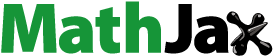
ABSTRACT
This study investigated the effect of milk oligopeptides and (α)-tocopherol on inhibition of linoleic acid oxidation using Fe2+-vitamin C induced linoleic acid oxidation model through analysis of malondialdehyde, peroxide value, and conjugated diene and triene in the model. The alteration of milk oligopeptides maximal absorption wavelength, fluorescent feature, and secondary structure were further investigated to elucidate the interactions between milk oligopeptide and (α)-tocopherol that altered the inhibitory effect of linoleic acid oxidation. Results showed that Pro-Tyr-Tyr-Ala-Lys (PYYAK) and Ile-Pro-Ile-Gln-Tyr (IPIQY) with (α)-tocopherol significantly inhibited the oxidation of linoleic acid and reduced the formation of malondialdehyde by 38% and 41%, respectively. Additionally, Ile-Pro-Ile-Gln-Tyr-Val (IPIQYV) and (α)-tocopherol synergistically reduced the peroxide value in the model by 36.8%. Milk oligopeptides exhibited a blue shift on its maximal absorption wavelength, and their absorbance value decreased with the increase of the (α)-tocopherol concentration. The fluorescent intensity of milk oligopeptides was reduced with the addition of (α)-tocopherol and such fluorescent intensity reductions resulted from the static quenching process through the formation of milk oligopeptide-(α)-tocopherol complex. Fourier transform infrared spectroscopy analysis revealed that (α)-tocopherol significantly altered the secondary structure of milk oligopeptides and the percentage of β-turn obviously increased in milk oligopeptide-(α)-tocopherol complex. These indicated that the inhibition of linoleic acid oxidation might result from complex formed between milk oligopeptide and (α)-tocopherol through inter-molecular interactions.
Introduction
Linoleic acid is one of the most important essential fatty acids that play vital roles in the human health.[Citation1] Linoleic acid mainly exists in edible oils made of soybean, peanut, sunflower, and corn, and it is considered a critical component in infant formula and other food products.[Citation2] Basically, linoleic acid consists of 18 carbons in a chain with 2 double bonds under a cis-conformation.[Citation2]Linoleic acid is highly sensitive to multiple environmental factors, including light, heat, and oxygen, and easily oxidized to form oxidized products, which could result in the spoilage of the food products and induce potential health concern for human.[Citation3,Citation4] Some synthesized antioxidants, such as butylated hydroxyanisole and tertiary butylhydroquinone, have been implemented in the linoleic acid-rich foods to prevent the oxidation of linoleic acid. However, these synthesized antioxidants have been paid more attention by customers on its potential health risk.[Citation5] (α)-Tocopherol, also known as vitamin E, is a natural antioxidant widely present in vegetable oil and it has been considered a best antioxidant that could protect linoleic acid from degradation.[Citation6] However, recent studies have suggested that thermal treatment, high level of metal ions, and oil spoilage could facilitate the oxidation of (α)-tocopherol, which could further induce the oxidation of linoleic acid in the food products.[Citation7]
Antioxidant peptides have gained much more attention in the field of food and nutritional sciences. It has been reported that antioxidant peptides and their hydrolytes in milk, soybean, egg, poultry, and seafood exhibited a high level of antioxidative capacities.[Citation8,Citation9] Milk oligopeptides, a major hydrolyte group released from the casein and whey protein hydrolysis, have been extensively studied due to their multiple functional features.[Citation10] For example, Nielsen et al. have reported that milk oligopeptides with bigger molecular weight possessed strong inhibitory activity on the oxidation of the ω-3 polyunsaturated fatty acids.[Citation11] It has also been reported that polyunsaturated fatty acids fortified milk supplemented with oligopeptides exhibited high stability against the fatty acid oxidation.[Citation12-Citation14] Some milk oligopeptides, such as Tyr-Phe-Tyr-Pro-Glue-Leu, Val-Lys-Glu-Ala-Met-Ala-Pro-Lys, and Ala-Val-Pro-Tyr-Pro-Gln-Arg, have been isolated from the milk protein hydrolytes.[Citation15,Citation16] However, the application of these isolated milk oligopeptides to prevent the lipid oxidation has not been fully investigated to our best knowledge.
Previous studies have reported that proteins and their hydrolyzed peptides could interact with (α)-tocopherol through non-covalent bonds (such as electrostatic and hydrophobic interactions) in soybean oil. Such interactions could alter the tertiary conformation of oligopeptides (Leu-Gln-His-Lys), which resulted in an improvement on prevention of the linoleic acid oxidation.[Citation17-Citation20] Tironi et al. have also reported that the protein hydrolytes from chives, with the presence of (α)-tocopherol, could decrease the conversion rate on secondary oxidation products in Rapeseed oil, and such an inhibitory effect was not affected by temperature.[Citation9] It has been known that the antioxidant capacity of oligopeptides is essentially determined by its amino acid sequences and its molecular weight.[Citation21] However, other components in foods could also impact their antioxidant property. For instance, a significant improvement in the antioxidant activity in foods was found due to the synergetic effect caused by an oligopeptide (Tyr-His-Tyr) and phenolic compounds.[Citation22] In our previous study, we have identified the antioxidant properties of multiple milk oligopeptides isolated from fermented yogurt.[Citation23] In this study, we further applied these milk oligopeptides to the linoleic acid oxidation model with or without the presence of (α)-tocopherol. The objective of this study was to investigate if milk oligopeptides could effectively inhibit the oxidation of linoleic acid with (α)-tocopherol, and further to elucidate the interactions between oligopeptides and (α)-tocopherol that could improve the stability of linoleic acid. The findings from this study could provide vital information on quality development on infant formula and other linoleic acid-rich food products.
Materials and methods
Chemicals and reagents
Milk oligopeptides used in the present study included Ile-Ala-Lys-Tyr-Ile (IAKYI), Pro-Tyr-Tyr-Ala-Lys (PYYAK), Asn-Gln-Phe-Leu-Pro-Tyr-Pro-Tyr-Tyr-Ala-Lys (NQFLPYPYYAK), Ile-Pro-Ile-Gln-Tyr (IPIQY), and Ile-Pro-Ile-Gln-Tyr-Val (IPIQYV). These oligopeptides were purchased from Zhejiang Ontores Biotech Co. Ltd with a purity >99% (Zhejiang, China). (α)-Tocopherol, linoleic acid, and 1,1,3,3-tetraoxy ethyl propane, with a purity of 96%, 99% and 99%, respectively, were purchased from Sigma-Aldric (Milwaukee, WI, USA). The other reagents and chemicals were of analytical grade and purchased from Huadong Medicine Co. Ltd. (Zhejiang, China) unless specifically noted.
