ABSTRACT
The physicochemical and functional properties of flour (MF) and a protein isolate (MPI) obtained from moring leaves were evaluated and compared. These properties were evaluated and compared with a commercial soybean protein isolate (SPI). MPI was obtained by alkaline solubilization at pH 11.5 and isoelectric precipitation at pH 4.5. The protein content of MPI was 75.8%, with a yield of 6.5% when obtained by lyophilization. Electrophoresis analysis confirmed the presence of proteins between 8 and 120 kDa, and FTIR analysis confirmed the yield of MPI and the decrease of non-protein constituents, such as carbohydrates. The MF showed good gelation properties [5 mL (100%) at 14% (w/v) flour]. MPI showed even better gelling properties (10% to 78%) than SPI and similar water and oil absorption capacities (WAC and OAC) (3 g g-1 and 1.5 g g-1 respectively) as SPI. The pH had a significant influence on these properties. It can be concluded that MPI is an important protein source and may be a viable alternative as a functional food ingredient comparable to SPI in some properties such as WAC, OAC, and emulsion stability at low pH.
Introduction
The food industry is looking for new protein ingredients due to the growing interest of consumers toward health, ethical food handling and/or religious preferences.[Citation1] The use of vegetable proteins (PP) is considered as a promising alternative, given their high nutrient content and good functional properties (FP), such as solubility, emulsifying capacity, foaming capacity, gelling capacity, oil absorption capacity, and water absorption capacity, among others.[Citation2] Soy protein (SP) has been one of the most widely used and marketed in the food industry, since it offers advantages in its price and its nutritional, functional, sensory, and medicinal properties, which have achieved consumer preference.[Citation3]
Vegetable protein isolates are mainly extracted from seeds, as they have a high protein content and high solubility. However, leaves have recently been recognized as abundant and low-cost sources of protein due to their ability to synthesize amino acids from unlimited and readily available sources such as sunlight, carbon dioxide, and atmospheric nitrogen.[Citation4] Leaves of various crops have been considered suitable for protein extraction because of their high protein content, regional availability, ability to meet societal needs, and current uses.[Citation5] In this regard, Aletor et al.,[Citation4] evaluated protein concentrates from the leaves of Vernonia amygdalina, Solanum africana, Amaranthus hybridus and Telfaria occidentalis. The solubility results indicated that protein extracts from the leaves of these vegetables could find good use in both acidic and alkaline foods and could be used to improve the nutritional value of low-protein foods.
For their part, Hojilla-Evangelista et al.[Citation6] evaluated an alfalfa leaf protein concentrate and found desirable properties of emulsifying ability and heat stability. Martin et al[Citation7] also noted that Ribulose 1,5-bisphosphate carboxylase/oxygenase (RuBisCO), obtained from sugar beet leaves, exhibited functional properties comparable or superior to those of whey and soy protein isolates. Famuwagun et al[Citation8] reported that protein isolates from the leaves of Amaranthus viridis, Solanum macrocarpon, and Telfaria occidentalis had higher solubility at basic pH, as well as higher structural flexibility and charge density, in addition to higher emulsifying and foaming capacity, revealing their potential to act as functional ingredients in food systems.
Materials and methods
Materials
Moringa leaves were collected from a plantation located in Soledad de Doblado, Veracruz, Mexico. The leaves were dehydrated at 40°C for 5 h in a commercial dehydrator (Excalibur, 3500 B, Saesa Trading, Luckey, Ohio, USA) and grounded in a food processor (Maxximum, MMV Series, USA). Moringa flour (MF) was vacuum packed. Soy protein isolate (PRONAT ProWinnerR), which contained 95% protein, was purchased from a natural products store (Puebla, Mexico).
Characterization of the moringa leaves flour
Crude protein, ethereal extract, crude fiber, ash content and moisture content of MF were determined following AOAC official methods of analysis[Citation9] 978.04, 930.09, 930.10, 930.05 and 930.04, respectively.
Extraction of moringa protein isolates
First, the MF was dispersed in distilled water (1:10, w/v) and then alkaline extraction was performed at pH between 11.5 and 12.0 using 1 M NaOH with stirring for 2 h. Subsequently, the solution was centrifuged at 12,768 g at 4°C for 15 min in a centrifuge (Thermo Scientific, SC210A, Hunan Labwe Scientific Instruments, Changsha, Hunan, China). The supernatant was collected by decanting and filtered with a cotton cloth. Isoelectric precipitation of protein in the supernatant was carried out by adjusting the pH between 1 and 5 with 2.5 N HCl; allowing the supernatant to stand for 2 h. The sample was centrifuged and centrifuged in a centrifuge. The sample was centrifuged at 12.768 g for 15 min and the supernatant was discarded. The isolated proteins were washed with distilled water until neutralized, then freeze-dried (FreeZone™ Freeze-Dry Systems, 7750020, Labconco Corporation, Kansas City, Missouri, California) for 48 h and stored in tubes at 27°C for further analysis. Alkaline extraction was performed following the methodology described by Gonzalez-Garza et al.[Citation10] Only the most representative extractions that presented significant differences are discussed in this study and were designated as MPI, MPI1, MPI2 and MPI3.
The isolate with the highest yield and protein content was characterized and compared with both MF and an SPI. The crude protein content of the MPI was determined by the Kjeldahl method.[Citation9] The yield was calculated according to the method reported by Kaushik et al.[Citation11] with the following equation: yield (%) = (P/S)*100, where P is the weight (g) of the protein isolate after lyophilization and S is the weight (g) of the flour used for protein extraction.
Physicochemical properties of the MF, MPI, and SPI
Sodium dodecyl sulfate-polyacrylamide gel electrophoresis (SDS-PAGE)
The molecular weight of proteins from MF, MPI, and SPI samples was determined by 12% SDS-PAGE according to the Laemmli.[Citation12] Proteins were visualized by Coomassie blue staining. Gels were photo-documented on a ChemiDoc MP imaging system with Image Lab v. 4.0 software (Bio-Rad, USA).
