- Sedev, R.;. Surface Tension, Interfacial Tension and Contact Angles of Ionic Liquids. Curr. Opin. Colloid Interface Sci. 2011, 16, 310–316. DOI: 10.1016/j.cocis.2011.01.011.
- Wei, W.; Li, J.; Yan, L.; Huang, G.; Zhen, D. Effect of Oxidization and Chitosan on the Surface Activity of Soy Protein Isolate. Carbohydr. Polym. 2016, 151, 700–706. DOI: 10.1016/j.carbpol.2016.06.004.
- Wang, W.; Li, J. S.; Yan, L. J.; Huang, G. X.; Dong, Z. Effect of Oxidization and Chitosan on the Surface Activity of Soy Protein Isolate. Carbohydr. Polym. 2016, 151, 700–706. DOI: 10.1016/j.carbpol.2016.06.004.
- Sanger, F.;. Oxidation of Insulin by Performic Acid. Nature. 1947, 160, 295. DOI: 10.1038/160295b0.
- Hirs, C. H.;. The Oxidation of Ribonuclease with Performic Acid. J. Biol. Chem. 1956, 219, 611.
- Liu, S. H.; Zhao, L. Z.; H, W. D. Study of the Oxidation Mechanism of Disulfide Bonds, Sulfhydryl Groups and Melanin in Human Epidermal Skin by X-Ray Photoelectron Spectroscopy. Acta Chim. Sinica. 1983, 41, 716–721. DOI: 10.1021/ar00096a006.
- Kalapathy, U.; Hettiarachchy, N. S.; Rhee, K. C. Effect of Drying Methods on Molecular Properties and Functionalities of Disulfide Bond-Cleaved Soy Proteins. J. Am. Oil Chem. Soc. 1994, 74, 195–199. DOI: 10.1007/s11746-997-0123-z.
- Kella, N. K. D.; Barbeau, W. E.; Kinsella, J. E. Effect of Disulfide Bond Cleavage on the Structure and Conformation of Glycinin. Int. J. Pept. Protein Res. 1986, 27, 421–432. DOI: 10.1111/j.1399-3011.1986.tb01037.x.
- Chen, N.; Zhao, M.; Sun, W. Z.; Ren, J. Y.; Cui, C. Effect of Oxidation on the Emulsifying Properties of Soy Protein Isolate. Food Res. Int. 2013, 52, 26–32. DOI: 10.1016/j.foodres.2013.02.028.
- Razumovsky, L.; Damodaran, S. Surface Activity−Compressibility Relationship of Proteins at the Air−Water Interface. Langmuir. 1999, 15, 1392–1399. DOI: 10.1021/la980873v.
- Damodaran, S.;. Adsorbed Layers Formed from Mixtures of Proteins. Curr. Opin. Colloid Interface Sci. 2005, 9, 328–339. DOI: 10.1016/j.cocis.2004.09.008.
- Damodaran, S.;. Protein-Stabilized Foams and Emulsions, In: Food Proteins and Their Applications. Damodaran, S., Paraf, A., Eds.; Marcel Dekker: New York, 1997; pp. 57–110.
- Medrano, A.; Abirached, C.; Araujo, A. C.; Panizzolo, L. A.; Moyna, P.; Añón, M. C. Correlation of Average Hydrophobicity, Water/Air Interface Surface Rheological Properties and Foaming Properties of Proteins. Food Sci. Technol. Int. 2012, 18, 187–193. DOI: 10.1177/1082013211415137.
- Chuan-He, T.; Lan, S. Role of Conformational Flexibility in the Emulsifying Properties of Bovine Serum Albumin. J. Agric. Food Chem. 2013, 61, 3097–3110. DOI: 10.1021/jf305471k.
- Han-Ni, L.; Chuan-He, T. Emulsifying and Interfacial Properties of Vicilins: Role of Conformational Flexibility at Quaternary And/Or Tertiary Levels. J. Agric. Food Chem. 2013, 61, 11140–11150. DOI: 10.1021/jf403847k.
- Aoki, H.; Taneyama, O.; Orimo, N.; Kitagawa, I. Effect of Lipophilization of Soy Protein on Its Emulsion Stabilizing Properties. J. Food Sci.. 2010, 46, 1192–1195. DOI: 10.1111/j.1365-2621.1981.tb03021.x.
- Kerensa, B.; Voragen, A. G. J.; Hamer, R. J.; De Jongh, H. H. J. Glycoforms of Beta-Lactoglobulin with Improved Thermostability and Preserved Structural Packing. Biotechnol. Bioeng. 2004, 86, 78–87. DOI: 10.1002/bit.20030.
- Simat, T.; Meyer, K.; Stöver, B.; Steinhart, H. Oxidation of Free and Peptide Bound Tryptophan. Adv. Exp. Med. Biol. 1996, 398, 655–659. DOI: 10.1007/978-1-4613-0381-7_106.
- Simat, T. J.; Steinhart, H. Oxidation of Free Tryptophan and Tryptophan Residues in Peptides and Proteins. J. Agric. Food Chem. 1998, 46, 490–498. DOI: 10.1021/jf970818c.
- Jiang, L.; Wang, Z.; Li, Y.; Meng, X.; Sui, X.; Qi, B.; Zhou, L. Relationship between Surface Hydrophobicity and Structure of Soy Protein Isolate Subjected to Different Ionic Strength. Int. J. Food Prop. 2015, 18, 1059–1074. DOI: 10.1080/10942912.2013.865057.
- Kanehisa, M. I.; Tsong, T. Y. Local Hydrophobicity Stabilizes Secondary Structures in Proteins. Biopolymers. 1980, 19, 1617–1628. DOI: 10.1002/bip.1980.360190906.