Fe2+-vitamin c induced linoleic acid oxidation model
The ferrous ion-vitamin C induced linoleic acid oxidation model was adopted from the previously published methods.[Citation24,Citation25] The model was further optimized in the present study in terms of the ferrous ion concentration and oxidation duration under a simple factor experiment. Briefly, 1.0 mL 0.4 mol/L linoleic acid in dimethyl sulfoxide (DMSO) solution was well mixed with 2.0 mL 0.2 mol/L acetic acid-sodium acetate buffer solution (pH 5.4). To optimize the concentration of the ferrous solution, the mixture was mixed with 1.0 mL of the FeSO4 solution (20–60 mmol/L) and 1.0 mL of 16 mmol/L vitamin C solution. The resultant mixture was incorporated with 2.0 mL water and then incubated in a 30°C water bath for 60 min under an oscillation. For the optimization of the oxidation duration, the mixture was mixed with 1.0 mL of 40 mmol/L FeSO4 solution and 1.0 mL of 16 mmol/L vitamin C solution. The resultant mixture was mixed with 2.0 mL of water and then incubated in the 30°C water bath for a certain time (15–120 min) under the same oscillation. After the incubation, the resultant mixture (7.0 mL) was stored at −20°C prior to the further analyses. Each experiment was carried out in triplicate.
To investigate the effect of (α)-tocopherol on the linoleic acid oxidation model, the mixture (1.0 mL 0.4 mol/L linoleic acid in DMSO and 2.0 mL 0.2 mol/L acetic acid-sodium acetate buffer, pH 5.4) was mixed with 1.0 mL of 40 mmol/L FeSO4 solution and 1.0 mL of 16 mmol/L vitamin C solution. The resultant mixture was mixed with 1.0 mL of the (α)-tocopherol solution with different concentrations (0–0.1 mmol/Lin DMSO) and 1.0 mL of water and then incubated in the 30°C water bath for 60 min under the same oscillation. The resultant mixture after the incubation was stored at −20°C prior to the further analyses. Each experiment was carried out in triplicate.
To study the effect of milk oligopeptides on the linoleic acid oxidation model, the mixture was mixed with 1.0 mL of 40 mmol/L FeSO4 solution and 1.0 mL of 16 mmol/L vitamin C solution. The resultant mixture was mixed with 1.0 mL of 15 mmol/L milk oligopeptide in water (IAKYI, PYYAK, NQFLPYPYYAK, IPIQY or IPIQYV) and 1.0 mL of water, and then incubated in the 30°C water bath for 60 min under the same oscillation. The resultant mixture after the incubation was stored at −20°C prior to the further analyses. Each experiment was carried out in triplicate.
For the combined effect of (α)-tocopherol and milk oligopeptide on the linoleic acid oxidation, the mixture was mixed with 1.0 mL of 40 mmol/L FeSO4 solution and 1.0 mL of 16 mmol/L vitamin C solution. The resultant mixture was mixed with 1.0 mL of 0.046 mmol/L (α)-tocopherol solution and 1.0 mL of 15 mmol/L milk oligopeptide (IAKYI, PYYAK, NQFLPYPYYAK, IPIQY or IPIQYV), and then incubated in the 30°C water bath for 60 min under the same oscillation. The resultant mixture after the incubation was stored at −20°C prior to the further analyses. Each experiment was carried out in triplicate.
Malondialdehyde measurement
The analysis of malondialdehyde in the oxidized linoleic acid model was carried out using the thiobarbituric (TBA) method with minor modifications.[Citation26] In brief, the oxidized linoleic acid mixture (7.0 mL) was mixed with 12.0 mL of 0.5% TBA (w/v). The resultant mixture was vortexed and then boiled for 15 min. Afterward, the mixture was cooled to the room temperature and then mixed with 5.0 mL N-butanol. Then, the resulting mixture was well vortexed and kept at the room temperature for 20 min for the phases to be separated. The N-butanol phase was collected through separatory funnel and then mixed with 0.5 g anhydrous sodium sulfate. The mixture was centrifuged at 2,800 × g for 15 min to collect the supernatant. The absorbance of the supernatant was measured at 532 nm on a UNICO-2102PC UV-VIS spectrophotometer (Dayton, NJ, USA). 1,1,3,3-Tetraoxyethylpropane was used as the external standard to calculate the malondialdehyde content. Each measurement was carried out in triplicate.
Peroxide value analysis
Peroxide value of the oxidized linoleic acid model was analyzed using the International Dairy Federation Standard Method with minor modifications.[Citation27] The oxidized linoleic acid mixture (7.0 mL) was mixed with 5.0 mL of chloroform: methanol (2:1, v/v) and 0.3 mL of 30% ammonium thiocyanate (w/v) solution. The resultant mixture was vortexed and then kept at the room temperature for 20 min. Subsequently, the absorbance of the mixture was measured at 500 nm on the same spectrophotometer and marked as the peroxide value. As water (7.0 mL) instead of the oxidized linoleic acid mixture, the absorbance of the resultant sample was measured under the same protocol and used as the blank. Each measurement was performed in triplicate.
Measurement of conjugated diene and triene
The measurement of the conjugated diene and triene followed the published methods with minor modifications.[Citation28,Citation29] Briefly, the oxidized linoleic acid mixture was extracted against 5.0 mL cyclohexane solution. The mixture was kept at the room temperature for 20 min for the phases to be separated. The upper phase (the cyclohexane phase) was collected and then further diluted using the cyclohexane solution. The diluted solution was measured at 237 nm and 272 nm on a UNICO-2102PC UV-VIS spectrophotometer (Dayton, NJ, USA) for conjugated diene (A1) and triene (A2), respectively. The cyclohexane solution was used as a blank. Each measurement was performed in triplicate. The values of the conjugated diene and triene in the linoleic acid model were calculated using the equations below,
Conjugated Diene = A1/b × c × 115
Conjugated Triene = A2/b × c × 920
where A1 and A2 were the absorbances of the diluted solution at 237 nm and 272 nm, whereas b and c represented the cuvette length and sample concentration. Absorbance coefficient 115 and 920 indicated the coefficient for the conjugated diene and triene, respectively.
Uv-vis spectrophotometry
The absorbance spectrum of the milk oligopeptide/(α)-tocopherol mixture was recorded using a SpectraMax 190 Microplate Reader (SPW Industrial, Laguna Hills, CA, USA). Milk oligopeptide (IAKYI, PYYAK, NQFLPYPYYAK, IPIQY or IPIQYV) was dissolved in 0.2 mol/L acetic acid-sodium acetate buffer solution (pH 5.4) to a concentration of 2.5 mmol/L. The milk oligopeptide solution was mixed with different (α)-tocopherol concentration (0, 0.05, 0.10, 0.20 and 0.30 mmol/L in DMSO). The mixture was kept at the room temperature for 5 min and then recorded the absorbance spectrum under a wavelength range from 270 nm to 320 nm. DMSO was used as the blank. The measurement was conducted in triplicate.