Color
The color of the MF, MPI and SPI was determined with a colorimeter (MiniScan, EZ 4500 L, Hunter Associates Laboratory, Inc., Reston, VA, USA) following the method described by Mir et al.[Citation13] and using the CIELAB scale; the results were expressed using the coordinates L*, a*, and b*.
Structural characterization by Fourier-transform infrared spectroscopy (FTIR)
The structural characterization of the MF, MPI and SPI was performed by FTIR with the attenuated total reflection (ATR) sampling method using a spectrophotometer (Thermo Scientific©, iS50, Bruker, Vertex, Wisconsin, USA). The technique used 64 scans with a 4 cm−1 resolution in the 4000–400 cm−1 spectral region. The spectra were analyzed with the Origin 6.1 software (OriginLab Corporation, USA).
Functional properties of the MF, MPI, and SPI
Solubility
The solubility of MF, MPI, and SPI was determined using the method proposed by Achouri et al.[Citation14] The samples were dissolved in distilled water (1:100, w/v) at different pH values (4.5, 5.0, 5.5, 6.0. 7.0, and 7.5); pH was adjusted with HCl or NaOH. The soluble protein content in the supernatant was determined with the Bradford[Citation15] method, and the amount of protein in the sample was determined using the Kjeldahl method.[Citation9]
Solubility was calculated with the following equation: Solubility (%) = (protein amount in the supernatant/ protein amount in the initial sample) *100.
Emulsifying capacity (EC) and emulsion stability (ES)
The EC and ES were determined using the method described by Lawal et al.[Citation16] For the EC, samples were homogenized in distilled water (1:100, w/v) at different pH values (4.5, 5.0, 5.5, 6.0. 7.0, and 7.5). A volume of 30 mL of canola oil was added to 20 mL of each solution. The emulsion was obtained by homogenizing with a mixer (Taurus, New Mixo, China) at medium speed for 2 min and centrifuged at 525 g for 2 min.
The EC was calculated with the equation: EC (%) = (Vf/Vi) *100; where Vf is the volume of the emulsifying layer and Vi is the volume of the total contents of the tube. The ES was determined by heating the emulsions at 80°C for 30 min and then centrifuging them at 525 g for 2 min. The ES was calculated with the equation: ES (%) = (Final volume of the emulsifying layer/Initial volume of the emulsifying layer) * 100.
Foaming capacity (FC) and foam stability (FS)
The FC and FS were determined following the method proposed by[Citation17,Citation18] MF, MPI, and SPI samples were homogenized in distilled water (1:100, w/v) at different pH values (4.5, 5.0, 5.5, 6.0. 7.0, and 7.5). Thirty mL of each solution, 30 mL was mixed with a mixer at a medium speed for 1 min. The FC was calculated with the following equation: FC (%) = ((V2-V1)/V1) *100; where V2 is the volume of the protein solution after mixing, and V1 is the volume of the solution before mixing. The FS was calculated by measuring the foam volume after 30 min with the following equation: FS (%) = (Foam final volume/ foam total volume) *100.
Gelation
The gelation capacity was determined following the method described by Coffman and García.[Citation17] Samples of MF, MPI, and SPI samples were homogenized in distilled water (14:100, w/v) at different pH values (4.5, 5.0, 5.5, 6.0. 7.0, and 7.5). Five mL of each solution were transferred to different tubes, heated in a water bath at 92°C for one hour and cooled to 4°C for two hours. Gelation was recorded based on milliliters gelled.
Water absorption capacity (WAC) and oil absorption capacity (OAC)
WAC was determined following the method established by Timilsena et al.[Citation19] MF, MPI, and SPI samples (300 mg) were homogenized in 4.5 mL of distilled water. The solutions were vortexed (Eppendorf, Equipar, Mexico) for 10 min, mixed in a roller shaker for 1 h, and centrifuged at 756 g for 10 min. The supernatant was discarded and the tube was manually decanted to drain the remaining water in the protein sediment. The following equation was used to calculate the WAC: WAC = (a-b) /(b); where a is the sample mass and the absorbed water, and b is the sample mass. The OAC was determined in the same way as the WAC, but with canola oil.
Statistical analysis
The results were expressed as the mean of three independent determinations through an analysis of variance (ANOVA). In a first experiment to obtain the moringa protein isolates, data were analyzed using a completely randomized design (CRD) with one factor, four treatments, and three repeats to determine the best treatment (p ≤ .05) (). Results from the functional properties were analyzed using a completely randomized design (CRD) with pH as the simple factor, for three treatments (MF, MPI, and SPI), three repeats, and considering the pH factor with six levels (4.5, 5, 5.5, 6, 6.5, 7, and 7.5). In both experiments, the significant differences between the means of the treatments were determined. A Turkey was carried out with a significance level of p ≤ .05 using the SAS® software (version 9.3, SAS®, 2002–2010).
Table 1. Moringa leaves protein isolates obtained by alkaline solubilization and isoelectric precipitation. Protein content and yield
Results and discussion
Characterization of the moringa leaves flour
MF proximate analysis (carbohydrates, 47.77%; proteins, 26.72%; ash, 9.68%; crude fiber, 5.82%; moisture, 5.6%; ether extract, 4.4%), showed that proteins were the second most abundant component after carbohydrates. The protein content was similar to that reported for alfalfa leaves flour (26%)[Citation6] and higher than the reported for blackberry leaves flour (24.34%).[Citation20] González-Garza et al.[Citation10] reported protein content of 26.54% in an ether extract of defatted moringa seed flour, similar to what we observed in this study. The higher protein content found may be because of the climate conditions where the plant grows up.