Fluorescence spectroscopy
The tertiary structural alteration of the milk oligopeptides with the presence of (α)-tocopherol was measured using fluorescence spectroscopy on a BioTek Synergy 4 (BioTek Instruments Inc., Winooski, VT, USA).[Citation30]Each milk oligopeptide was dissolved in 0.2 mol/L acetic acid-sodium acetate buffer solution (pH 5.4) to a concentration of 0.1 µmol/L, and further mixed with different (α)-tocopherol concentration (0, 0.005, 0.01, 0.015, 0.02 and 0.025 nmol/L in DMSO, respectively). The mixed solution was then kept at the room temperature for 3 min and then analyzed on fluorescence spectroscopy at 25°C. The excitation wavelength of the mixed solution was set at 280 nm and emission spectra were recorded from 300 nm to 500 nm with a resolution of 2.5 nm. DMSO was used as the reference and each experiment was carried out in triplicate.
Fourier transform infrared spectroscopy (FTIR)
The milk oligopeptide solution (0.3 mol/L) and the milk oligopeptide and (α)-tocopherol mixed solution under a molar ratio of 1:1 were freeze-dried. The freeze-dried sample (1 mg) was mixed with 100 mg of pure KBr. The resultant mixture was ground into a fine powder and then analyzed on a Thermo Nicolet Nexus 470 FTIR (Thermo Nicolet, Madison, WI, USA). Pure KBr powder was used as the background. The FTIR spectra of the sample were obtained over a wave number range of 1,800 to 1,400 cm−1 with a resolution of 4 cm−1 and a wave number accuracy at 0.01 cm−1. Each signal was scanned at 32 times and each measurement was carried out in triplicate. The FTIR spectrum was calibrated under the amide band I (1,700–1,600 cm−1) using PeakFit Version 4.12 software (Chicago, IL, USA) and then further deconvolution to fit in Gaussian regression based on the second derivative spectra for determination of the percentage of secondary structures.
Statistical analysis
Data were expressed as the mean ± standard deviation of triplicate tests. One-way analysis of variance (ANOVA) was used to determine the significant differences between the means under Tukey Test at a 0.05 significant level using Origin Pro 7.5 (OriginLabCorp., Wellesley Hills, MA, USA).
Results and discussion
Optimization of Fe2+-vitamin c-induced linoleic acid oxidation model
In the linoleic acid oxidation model, ferrous ion (Fe2+) was a critical component to initiate the radical chain reaction. Meanwhile, vitamin C in the model could reduce the oxidized state Fe3+ back to ferrous ion, causing the oxidation of linoleic acid to yield malondialdehyde.[Citation25] In the present study, we investigated the effect of both ferrous ion concentration and oxidation duration on the oxidation of linoleic acid in the model (). When the oxidation duration of the linoleic acid model was fixed at 60 min, increasing the ferrous ion from 20 mmol/L to 40 mmol/L in the model significantly elevated the yield of malondialdehyde in the model (). The malondialdehyde level in the model with the ferrous ion concentration at 60 mmol/L appeared to be as similar as that treated with the 40 mmol/L ferrous ion concentration. Our result was consistent with the previously published study.[Citation25]The optimal ferrous ion concentration used in the linoleic acid oxidation model was selected to be 40 mmol/L. Regarding the effect of oxidation duration, the oxidation model was conducted under the ferrous ion concentration at 40 mmol/L (). Incubating the linoleic acid model for 15 min and 30 min did not significantly result in the formation of malondialdehyde (less than 1 µg). However, a dramatic increase in the malondialdehyde level was observed in the linoleic acid oxidation model after 60 min of the oxidation duration. The extension of the oxidation duration to 120 min still increased the yield of malondialdehyde in the model. However, the malondialdehyde accumulation rate was not as rapid as that in the model with 60 min of the oxidation. Therefore, the oxidation duration of the model was set at 60 min.
Figure 1. Effect of ferrous ion concentration and oxidation duration on the formation of malondialdehyde in Fe2+-vitamin C-induced linoleic acid oxidation model. (a) Ferrous ion concentration and (b) oxidation duration. Data are the mean ± standard deviation of triplicate tests. Different letters represent significant difference in the means at a significant level of 0.05
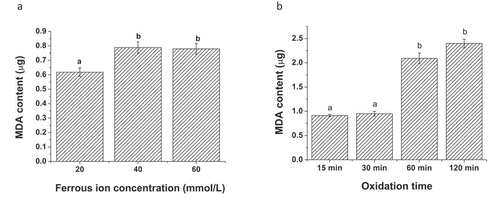
Effect of (α)-tocopherol, milk oligopeptide, and milk oligopeptide-(α)-tocopherol on linoleic acid oxidation model
It was accepted that (α)-tocopherol played an important role in regulating the oxidation of linoleic acid, and its concentration significantly determined the linoleic acid oxidation rate in the oil-rich foods.[Citation7] It has been reported that the oxidation of linoleic acid could be inhibited with low concentration of (α)-tocopherol, whereas high level of (α)-tocopherol could dramatically accelerate the linoleic acid oxidation. This process was illustrated in the following equations.[Citation31]
where ,
,
,
, LH, and LOOH represent (α)-tocopherol, lipid peroxyl radicals, nonradical products, (α)-tocopheroxyl radicals, active bisallylic methylene groups in polyunsaturated fatty acids and peroxidation products, respectively. When (α)-tocopherol was present in oil in a low concentration level, (α)-tocopherol could rapidly interact with lipid peroxyl radicals to form the nonradical products. Such reactions could further cleave the lipid radical chain reaction.[Citation32] However, (α)-tocopherol with high concentration in the model could result in a dramatic accumulation of (α)-tocopheroxyl radicals. Meanwhile, no enough lipid peroxyl radicals were available to interact with (α)-tocopheroxyl radicals to form the nonradical products. The extensive (α)-tocopheroxyl radicals could further react with active bisallylic methylene groups in polyunsaturated fatty acids. As a result, (α)-tocopheroxyl radicals were reduced back to (α)-tocopherol and lipid peroxyl radicals and peroxidation products were formed.[Citation32] This could enhance the oxidation of lipids.[Citation31] In the present study, with the increase of the (α)-tocopherol concentration from 0 to 0.023 mmol/L in , the accumulation of malondialdehyde showed a significant reduction in the linoleic acid oxidation system (p < .05), which indicated that (α)-tocopherol at this concentration range reduced the oxidation of linoleic acid. However, an increase on malondialdehyde was found in the model when the concentration of (α)-tocopherol was above 0.046 mmol/L. Therefore, the best (α)-tocopherol level in the linoleic acid oxidation model was 0.023 mmol/L.