Extraction of moringa protein isolates
The protein content and yield of the lyophilized moringa protein isolates obtained by alkaline solubilization and isoelectric precipitation at different pH is shown in . The pH conditions during the protein extraction process influence the amount of protein, yield, and color (p < .05). Protein percentages ranged from 40.43 to 75.77%, and yield from 2 to 6.5%. The MPI sample showed the highest protein percentage (75.77%) and yield (6.50%). The MPI protein content was higher than that of a moringa seed isolate (49.67%) extracted at pH 11.0 and 4.0,[Citation10] which is similar to the reported for protein isolates from chickpea obtained by the optimization process[Citation21] and lower than the isolates from white goosefoot leaves (Chenopodium album) (10.66 and 10.98%) extracted by alkaline solubilization at pH 11.0 and 12.0, respectively, with isoelectric precipitation at pH 4.5. The protein contents indicate the absence of a pre-treatment step to remove chlorophylls, soluble contaminants, phenolic remnants. The yield of the protein isolates could be due to the high hydrophobic character of leaf proteins, as was mentioned by Borgues et al.[Citation22] This high hydrophobic character could encourage protein aggregation that leads to reduced solubility in aqueous solutions. In addition, may be there little cell breakage, which makes protein extraction difficult.
The Codex Alimentarius[Citation23] indicates that soy isolates must comply with a protein content of 90% in dry weight and that the protein isolates from other plants must have a percentage higher than 40%. Thus, the MPI obtained in this study can be considered as a food supplement with a high protein value to be incorporated into other food matrices. Regarding color, the MPI1 and MPI3 samples showed an opaque yellow-green color. The MPI sample had a more luminous and yellow color, but less red when the isoelectric precipitation pH increased, unlike the MPI2 sample that, at lower pH values, showed a totally dark color as shown in Supplementary Table 1. The isoelectric precipitation pH largely determined the color; pH values lower than 3 presented black hues, and pH values greater than 3 resulted in brown-gray hues.[Citation24] It is important to consider the color of the protein isolate when determining the optimal pH range for extraction, as it will affect the final color of the food and therefore the consumer acceptance.
Physicochemical properties of the MF, MPI, and SPI
Sodium dodecyl sulfate-polyacrylamide gel electrophoresis (SDS-PAGE)
The SDS-PAGE analysis revealed the protein nature of the MF, MPI, and SPI, as shown in . The molecular weights of the MF proteins were 8, 12, 14, 23, 28, 38, 42, 48, 53, 66,70, 75, 90, 120, and 180 kDa. The most abundant protein had an approximate molecular weight of 53 kDa. These results are similar to those reported by Banik et al.,[Citation25] who reported proteins with molecular weights of 12, 14, 21, 28, 34, 51, 67, 100, and 146 kDa in the moringa leaves flour obtained from the Salt Lake region in Kolkata, India. Molecular weights of the proteins in the MPI were 8, 13, 23, 28, 40, 50, 55, 66, 70, 73, 75, and 120 kDa. As can be seen, 7 bands of the MF are retained in the MPI (approximately 50% of the bands in the MF), the other polypeptides in the MF, may not precipitate at pH 4.5. The other bands in the MPI, different from the MF, could be polypeptides resulting from hydrolyzed proteins from the MF. Paula et al.[Citation26] reported molecular weights greater than 29 kDa for a protein isolate obtained from moringa leaves. In this report, we found four of the seven bands they reported, (). The differences in both results could be related to the protocol used to obtain the protein isolate since the extracted proteins will depend on the buffers and reagents used. Previous studies have reported molecular weights of 6.5, 14.2, 29.0, and 40.0 kDa,[Citation27] and 6.0 and 50.0 kDa[Citation10] for a protein isolate obtained from moringa seeds by alkaline extraction at different pH (9,10, and 11) and isoelectric solubilization (4, 4.5, and 5).
Figure 1. SDS-PAGE at 12% protein profile of moringa flour (MF), a moringa protein isolate (MPI), and a soy protein isolate (SPI). Lane 1: Molecular marker, Lane 2: MF, Lane 3: MPI, Lane 4: SPI.
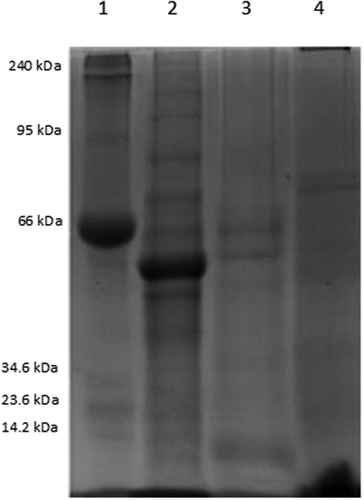
Tarone et al.[Citation28] mentioned that 7S globulins, trimeric glycoproteins, comprise molecular weights between 57, 58, and 42 kDa and are associated with hydrophobic interactions. Therefore, the proteins found in this study could correspond to the group of 7S globulins. The molecular weights of the polypeptides in the SPI were 1 − 12 kDa, 26, 28, 42, 52, 58, 66, 70, and 73 kDa; this coincides with the 7S globulins (β-conglycinin) composed by the α1, α, and β subunits, and the 11S glycinin composed of several acidic and basic polypeptides.[Citation29]
Color
The natural green color of moringa leaves results from the chlorophyll mixture directly linked to magnesium; during the drying process, chlorophyll changes into pyropheophytin and pheophytin. Thus, at higher temperatures, the green color decreases.[Citation30]
The MF had an opaque yellow-green color, probably due to the chlorophyll degradation during the dehydration process. Devisetti et al.[Citation31] reported L* = 49.1, a* = −1.19, and b* = 29.4 in moringa leaves. In this study, MF also showed a green color, but it was slightly less luminous and yellow (L* = 46.82, a* = −7.35, and b* = 32.55). This color difference could be explained by the different temperatures used during the dehydration process, as indicated by Ali et al.[Citation32]
For the MPI, the L* and b* values were lower and showed a darker color, (L* = 23.74, a* = 2.13, and b* = 14.03) appearing more red and less yellow (as shown in Supplementary Table 1), than the MF and SPI samples. The MF color is probably due to the presence of polyphenols, which oxidize and form structures such as o-quinones or o-dihydroxy that are linked by the thiol (-SH) or amino (-NH2) group.[Citation33] The luminosity of the SPI was higher than that of MF and the MPI; the SPI showed a beige to pale yellow color derived from the color of soybeans (L* = 89.44, a* = 0.65, and b* = 17.64). The lightness, redness, and yellowness of MPI is higher than that reported for the Moringa oleifera leaf protein complex,[Citation34] This difference could be the result of the process to obtain the protein complex, as they do not mention how they obtained the complex.
Structural characterization by Fourier-transform infrared spectroscopy (FTIR)
MF, MPI, and SPI FTIR spectra in the 1800–800 cm−1 region are shown in . The MF spectrum shows absorption bands associated with proteins in the 1650–1540 cm−1 region and carbohydrates in the 1800–1100 cm−1region. The MPI spectrum showed an increase in the protein absorption band, mainly at 1,633 cm− 1 and 1,550 cm− 1, which correspond to the amide I and amide II, respectively, which are associated with the protein secondary structure. Regarding carbohydrates, the absorption band decreases compared to the MF spectrum, which indicates a high protein content and low carbohydrate content. The SPI and MPI spectra were very similar; both show absorption bands associated with proteins and lack absorption bands related to carbohydrates; indicating a similar group and structure. These results coincide with those reported in the literature, which mention that the protein isolate from soybeans must contain 90% protein (on a dry basis) and must not contain sugars or dietary fiber. The FTIR analysis shows that the alkaline solubilization (pH 11.5) and isoelectric precipitation (pH 4.5) method allowed us to exhibit the increase of the proteins on the MPI and the decrease in the content of non-protein constituents, such as carbohydrates.
Solubility
The water solubility of proteins is of great importance in food technology since it affects properties like emulsification, gelation, foaming, and others.[Citation10] The solubility of MF, MPI, and SPI was determined at pH 4.5 to 7.5. Results showed that MF solubility at pH 4.5 was 1.41%; this pH value corresponds to the isoelectric point of the protein fractions in MF. The maximum solubility of 49.40% was obtained at pH 7.5 (). The solubility of MF increased as the pH was more alkaline, which indicates that solubility is pH-dependent.[Citation35] At pI, proteins have no net charge due to the balance between positive and negative charges. Therefore, reducing the electrostatic repulsion promotes protein aggregation and precipitation through hydrophobic interactions. However, if the protein remains in an acidic or alkaline environment, the electrostatic repulsion and hydration of the charged residues promote solubilization. These values were similar to those reported for legumes.[Citation36] The behavior of the protein solubility of the MF, may indicate that the proteins in the moringa leaves are mainly acidic proteins, rich in acidic amino acids, as aspartic and glutamic acids. These findings agree with those reported by Cattan et al.[Citation37] who found that the higher content of amino acids corresponded precisely to these amino acids.
Figure 3. Mean values of the solubility of moringa leaves flour (MF), moringa protein isolate (MPI), and soy protein isolate (SPI) at different pH values. Error bars represent the standard error for triplicate experiments. Comparisons were made with a Tukey test. n = 3.
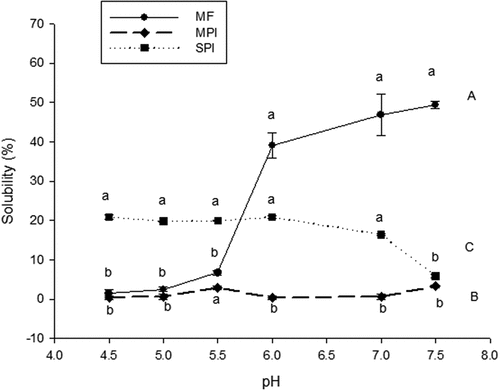
Regarding the solubility results, Cattan et al.[Citation37] found the same behavior, but higher solubility, the difference may be due to the drying process of the leaves, as they used lyophilization. The drying process we used could affect the conformation of the proteins, and for that reason, the solubility was less.
The solubility of the MPI showed increasing and decreasing trends as the pH variated from acidic to alkaline (), with a maximum peak at pH 5.5 and 7.5. As in MF, the minimum solubility was observed at its pI. The solubility of the MPI was lower than the reported for isolates extracted from leaves of sugar beet[Citation7] and alfalfa.[Citation6] The different solubility of MF and the MPI may be due to the protein nature of each of them and the extraction conditions as well. There was no significant difference between MPI and MF solubility at pH 4.5 (p > .05), while at other pH values, there was a significant difference (p < .05). The solubility of the MPI diminished drastically, in comparison with that of MF. Maybe the conditions to which the moringa flour was subjected were very drastic, which caused the conformation of the proteins to change drastically. The MPI solubility provides important information about its possible applications in food matrices.
The solubility of the SPI at different pH values was similar. There was no significant difference (p > .05) between pH 4.5 to 7.5. Martin et al.[Citation7] and Zheng et al.[Citation20] reported solubility values of 80% and 38% for soy isolates at pH 4.0 and 8.0, respectively. The SPI used in this work showed lower solubility, the process used to obtain the isolate may have an effect on the protein conformation and functionality. Protein solubility results from its tensoactive properties and can be affected by hydrogen bonds, electrostatic and hydrophobic interactions; the composition and distribution of the amino acids, molecular flexibility, form, and size.[Citation38]
Emulsifying capacity (EC) and emulsion stability (ES): Because of the importance of emulsions in food systems, it is necessary to understand their behavior in function of the medium pH. The EC and ES of the MF, MPI, and SPI were pH-dependent ()). There was an effect of the solubility in the EC of MF and MPI (). For MF, EC was lower at pH 4.5 to 5.5 than at the other pH values, there was no significant difference between pH 4.5 to 5.5 (p > .05), at pH 6 was the highest EC, 31.67% followed by 21.67% at pH 7, showing significant difference between these pH values (p < .05). The EC values found in this study to those reported by Aye and Adegun[Citation39] at pH 7.0 for moringa leaves flour. The EC of MF was low at pH 4.5, 5.0, and 5.5, probably because these pH values are within the pI range of the proteins, which results in low solubility, hydration, and absence of repulsive electrostatic forces that inhibit the aggregation of oil droplets. While the ES of MF was higher under alkaline conditions, 10% at pH 6.0 and 7.5 (); these pH values could have created an electrostatic repulsion strong enough to avoid the emulsion rupture by the aggregation of oil droplets.