Figure 2. Effect of (α)-tocopherol on inhibition of linoleic acid oxidation in Fe2+-vitamin C induced linoleic acid oxidation model. Data are the mean ± standard deviation of triplicate tests
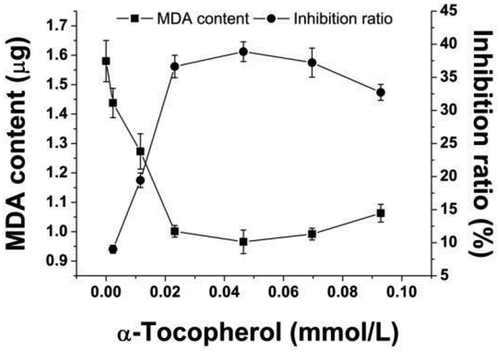
To better elucidate the synergetic effect of milk oligopeptide and (α)-tocopherol on regulating the oxidation of linoleic acid in the model, individual milk oligopeptide (15 mmol/L) was introduced to the Fe2+-vitamin C induced linoleic acid oxidation model and compared with the model treated with 15 mmol/L milk oligopeptide and 0.023 mmol/L (α)-tocopherol. shows the formation of malondialdehyde in the model. It was observed that the individual milk oligopeptides, including IAKYI, NQFLPYPYYAK, IPIQY, and IPIQYV, significantly reduced the yield of malondialdehyde in the model compared to the control (p < .05). However, the combination of (α)-tocopherol with NQFLPYPYYAK or IPIQYV did not significantly improve the inhibitory effect on the linoleic acid oxidation in the model. It should be worth noting that the model treated with (α)-tocopherol and IAKYI even exhibited an increase in the formation of malondialdehyde in the model, indicating that a negatively synergetic effect was established between (α)-tocopherol and IAKYI on the inhibition of the linoleic acid oxidation. On the contrast, the incorporation of (α)-tocopherol with the milk oligopeptide PYYAK or IPIQY further inhibited the oxidation of linoleic acid, resulting in a low level of malondialdehyde in the model (). It has been reported that Tyr-His-Tyr, Tyr-Lys-Tyr, and Tyr-Arg-Tyr could only exert a positively synergetic effect with (δ)-tocopherol rather than (α)-tocopherol on the inhibition of the linoleic acid oxidation.[Citation22] Valeria et al. also reported that different synergetic effects were established between different protein hydrolyzates and tocopherol on the inhibition of lipid oxidation, and therefore suggested that it might be the difference on the interaction between peptides and tocopherol that caused the difference on inhibiting lipid oxidation.[Citation9]
Figure 3. Effect of milk oligopeptides and milk oligopeptides with optimal (α)-tocopherol concentration on inhibition of linoleic acid oxidation in Fe2+-vitamin C-induced linoleic acid oxidation model. (a) Malondialdehyde, (b) Peroxide value and (c) Conjugated diene and triene. Data are the mean ± standard deviation of triplicate tests. # represents significant difference between milk oligopeptides, (α)-tocopherol, milk oligopeptide-(α)-tocopherol and the control on the means at a significant level of 0.05. * means significant difference between milk oligopeptides, milk oligopeptide-(α)-tocopherol and (α)-tocopherol on the means at a significant level of 0.05
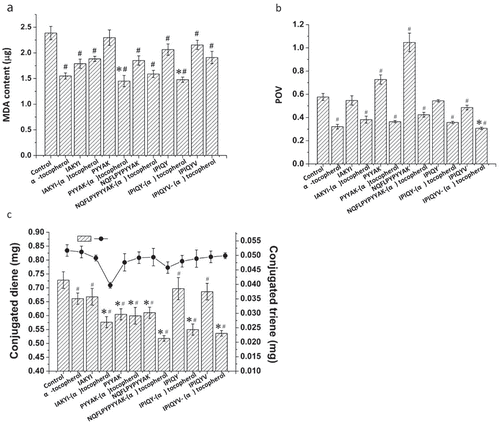
, exhibit the peroxide value and conjugated diene and triene in the linoleic acid oxidation model, respectively. It was found that the milk oligopeptide IPIQYV significantly inhibited the peroxide value in the model compared to the control, whereas PYYAK and NQFLPYPYYAK accelerated the process of the conversion from linoleic acid to its peroxides. It should be noted that the combination of IPIQYV and (α)-tocopherol significantly inhibited the peroxide value of the model compared to the model treated only by IPIQYV or (α)-tocopherol (). Additionally, the introduction of the milk oligopeptide, including IAKYI, NQFLPYPYYAK, IPIQY, IPIQYV, and PYYAK, lowered the formation rate of conjugated diene and triene in the model compared to the control. Moreover, an obviously positive synergetic effect was found between these milk oligopeptides and (α)-tocopherol on inhibiting the release of conjugated diene and triene (). These indicated that these milk oligopeptides might not play an essential role in inhibiting the formation of hydroperoxides, the initial product of linoleic acid oxidation. Hydroperoxides could further be converted into conjugated diene and triene through conformation and unsaturated bonds rearrangement.[Citation33,Citation34] However, milk oligopeptides could exert a critical effect on inhibiting the formation of conjugated diene and triene and the inhibitory effect was mainly depended on the amino acid components and sequence of the oligopeptides.[Citation10] It should be noted that the interaction between milk oligopeptide and (α)-tocopherol could also alter the inhibitory effect of the linoleic acid oxidation.[Citation35] We further investigated the interaction between these oligopeptide and (α)-tocopherol.
Interaction between milk oligopeptide and (α)-tocopherol
UV-Vis Spectrometry: UV-Vis spectrometry is widely used to evaluate the conformation alteration of proteins and/or peptides.[Citation36-Citation38] Essentially, tyrosine as one of aromatic amino acids can be absorbed at the wavelength of 280 nm. Any interaction of peptides containing tyrosine residues with other molecules could change the conformation of the peptides structure. Such conformational alterations could result in a shift on the maximal absorption wavelength of peptides.[Citation37] In the present study, these milk oligopeptides exhibited a maximal absorption at 290 nm in UV-Vis spectra (), and this maximal wavelength absorption was mainly attributed to the π-π* transition of the aromatic amino acids in these oligopeptides.[Citation39] The increase of the (α)-tocopherol concentration in the milk oligopeptide solution resulted in a blue shift on its maximal absorption wavelength. For example, NQFLPYPYYAK shifted its maximal absorption wavelength from 290 nm to 287 nm after (α)-tocopherol was introduced (). IAKYI and IPIQYV were absorbed maximally at 289 nm and the incorporation of (α)-tocopherol led to their maximal absorption wavelength shift to 287 nm. It was speculated that (α)-tocopherol might interact with milk oligopeptide to decrease the electronic excitation energy for the π-π* transition of chromophore rings in the aromatic amino acid residues. This could further alter the hydrogen bonds between aromatic amino acid residues (tyrosine) and solvent molecules, which caused the maximal absorption wavelength shift of the milk oligopeptides.[Citation38,Citation40]