Figure 4. Mean values of (a) emulsifying capacity, (b) emulsion stability, (c) foaming capacity, (d) foam stability, and (e) gelation for moringa leaves flour (MF), moringa protein isolate (MPI), and soy protein isolate (SPI) at pH 4.5, 5.0, 5.5, 6.0, 7.0, and 7.5. The error bars represent the standard error. Results of each treatment at different pH values were compared with a Tukey test; different superscripts indicate significant differences (p ≤ .05); this is indicated in the text. n = 3.
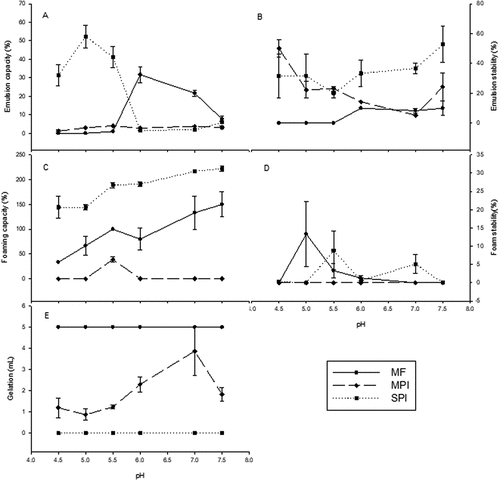
MF showed a more significant emulsifying activity than the MPI, perhaps the non-protein components in MF promote the emulsification. The MPI showed low EC due to the low solubility of its proteins (). Under these conditions, it is difficult for the proteins to rapidly move to the oil/water interface. The low electric charge intensity in the interface decreases the repulsion intensity between the oil droplets, increasing aggregates formation.[Citation40] ES of MPI was higher at pH 4.5 (50%) and significantly different (p ≤ .05) to the ES evaluated at pH 5.0, 5.5, 6.0, 7.0, and 7.5. The ES decreases as the pH reaches 7.0 (). The ES values of MPI were proportional to the solubility as it is in the MF, there was a direct effect of the solubility in the emulsion stability. However, meanwhile, the solubility of the MF was significantly higher (approximately 60 times higher), the emulsion stability was higher in the MPI. This may indicate that the high pH solubilization and isoelectric precipitation process, changed the conformation of the proteins in the MPI, having the ability to adopt a flexible structure that can interact with the oil phase by the hydrophobic amino acids exposed and with the water phase by the polar amino acids, so maintaining the stability. During the homogenization, the proteins are absorbed to the oil droplets’ surface, which lowers the interfacial tension and inhibits the coalescence of the droplets by forming membranes protective around the droplets[Citation41].
Interestingly, the MPI showed significantly higher emulsion stability than SPI at pH 4.5 (p < .05), and there was no significant difference (p > .05) between SPI and MPI at pH 5 and 5.5 () despite the great difference in solubility (), 40 times higher in SPI than in MPI. Perhaps because, at these low pH values, close to the isoelectric point, the net charge of the MPI proteins is near zero, reducing electrostatic repulsion and increasing hydrophobic interactions, which may promote the interaction with the oil phase that makes the stability of the emulsion.
Benelhadj et al.[Citation42] indicated a 60% EC at pH 7.0 and a 65% ES at pH 3.0 for a Spirulina isolate. Embaby et al.[Citation43] reported a 49.56% EC and a 78.95% ES for an Acacia isolate. The SPI showed the highest EC at pH 5.5 and 6.0, 52.3 and 41.33%, respectively (). This is because the pH modifies the surface charge, generating a balance between the dissociation of the carboxyl and amino groups of the protein molecules (hydrophilic-lipophilic balance), which influences the tensoactive properties. These EC values were significantly different (p ≤ .05) to those evaluated at pH 7.0 and 7.5. The ES increased with more alkaline pH values. The highest ES value for SPI was observed at pH 7.5 (). Therefore, the pH influences the ionization of the polar groups of tensoactive molecules; these molecules induce sufficient repulsive electrostatic forces to break the cohesion of the interface film,[Citation44] contributing to the emulsion stability. Feyzi et al.[Citation45] reported a 36% EC at pH 8.0 and an 88% ES at pH 6.0 for a soy protein isolate.
Foaming capacity (FC) and foam stability (FS)
FC and FS are important in the food industry; the characteristic texture of several foods depends on a foamed structure (bread, meringues, ice creams, mousses, milkshakes, beer, etc.). ) show the FC and FS profiles. There was significant difference (P < .05) in the FC of MF at the different pH values. The FC of MF increased as the pH value moved away from the isoelectric point of the proteins and solubility increased (). MF had a higher FS at pH 5.0; however, it did not show significant differences (p > .05) at the pH 5.5 value (). These values are greater than those found by Aye and Adegun (2013),[Citation39] who reported a FC of 10% and FS of 2% for moringa leaves flour. The drying conditions of the moringa leaves perhaps had an impact on the properties of the components in the flour.
MPI only showed FC (38.88%) at pH 5.5, which is the same pH where the MPI was soluble (). This indicates that the MPI can be used in food matrices at pH 5.5, where foaming capacity is guaranteed, such as some meat products, like sausages and hams. The low FC was probably caused by the limited availability of proteins to diffuse at the water-air interface and form foams. Due to its low solubility, the MPI did not show FS; certain solubility is required to guarantee protein absorption in the interface.