Figure 4. Effect of (α)-tocopherol on alteration of maximal absorption wavelength of milk oligopeptides in UV-Vis spectra. a→ e represents the concentration of (α)-tocopherol. The concentrations are: a = 0.0 mmol/L, b = 0.05 mmol/L, c = 0.10 mmol/L, d = 0.20 mmol/L, and e = 0.30 mmol/L
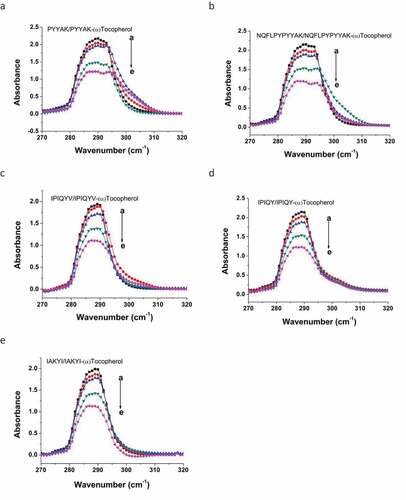
Fluorescence Spectroscopy: Fluorescence spectroscopy is always used to reflect the interactions between proteins/peptides and other molecules. Generally, tryptophan and tyrosine are the essential amino acid residues in proteins/peptides that possess fluorescent property.[Citation36] In the present study, the milk oligopeptides only contained tyrosine residue. These milk oligopeptides exhibited the maximal emission wavelength of 304–306 nm under a 280 nm excitation wavelength (). (α)-Tocopherol did not significantly alter the maximal emission wavelength of these milk oligopeptides. However, the endogenous fluorescent intensity of the milk oligopeptides was reduced with the incorporation of (α)-tocopherol and the reduction of the fluorescent intensity in these oligopeptides became stronger with the increase of the (α)-tocopherol concentration in the milk oligopeptide solution. It has been confirmed that the fluorescent quenching consisted of static and dynamic quenching.[Citation36,Citation41] In the dynamic quenching process, the collision between fluorescent substance and quencher results in the transfer of energy and/or electrons. The static quenching results from the formation of complex between fluorescent substance and quencher.[Citation41,Citation42] The Stern-Volmer formula was used in the present study to elucidate the fluorescent quenching mechanism of the milk oligopeptide with the presence of (α)-tocopherol (). A linear curve was generated between the ratio of each milk oligopeptide fluorescent quenching over the concentration of (α)-tocopherol and the Stern-Volmer constant (Ksv) and quenching rate constant (Kq) are listed in . represents the average life span of fluorescent molecule without addition of (α)-tocopherol and is general 10−8s.[Citation43] IPIQY interacted with (α)-tocopherol obtained the significant high Ksv and Kq values compared to other milk oligopeptides (p < .05). Also, Ksv and Kq values of PYYAK and IAKYI were higher than those of NQFLPYPYYAK and IPIQYV interacted to (α)-tocopherol (p < .05). Based on , IPIQY and PYYAK with (α)-tocopherol exhibited the antioxidative abilities on linoleic acid oxidation superior to other milk oligopeptides and therefore these results might be correlated to the MDA generation in linoleic acid oxidation system. Essentially, higher values of the Stern-Volmer constant (Ksv) were found in all milk oligopeptides interacted with (α)-tocopherol. Meanwhile, the quenching rate constant (Kq) of these samples were much higher than the quenching rate constant caused by the greatest collisions among biological molecules (2.0 × 1010 Lmol−1s−1). These illustrated that the quenching mechanism between the milk oligopeptides and (α)-tocopherol essentially resulted from the static process. It was speculated that the interaction between the milk oligopeptide and (α)-tocopherol could result in the formation of the milk oligopeptide-(α)-tocopherol complex, which altered the structural conformation of the milk oligopeptide and thus decreased its endogenous fluorescent intensity.[Citation42]
Table 1. Fluorescent quenching constant (Ksv) and quenching rate constant (Kq) of milk oligopeptide-(α)-tocopherol complexes
Figure 5. Effect of (α)-tocopherol on reduction of fluorescent intensity of milk oligopeptides. a→ f represents the concentration of (α)-tocopherol. The concentrations are: a = 0.0 × 10−10 mol/L, b = 0.05 × 10−10 mol/L, c = 0.10 × 10−10 mol/L, d = 0.15 × 10−10 mol/L, e = 0,20 × 10−10 mol/L and f = 0.25 × 10−10 mol/L
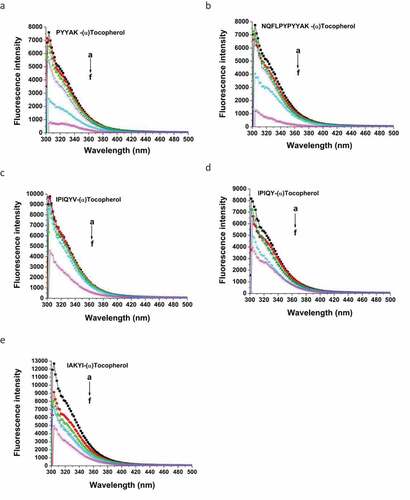
Figure 6. Stern-Volmer fitting curve using milk oligopeptides and (α)-tocopherol. Stern-Volmer formula:
, where F0 and F represent fluorescent intensity of milk oligopeptide before and after the addition of (α)-tocopherol. Kq and Ksv are quenching rate constant and quenching, respectively.
represents the average life span of fluorescent molecule without addition of (α)-tocopherol
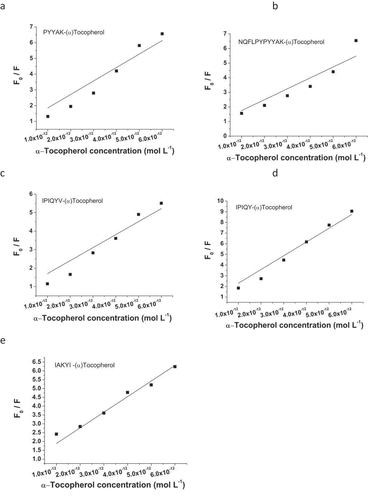
Figure 7. Fourier transform infrared spectrum of milk oligopeptides and milk oligopeptide-(α)-tocopherol complexes
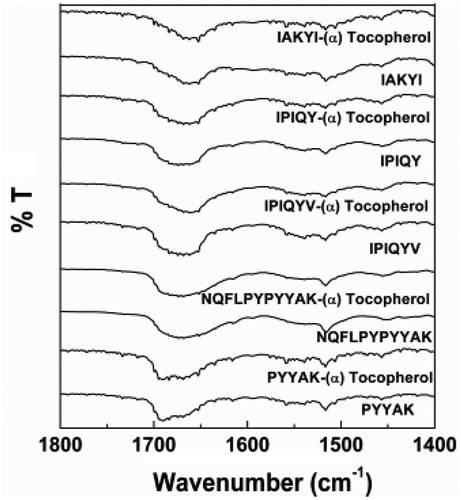
Fourier Transform Infrared Spectroscopy (FTIR): FTIR is a common approach to study the conformational changes of secondary structure of proteins and peptides through unveiling the driving forces of inter- and intra-molecular interactions.[Citation43] In the present study, the FTIR spectra of these milk oligopeptides exhibited typical amide I and II at 1600–1700 cm−1 and 1500–1600 cm−1, respectively. The amide I and amide II were two dominant vibrational bands of peptide backbone. The absorption of the amide I in these oligopeptides was mainly attributed to the stretching vibration of carbonyl C = O in amino acid residues (approximately 80%), whereas the C-N stretching vibration and the C-N-H plane bending resulted in the absorption of the amide II.[Citation44] The amide I band has been confirmed to be more sensitive than the amide II in terms of the alteration of the protein secondary structure.[Citation45] The vibration frequencies of the amide I band (mainly C = O stretching frequency) were revealed to be closely related to each secondary structure of protein/peptide. The amide I band was high sensitivity to slight alteration of molecular geometry and hydrogen bonding, which determined its unique importance to analyze protein/peptide spatial conformation changes. The second derivative and curve-fitting analysis were established to qualify and quantify the composition of various secondary structures of α-helix, β-sheet, β-turn, and random coil.[Citation46] In our study, it was observed that the incorporation of (α)-tocopherol shifted the peak of the oligopeptide amide I toward lower wavenumber by 1–4 cm−1, and the peak shape of the amide I was altered (). These demonstrated that the milk oligopeptides interacted with (α)-tocopherol, altering the amide I structural conformation of the milk oligopeptides.[Citation45] It has been known that the secondary structures mainly consisted of α-helix, β-sheet, β-turn, and random coil, and these secondary structures were all assembled on the amide I band in proteins and peptides.[Citation36] Therefore, we further investigated the percentage of each secondary structure in the amide I of these milk oligopeptides using second derivative analysis and curve-fitting analysis (). It was reported that the peak at 1646–1664 cm−1, 1610–1640 cm−1 and 1682–1700 cm−1, 1664–1681 cm−1, and 1637–1645 cm−1 represented the structure of α-helix, β-sheet, β-turn and random coil in the amide I, respectively.[Citation30,Citation43,Citation46,Citation47]
Figure 8. Second derivative and curve-fitting analysis of secondary structure of milk oligopeptide and milk oligopeptide-(α)-tocopherol complex using PeakFit 4.12 software
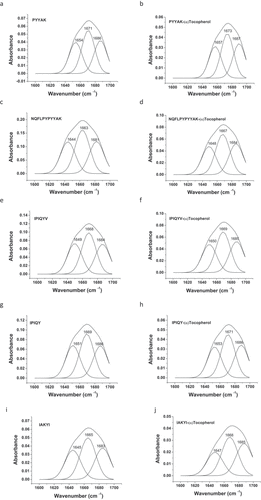
The FTIR spectra in revealed the spatial conformation alteration of milk oligopeptides before and after the interaction to (α)-tocopherol . The calculated percentages of each secondary structure in the milk oligopeptides with and without the addition of (α)-tocopherol were listed in and were attempted to characterize the interaction behavior between milk oligopeptides and (α)-tocopherol. It was observed that (α)-tocopherol altered the secondary structure of these milk oligopeptides. For instance, IAKYI did not show any α-helix structure without (α)-tocopherol. However, the interaction of IAKYI with (α)-tocopherol led to the IAKYI-(α)-tocopherol complex with a 26.2% of the α-helix structure. The conversion from α-helix and random coil to β-sheet and β-turn occurred to NQFLPYPYYAK after the interaction with (α)-tocopherol. It should be noted that the milk oligopeptide-(α)-tocopherol complex increased the percentage of the β-turn structure, indicating that the interaction between milk oligopeptide and (α)-tocopherol impacted the hydrogen bonds of the milk oligopeptides and thus altered the structure of milk oligopeptides.[Citation36] It has been reported that β-turn played a vital role in the antioxidant capacity of peptides and the ratio of β-turn to β-sheet could reflect the antioxidant feature of peptides.[Citation48] In the present study, it was found that IPIQY, IPIQYV, and PYYAK significantly inhibited the oxidation of linoleic acid (). Additionally, IPIQY and PYYAK with the presence of (α)-tocopherol dramatically reduced the formation rate of malondialdehyde (). However, IPIQYV with (α)-tocopherol synergistically inhibited the peroxide value during the linoleic acid oxidation (). IPIQY, IPIQYV, and PYYAK, compared to the other oligopeptides, possessed higher percentage of β-turn and β-turn/β-sheet value (). These indicated that the interactions between these oligopeptides and (α)-tocopherol, such as hydrogen bonds and van der waals force, could induce an alteration on the β-turn structure to enhance the antioxidant activity, which could further enhance the inhibitory effect on the linoleic acid oxidation.
Table 2. Secondary structure percentage in milk oligopeptides and milk oligopeptide-(α)-tocopherol complexes
Conclusion
In conclusion, milk oligopeptides were introduced to Fe2+-vitamin C-induced linoleic acid oxidation model fortified with (α)-tocopherol. Milk oligopeptides, PYYAK and IPIQY, appeared to significantly enhance the inhibition of the linoleic acid oxidation with (α)-tocopherol through inhibiting the formation of malondialdehyde. IPIQYV coupled with (α)-tocopherol reduced the peroxide value of the linoleic acid oxidation model. The increase of the (α)-tocopherol concentration altered the maximal absorption wavelength of these milk oligopeptides. Fluorescence spectroscopy analysis illustrated that the endogenous fluorescent intensity reduction of milk oligopeptides resulted from the static quenching process through the inter-molecular interactions between milk oligopeptides and (α)-tocopherol. Fourier Transform Infrared Spectroscopy analysis indicated that the β-turn secondary structure increased in milk oligopeptides with the addition of (α)-tocopherol.
Acknowledgments
This study was financially supported by National Natural Science Foundation of China (No.31741102), Major Science and Technology Projects and Major Agricultural Project of Zhejiang Province (No.2017C02033), National Natural Science Foundation of China (No.31871763), Key Research and Development Projects of Zhejiang Province (No. 2018C02053), and Natural Sciences Fund of Zhejiang Province (No. LY14C200007).
Additional information
Funding
References
- Elias, P. M.; Brown, B. E.; Ziboh, V. A. The Permeability Barrier in Essential Fatty Acid Deficiency: Evidence for a Direct Role for Linoleic Acid in Barrier Function. J. Invest. Dermatol. 1980, 74, 230–233. DOI: 10.1111/1523-1747.ep12541775.
- Sanders, T. A. B.;. Polyunsaturated Fatty Acids in the Food Chain in Europe. Am. J. Clin. Nutr. 2000, 71, 176–178. DOI: 10.1093/ajcn/71.1.176s.
- Li, G. P.; Singh, A.; Liu, Y. D.; Sunderland, B.; Li, D. Comparative Effects of Sandalwood Seed Oil on Fatty Acid Profiles and Inflammatory Factors in Rats. Lipids. 2013, 48, 105–113. DOI: 10.1007/s11745-012-3752-4.
- Mateos, R.; Trujillo, M.; Carmen Pérez-Camino, M.; Moreda, W.; Cert, A. Relationships between Oxidative Stability, Triacylglycerol Composition, and Antioxidant Content in Olive Oil Matrices. J. Agric. Food Chem. 2005, 53, 5766–5771. DOI: 10.1021/jf0504263.
- Girardi, F.; Cichelli, A.; Perri, E.; Basti, C.; d’Alessandro, N. Oxidative Treatments of Solid Olive Residues : Effects on Phenolic and Fatty Acid Fractions. Eur. J. Lipid Sci. Technol. 2014, 116, 352–359. DOI: 10.1002/ejlt.201300083.
- Rubilar, M.; Morales, E.; Sáez, R.; Acevedo, F.; Palma, B.; Villarroel, M.; Shene, C. Polyphenolic Fractions Improve the Oxidative Stability of Microencapsulated Linseed Oil. Eur. J. Lipid Sci. Technol. 2012, 114, 760–771. DOI: 10.1002/ejlt.201100230.