The FC of MPI was lower than the reported by Martin et al.,[Citation7] who reported, for a sugar beet leaf isolate, a 60% FC at pH 7.0 and a FS similar to a commercial soy isolate. Feyzi et al.[Citation45] reported a 136.67% FC at pH 8.0 and a higher FS, approximately 100%, at pH 3.0, 4.5, and 6.0 for a fenugreek seed isolate. The FC of the SPI increased as the medium was more alkaline (). This could be explained by the increase in solubility and the protein net charges, which weakened the hydrophobic interactions and increased protein flexibility, decreasing the surface tension and increasing the diffusion rate of protein to the water-air interface to encapsulate air bubbles.[Citation46] The highest FC of the SPI was 222.22% at pH 7.5; this value was higher than the 50% at pH 6.0 reported by Feyzi et al.[Citation45] for a commercial soy isolate. The SPI exhibited a higher FS at pH 5.5 (); under these conditions, the electrostatic repulsions reduced the coalescence of air bubbles. This value is lower than the 95% FS at pH 6.0 reported for a soy protein isolate.[Citation45] Both MF and MPI showed acceptable FC, which suggests their possible use as an aerating agent in food systems, for example, in whipped toppings and ice cream mixes.
Gelation
Gelation is an important property that affects food texture. The results for MF, MPI, and SPI are shown in . Due to its high solubility, MF had a better gel formation capacity than the MPI and SPI at the evaluated pH values. The gelation of MF at the different pH values was not significantly different (p > .05), which indicates that MF is able to exhibit gelation in products with a wide range of pH values. The higher gelation in the MF may be due to non-protein components of the flour, such as carbohydrates; Adebowale and Lawal[Citation47] reported that the content of carbohydrates had a positive effect in the gelation properties of a mucuna bean protein concentrate. A pH variation did not have any effect. This result is similar to Adebowale and Lawal,[Citation47] they tested at pH from 2 to 10, and in the range of 4 to 10 the gelation activity was the same at 14% of concentrate, as found in this work.
Proteins with firm gel formation capacity at low concentrations are considered desirable.[Citation48] Ogunsina et al.[Citation49] reported a gelation concentration of 14% in moringa seed flour. The gelation of the MPI was higher under alkaline conditions, which favor protein denaturation. Thermal gelation of proteins implies molecular unfolding on heating, then the aggregation of proteins emanating from the recently exposed groups to minimize the energy of the system, and formation of a continuous protein network giving a more elastic structure.[Citation50] The minimum gelation concentration value of the MPI was 7%; this value is lower than the 8% reported for Chinese quince,[Citation51] lower than 12% reported for Lathyrus sativus L.,[Citation47] lower than 23% reported for lupine protein isolated.[Citation52] Due to their high gelation, MF and MPI could be used in tofu and meat products for water, flavor, and ingredients retention.[Citation37]
Although the SPI is more soluble than the MPI, it did not show gelation. Martin et al.[Citation7] reported gelation at 100% concentration of soy protein isolate at pH 4.0 and 7.0. Zheng et al.[Citation20] reported gel formation at 14% concentration of soy protein isolate at pH 7.0. The differences may be due on the protocols used to obtain the isolates.
Water absorption capacity (WAC) and oil absorption capacity (OAC)
WAC is a basic functional property of the protein components that determine the quality (texture, appearance, flavor retention) and yield of finished meat products and other food preparations.[Citation39] MF had a higher WAC compared to the MPI and SPI (); this was probably caused by the more significant presence of lateral polar chains in the amino acids[Citation42] and the non-protein constituents of the MF. This result is higher than the one reported before,[Citation37] perhaps the drying conditions had an influence on this result. WAC of MF was higher than the reported by Devisetti et al.[Citation31] for moringa seed flour.
Figure 5. Mean values of (a) water absorption capacity (WAC) and (b) oil absorption capacity (OAC) of the moringa leaves flour (MF), moringa protein isolate (MPI), and soy protein isolate (SPI). Error bars represent the standard error for triplicate experiments. Results were compared with a t-Student test; different superscripts indicate significant differences (p ≤ .05). n = 3.
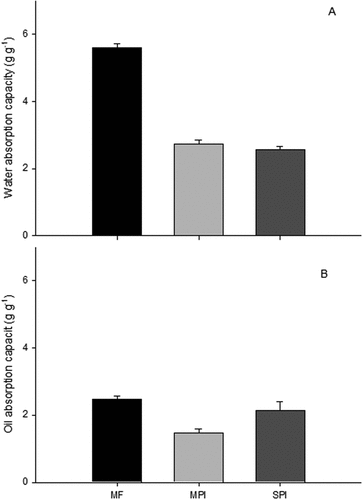
According to these results, MF could be used as a meat extensor due to its high protein content and functional properties, such as WAC, emulsifying capacity, and gelation, which improve flavor and texture.[Citation10] The WAC of the MPI was not significantly different (p > .05) compared to the SPI (). The extraction method probably affected the protein structure, which allowed the MPI to present WAC. This situation was similar to that reported by Al-Kahtani and Abou-Arab,[Citation53] who determined a WAC of 2.2 and 2.1 g g−1 for protein isolates from moringa and soy seeds, respectively. The SPI had a WAC of 2.52 g g−1, which is 63% of the value reported by Jain et al.[Citation27] for a commercial soy isolate. Additionally, they did not find WAC in a moringa seed isolate. The WAC of the MPI was superior to the reported for pea seed isolates[Citation54] and doubled the M. oleifera leaf protein complex WAC.[Citation34]
WAC variations for MF, MPI, and SPI are related to the nature and type of proteins, their amino acid composition, protein conformation, and the relationship between surface polarity and hydrophobicity, as well as the number and type of polar groups.[Citation1] The hydrophilic properties of the proteins are associated with the presence of polar groups, such as carbonyl, hydroxyl, amino, carboxyl, and sulfhydryl.[Citation53]
The MPI and SPI could be used as agglutinating agents in several foods, improving texture, mouthfeel, and viscosity; this is the case for baked products, such as bread, cakes, and muffins. Moreover, the isolates can be used in viscous foods since the WAC values range from 1.49–4.72 g g1.