- Cao, J.; Li, H. Y.; Xia, X.; Zou, X. G.; Li, J.; Zhu, X. M.; Deng, Z. Y. Effect of Fatty Acid and Tocopherol on Oxidative Stability of Vegetable Oils with Limited Air. Int. J. Food. Prop. 2015, 18, 808–820. DOI: 10.1080/10942912.2013.864674.
- Samaranayaka, A. G. P.; Li-chan, E. C. Y. Food-derived Peptidic Antioxidants: A Review of Their Production, Assessment, and Potential Applications. J. Funct. Foods. 2011, 3, 229–254. DOI: 10.1016/j.jff.2011.05.006.
- Tironi, V. A.; Añón, M. C. Antioxidant Activity of Amaranth Protein Hydrolysate against Thermal Oxidation of Vegetable Oils. J. Am. Oil Chem. Soc. 2014, 91, 1583–1594. DOI: 10.1007/s11746-014-2509-z.
- Power, O.; Jakeman, P.; FitzGerald, R. J. Antioxidative Peptides: Enzymatic Production, in Vitro and in Vivo Antioxidant Activity and Potential Applications of Milk-derived Antioxidative Peptides. Amino Acids. 2013, 44, 797–820. DOI: 10.1007/s00726-012-1393-9.
- Skall Nielsen, N.; Debnath, D.; Jacobsen, C. Oxidative Stability of Fish Oil Enriched Drinking Yoghurt. Int. Dairy J. 2007, 17, 1478–1485. DOI: 10.1016/j.idairyj.2007.04.011.
- Farvin, K. H. S.; Baron, C. P.; Nielsen, N. S.; Jacobsen, C. Antioxidant Activity of Yoghurt Peptides : Part 1- in Vitro Assays and Evaluation in ω-3 Enriched Milk. Food Chem. 2010, 123, 1081–1089. DOI: 10.1016/j.foodchem.2010.05.067.
- Rossini, K.; Noreña, C. P. Z.; Cladera-Olivera, F.; Brandelli, A. Casein Peptides with Inhibitory Activity on Lipid Oxidation in Beef Homogenates and Mechanically Deboned Poultry Meat. LWT - Food Sci. Technol. 2009, 42, 862–867. DOI: 10.1016/j.lwt.2008.11.002.
- Hogan, S.; Zhang, L.; Li, J. R.; Wang, H. J.; Zhou, K. Q. Development of Antioxidant Rich Peptides from Milk Protein by Microbial Proteases and Analysis of Their Effects on Lipid Peroxidation in Cooked Beef. Food Chem. 2009, 117, 438–443. DOI: 10.1016/j.foodchem.2009.04.040.
- Hernández-Ledesma, B.; Dávalos, A.; Bartolomé, B.; Amigo, L. Preparation of Antioxidant Enzymatic Hydrolysates from α -lactalbumin and β-Lactoglobulin- Identification of Active Peptides by HPLC-MS/MS. J. Agric. Food Chem. 2005, 53, 588–593. DOI: 10.1021/jf048626m.
- Hernández-Ledesma, B.; Miralles, B.; Amigo, L.; Ramos, M.; Recio, I. Identification of Antioxidant and ACE-inhibitory Peptides in Fermented Milk. J. Sci. Food Agric. 2005, 85, 1041–1048. DOI: 10.1002/jsfa.2063.
- Li, X. R.; Yan, Y. H. Comparative Study of the Interactions between Ovalbumin and Five Antioxidants by Spectroscopic Methods. J. Fluoresc. 2017, 27, 213–225. DOI: 10.1007/s10895-016-1948-3.
- Li, X. R.; Chen, D. J.; Wang, G. K.; Lu, Y. Study of Interaction between Human Serum Albumin and Three Antioxidants : Ascorbic Acid, a -tocopherol, and Proanthocyanidins. Eur. J. Med. Chem. 2013, 70, 22–36. DOI: 10.1016/j.ejmech.2013.09.033.
- Young Park, E.; Nakamura, Y.; Sato, K.; Matsumura, Y. Effects of Amino Acids and Peptide on Lipid Oxidation in Emulsion Systems. J. Am. Chem. Soc. 2012, 89, 477–484.
- Kim, S. K.; Kim, Y. T.; Byun, H. G.; Nam, K. S.; Joo, D. S.; Isolation, S. F. Characterization of Antioxidative Peptides from Gelatin Hydrolysate of Alaska Pollack Skin. J. Agric. Food Chem. 2001, 49, 1984–1989. DOI: 10.1021/jf000494j.
- Huang, W. Y.; Majumder, K.; Wu, J. P. Oxygen Radical Absorbance Capacity of Peptides from Egg White Protein Ovotransferrin and Their Interaction with Phytochemicals. Food Chem. 2010, 123, 635–641. DOI: 10.1016/j.foodchem.2010.04.083.
- Saito, K.; Jin, D. H.; Ogawa, T.; Muramoto, K.; Hatakeyama, E.; Yasuhara, T.; Nokihara, K. Antioxidative Properties of Tripeptide Libraries Prepared by the Combinatorial Chemistry. J. Agric. Food. Chem. 2003, 51, 3668–3674. DOI: 10.1021/jf021191n.
- Yuan, H. N.; Lv, J. M.; Gong, J. Y.; Xiao, H. L.; Zhao, G. S.; Xiao, G. N.; Xu, H.; Wang, W. C. Microbial Transglutaminase Enhances Antioxidant Activity of Yogurt through Altering Pattern of Water-soluble Peptides and Increasing Release of Amino Acids. Int. J. Food Sci. Technol. 2018, 53, 1030–1044. DOI: 10.1111/ijfs.13679.
- Kunimoto, M.; Inoue, K.; Nojima, S. Effect of Ferrous Ion and Ascorbate-induced Lipid Peroxidation on Liposomal Membranes. Biochim. Biophys. Acta. Biomembr. 1981, 646, 169–178. DOI: 10.1016/0005-2736(81)90284-4.
- Yamamoto, K.; Takahashi, M.; Niki, E. Role of Iron and Ascorbic Acid in the Oxidation of Methyl Linoleate Micelles. Chem. Lett. 1987, 16, 1149–1152. DOI: 10.1246/cl.1987.1149.
- Leonard, T. R.; Gregory, W. T.; Michael, L. C.; Gerald, C. C.; Raphael, B. O.; David, B. O. Lipid Peroxidation and the Thiobarbituric Acid Assay: Standardization of the Assay When Using Saturated and Unsaturated Fatty Acids. Korean. Soc. Biochem. Mol. Biol. 2004, 37, 749–752.
- Hornero-Méndez, D.; Pérez-Gálvez, A.; Mínguez-Mosquera, I. A Rapid Spectrophotometric Method for the Determination of Peroxide Value in Food Lipids with High Carotenoid Content. J. Am. Oil Chem. Soc. 2001, 78, 1151–1155. DOI: 10.1007/s11746-001-0404-y.
- Laguerre, M.; López-Giraldo, L. J.; Lecomte, J.; Baréa, B.; Cambon, E.; Tchobo, P. F.; Baroun, N.; Villeneuve, P. Conjugated Autoxidizable Triene (CAT) Assay: A Novel Spectrophotometric Method for Determination of Antioxidant Capacity Using Triacylglycerol as Ultraviolet Probe. Anal. Biochem. 2008, 380, 282–290. DOI: 10.1016/j.ab.2008.06.006.
- Tyson, C. A.; Frazier, J. M. In Vitro Toxicity Indicators (section VII Lipid Peroxidation); Academic Press, Inc: San Diego, CA, 1994.
- Yuan, H. N.; Lv, J. M.; Gong, J. Y.; Xiao, G. N.; Zhu, R. Y.; Li, L.; Qiu, J. N. Secondary Structures and Their Effects on Antioxidant Capacity of Antioxidant Peptides in Yogurt. Int. J. Food Prop. 2018, 21, 2167–2180. DOI: 10.1080/10942912.2018.1501700.
- Rossi, M.; Alamprese, C.; Ratti, S. Tocopherols and Tocotrienols as Free Radical-scavengers in Refined Vegetable Oils and Their Stability during Deep-fat Frying. Food Chem. 2007, 102, 812–817. DOI: 10.1016/j.foodchem.2006.06.016.
- Upston, J. M.; Terentis, A. C.; Stocker, R. Tocopherol-mediated Peroxidation of Lipoproteins: Implications for Vitamin E as a Potential Antiatherogenic Supplement. Faseb. J. 1999, 13, 977–994. DOI: 10.1096/fasebj.13.9.977.
- Pratt, D. A.; Tallmant, K. A.; Porter, N. A. Free Radical Oxidation of Polyunsaturated Lipids: New Mechanistic Insights and the Development of Peroxyl Radical Clocks. Acc. Chem. Res. 2011, 44, 458–467. DOI: 10.1021/ar200024c.
- Barclay, L. R. C.; Ingold, K. U. Autoxidation of A Model Membrane. A Comparision of the Autoxidation of Egg Lecithin Phosphatidylcholine in Water and in Chlorobenzene. J. Am. Chem. Soc. 1980, 102, 7792–7794. DOI: 10.1021/ja00546a033.
- Li, Y. W.; Li, B.; He, J. G.; Qian, P. Structure–Activity Relationship Study of Antioxidative Peptides by QSAR Modeling: The Amino Acid Next to C-terminus Affects the Activity. J. Pept. Sci. 2011, 17, 454–462. DOI: 10.1002/psc.1345.
- Darwish, S. M.; Abu Sharkh, S. E.; Abu Teir, M. M.; Makharza, S. A.; Abu-hadid, M. M. Spectroscopic Investigations of Pentobarbital Interaction with Human Serum Albumin. J. Mol. Struct. 2009, 963, 122–129. DOI: 10.1016/j.molstruc.2009.10.023.
- Ni, Y. N.; Lin, D. Q.; Kokot, S. Synchronous Fluorescence, UV–Visible Spectrophotometric, and Voltammetric Studies of the Competitive Interaction of Bis (1, 10-phenanthroline) Copper (II) Complex and Neutral Red with DNA. Anal. Biochem. 2006, 352, :231–242. DOI: 10.1016/j.ab.2006.02.031.
- Han, X. L.; Tian, F. F.; Ge, Y. S.; Jiang, F. L.; Lai, L.; Li, W. D.; Yu, Q. L. Y.; Wang, J.; Lin, C.; Liu, Y. Spectroscopic, Structural and Thermodynamic Properties of Chlorpyrifos Bound to Serum Albumin: A Comparative Study between BSA and HSA. J. Photochem. Photobiol. B. 2012, 109, 1–11. DOI: 10.1016/j.jphotobiol.2011.12.010.
- Poureshghi, F.; Ghandforoushan, P.; Safarnejad, A.; Soltani, S. Interaction of an Antiepileptic Drug, Lamotrigine with Human Serum Albumin (HSA): Application of Spectroscopic Techniques and Molecular Modeling Methods. J. Photochem. Photobiol. B. 2017, 166, 187–192. DOI: 10.1016/j.jphotobiol.2016.09.046.
- Agarwal, S.; Jangir, D. K.; Mehrotra, R. Spectroscopic Studies of the Effects of Anticancer Drug Mitoxantrone Interaction with Calf-thymus DNA. J. Photochem. Photobiol. B. 2013, 120, 177–182. DOI: 10.1016/j.jphotobiol.2012.11.001.
- Wang, Y. Q.; Zhang, H. M.; Zhang, G. C.; Tao, W. H.; Tang, S. H. Interaction of the Flavonoid Hesperidin with Bovine Serum Albumin: A Fluorescence Quenching Study. J. Lumin. 2007, 126, 211–218. DOI: 10.1016/j.jlumin.2006.06.013.
- II’ichew, Y. V.; Perry, J. L.; Simon, J. D. Interaction of Ochratoxin A with Human Serum Albumin. A Common Binding Site of Ochratoxin A and Warfarin in Subdomain IIA. J. Phys. Chem. 2002, 106, 460–465. DOI: 10.1021/jp012315m.
- Darwish, S. M.; Ghithan, J.; Abuteir, M. M.; Faroun, M.; Abu-hadid, M. M. Spectroscopic Investigations of Pentobarbital Interaction with Transthyretin. J. Spectrosc. 2012, 2013, 1–10. DOI: 10.1155/2013/927962.
- Zandomeneghi, G.; Krebs, M. R. H.; McCammon, M. G.; Fändrich, M. FTIR Reveals Structural Differences between Native β-sheet Proteins and Amyloid Fibrils. Protein Sci. 2004, 13, 3314–3321. DOI: 10.1110/ps.041024904.
- Zhang, G. W.; Zhao, N.; Hu, X.; Tian, J. Interaction of Alpinetin with Bovine Serum Albumin : Probing of the Mechanism and Binding Site by Spectroscopic methods.Spectrochimica. Acta. A. Mol. Biomol. Spectrosc. 2010, 76, 410–417. DOI: 10.1016/j.saa.2010.04.009.
- Kong, J. L.; Yu, S. N. Fourier Transform Infrared Spectroscopic Analysis of Protein Secondary Structures. Acta Biochim. Biophys. Sin. 2007, 39, 549–559. DOI: 10.1111/j.1745-7270.2007.00320.x.
- Darwish, S. M.; Aiaidah, S. Y.; Khalid, I. M.; Abuteir, M. M.; Qawasmi, L. Spectroscopic Investigations of β -amyloid Interactions with Propofol and L-Arginine. J. Biophys. 2015, 5, 50–67.
- Cian, R. E.; Vioque, J.; Drago, S. R. Structure–Mechanism Relationship of Antioxidant and ACE I Inhibitory Peptides from Wheat Gluten Hydrolysate Fractionated by pH. Food Res. Int. 2015, 69, 216–223. DOI: 10.1016/j.foodres.2014.12.036.