The OAC is an important functional property that increases or improves flavor and taste retention of various food products.[Citation36] MF showed a higher OAC value than MPI (p < .05) and there was no significant difference between MF and SPI. There was no significant difference (p > .05) between the MPI and SPI (). The OAC value for MF was higher than the reported by Ogunsina et al.[Citation49] and Devisetti et al.,[Citation31] 1.29 and 1.77 g g−1, respectively. Xu et al.[Citation55] reported a lower OAC value for a chickpea seed isolate than the values obtained in this study. The OAC value for MPI was higher than 0.89 g g−1 reported for the Moringa oleifera leaf protein complex,[Citation34] this difference may be due to the process to obtain the protein complex, as they did not mention how they obtained it. The different OAC values for MF, MPI and SPI are influenced by parameters such as the size of the macromolecules, the surface area, electric charge, and hydrophobicity, which affect oil absorption.
Conclusion
The alkaline solubilization and isoelectric precipitation method allowed the extraction of protein isolates from moringa leaves. The pH conditions influenced the physicochemical properties of yield, protein content, color, and electrophoretic profile of the moringa protein isolates. The electrophoretic and FTIR analyses allowed the identification of the protein nature of the MPI. The MPI solubility was lower than that of MF, probably because of the type of proteins shown in the electrophoretic profiles and structural changes in the isolate proteins. MF showed good gelation properties. The MPI showed gelation, the WAC and OAC values were similar to those of the SPI. Due to its physicochemical and functional properties, the MPI could be used as a functional ingredient in the food industry, comparable in some properties like WAC, OAC, and emulsion stability at low pH, to the commercial soy isolate.
Supplemental Material
Download ()Acknowledgments
We thank the Consejo Nacional de Ciencia y Tecnología (CONACyT) for funding this research. (grant number 214O376). The authors gratefully acknowledge to Dr. Rigoberto Castro Rivera for his help with the figures.
Disclosure statement
No potential conflict of interest was reported by the author(s).
Supplementary material
Supplemental data for this article can be accessed on the publisher’s website
Additional information
Funding
References
- Lam, A. C. Y.; Can, K. A.; Tyler, R. T.; Nickerson, M. T. Pea Protein Isolates: Structure, Extraction, and Functionality. Food Rev. Int. 2018, 34(2), 126–147. DOI: 10.1080/87559129.2016.1242135.
- Makeri, M. U.; Mohamed, S. A.; Karim, R.; Ramakrishnan, Y.; Muhammad, K. Fractionation, Physicochemical, and Structural Characterization of Winged Bean Seed Protein Fractions with Reference to Soybean. Int. J. Food Prop. 2017, 20(52), 2220–2236. DOI: 10.1080/10942912.2017.136910.
- Tang, C. H. Nanostructured Soy Proteins: Fabrication and Applications as Delivery Systems for Bioactives (A Review). Food Hydrocolloids. 2019, 91, 92–116. DOI: 10.1016/j.foodhyd.2019.01.012.
- Aletor, O.; Oshodi, A. A.; Ipinmoroti, K. Chemical Composition of Common Leafy Vegetables and Functional Properties of Their Leaf Protein Concentrates. Food Chem. 2002, 78, 63–68. DOI: 10.1016/S0308-8146(01)00376-4.
- Tenorio, A. T.; Gieteling, J.; De Jong, G. A. H.; Boom, R. M.; Der Goot, A. J. V. Recovery of Protein from Green Leaves: Overview of Crucial Steps for Utilization. Food Chem. 2016, 203, 402–408. DOI: 10.1016/j.foodchem.2016.02.092.
- Hojilla-Evangelista, M. P.; Selling, G. W.; Hatfield, R.; Digman, M. Extraction, Composition, and Functional Properties of Dried Alfalfa (Medicago Sativa L.) Leaf Protein. J. Sci. Food Agric. 2017, 97(3), 882–888. DOI: 10.1002/jsfa.7810.
- Martin, A. H.; Castellani, O.; De Jong, G. A. H.; Bovetto, L.; Schmitt, C. Comparison of the Functional Properties of RuBisCO Protein Isolate Extracted from Sugar Beet Leaves with Commercial Whey Protein and Soy Protein Isolates. J. Sci. Food Agric. 2019, 99(4), 1568–1576. DOI: 10.1002/jsfa.9335.
- Famuwagun, A. A.; Alashi, A. M.; Gbadamosi, S. O.; Taiwo, K. A.; Oyedele, D. J.; Adebooye, O. C.; Aluko, R. E. Comparative Study of the Structural and Functional Properties of Protein Isolates Prepared from Edible Vegetable Leaves. Int. J. Food Prop. 2020, 23(1), 955–970. DOI: 10.1080/10942912.2020.1772285.
- AOAC International. Method 978.204: Crude Protein; Method 930.09: Ethereal Extract; Method 930.10: Crude Fiber; Method 930.05: Ashes; Method 930.04: Moisture. Official Methods of Analysis of AOAC International, 1990, 15th ed. Pp. 40–68. Arlington, Virginia: Association of Official Analytic Chemists International.
- González-Garza, N. G.; Chuc-Koyoc, J. A.; Torres-Castillo, J. A.; García-Zambrano, E. A.; Betancur-Ancona, D.; Chel-Gerrero, L.; Sinagawa-García, S. R. Biofunctional Properties of Bioactive Peptide Fractions from Protein Isolates of Moringa Seed (Moringa Oleifera). J. Food Sci. Technol. 2017, 54(13), 4268–4276. DOI: 10.1007/s13197-017-2898-8.
- Kaushik, P.; Dowling, K.; McKnight, S.; Barrow, C. J.; Wang, B.; Adhikari, B. Preparation, Characterization and Functional Properties of Flax Seed Protein Isolate. Food Chem. 2016, 197, 212–220. DOI: 10.1016/j.foodchem.2015.09.106.
- Laemmli, U. K. Cleavage of Structural Proteins during the Assembly of the Head of Bacteriophage T4. Nature. 1970, 227(5259), 680–685. DOI: 10.1038/227680a0.
- Mir, N. A.; Riar, C. S.; Singh, S. Effect of pH and Holding Time on the Characteristics of Protein Isolates from Chenopodium Seeds and Study of Their Amino Acid Profile and Scoring. Food Chem. 2019, 272, 165–173. DOI: 10.1016/j.foodchem.2018.08.048.
- Achouri, A.; Nail, V.; Boye, J. I. Sesame Protein Isolate: Fractionation, Secondary Structure and Functional Properties. Food Res. Int. 2012, 46(1), 360–369. DOI: 10.1016/j.foodres.2012.01.001.
- Bradford, M. M. A Rapid and Sensitive Method for the Quantitation of Microgram Quantities of Protein Utilizing the Principle of Protein–dye Binding. Anal. Biochem. 1976, 72(1–2), 248–254. DOI: 10.1016/0003-2697(76)90527.
- Lawal, O. S.; Adebowale, K. O.; Adebowale, Y. A. Functional Properties of Native and Chemically Modified Protein Concentrates from Bambarra Groundnut. Food Res. Int. 2007, 40(8), 1003–1011. DOI: 10.1016/j.foodres.2007.05.011.
- Coffman, C. W.; García, V. V. Functional Properties and Amino Acid Content of a Protein Isolate from Mung Bean Flour. J. Food Technol. 1977, 12(5), 473–484. DOI: 10.1111/j.1365-2621.1977.tb00132.x.
- Yuceer, M. Structural and Rheological Characterization of Liquid Egg White Modified with Phospholipase A2 Enzyme. J. Food Process. Preserv. 2020, 00, e14450. DOI: 10.1111/jfpp.14450.
- Timilsena, Y. P.; Adhikari, R.; Barrow, C. J.; Adhikari, B. Physicochemical and Functional Properties of Protein Isolate Produced from Australian Chia Seeds. Food Chem. 2016, 212, 648–656. DOI: 10.1016/j.foodchem.2016.06.017.
- Zheng, T.; Li, X.; Taha, A.; Wei, Y.; Hu, T.; Fatamorgana, P. B.; Zhang, Z.; Liu, F.; Xu, X.; Pan, S., et al. Effect of High Intensity Ultrasound on the Structure and Physicochemical Properties of Soy Protein Isolates Produced by Different Denaturation Methods. Food Hydrocolloids. 2019, 97, 105216–105224. DOI: 10.1016/j.foodhyd.2019.105216.
- Anusha, R.; Deepti, S.; Vinita, P.; Rajendra, K.; Neelam, Y. Process Optimization of Chickpea (Cicer Arietinum L.) Seed Protein Isolates for Functional Foods. Res. J. Biotechnol. 2021, 16(2), 163–172.
- Borgues, T. E. M.; Barbieri, C. M. R.; Neves, V. A.; Apareci, S. M.; Arantes-Pereira, L. Chemical Characteristics and Fractionation of Proteins from Moringa Oleifera Lam. Leaves. Food Chem. 2014, 147, 51–54. DOI: 10.1016/j.foodchem.2013.09.135.
- Codex, A. Norma General Del Codex Alimentarius Para Los Productos Proteínicos Vegetales. Codex. Alimentarius. 1995, 7, 113–120.
- Fasolin, L. H.; Pereira, R. N.; Pinheiro, A. C.; Martins, J. T.; Andrade, C. C. P.; Ramos, O. L.; Vicente, A. A. Emergent Food Proteins – Towards Sustainability, Health and Innovation. Food Res. Int. 2019, 125, 108586–108648. DOI: 10.1016/j.foodres.2019.108586.
- Banik, S.; Biswas, S.; Karmakar, S. Extraction, Purification, and Activity of Protease from the Leaves of Moringa Oleifera. F1000Research. 2018, 7, 1151–1164. DOI: 10.12688/f1000research.15642.1.
- Paula, P. C.; Sousa, D. O. B.; Oliveira, J. T. A.; Carvalho, A. F. U.; Alves, B. G. T.; Pereira, M. L.; Farias, D. F.; Viana, M. P.; Santos, F. A.; Morais, T. C., et al. A Protein Isolate from Moringa Oleifera Leaves Has Hypoglycemic and Antioxidant Effects in Alloxan-Induced Diabetic Mice. Molecules. 2017, 22(2), 271–286. DOI: 10.3390/molecules22020271.
- Jain, A.; Subramanian, R.; Manohar, B.; Radha, C. Preparation, Characterization and Functional Properties of Moringa Oleifera Seed Protein Isolate. Assoc. Food Scientists Technol. 2019, 56, 2093–2104. DOI: 10.1002/jsfa.7810.
- Tarone, A. G.; Fasolin, L. H.; De Assis, P. F.; Dupas, H. M.; Lopes, C. R. Influence of Drying Conditions on the Gelling Properties of the 7S and 11S Soy Protein Fractions. Food Bioprod. Process. 2013, 91(2), 111–120. DOI: 10.1016/j.fbp.2012.11.010.
- Paglarini, C. S.; Martini, S.; Pollonio, M. A. R. Physical Properties of Emulsion Gels Formulated with Sonicated Soy Protein Isolate. Int. J. Food Sci. Technol. 2019, 54(2), 451–459. DOI: 10.1111/ijfs.13957.
- Buchaillot, A.; Caffin, N.; Bhandari, B. Drying of Lemon Myrtle (Backhousia Citriodora) Leaves: Retention of Volatiles Colour. Drying Technol. 2009, 27(3), 445–450. DOI: 10.1080/07373930802683740.
- Devisetti, R.; Sreerama, Y. N.; Bhattacharya, S. Processing Effects on Bioactive Components and Functional Properties of Moringa Leaves: Development of a Snack and Quality Evaluation. J. Food Sci. Technol. 2016, 53, 649–657. DOI: 10.1007/s13197-015-1962-5.
- Ali, M. A.; Yusof, Y. A.; Chin, N. L.; Ibrahim, M. N.; Basra, S. M. A. Drying Kinetics and Colour Analysis of Moringa Oleifera Leaves. Agric. Agric. Sci. Procedia. 2014, 2, 394–400. DOI: 10.1016/j.aaspro.2014.11.055.
- Malik, M. A.; Saini, C. S. Polyphenol Removal from Sunflower Seed and Kernel: Effect on Functional and Rheological Properties of Protein Isolates. Food Hydrocolloids. 2017, 63, 705–715. DOI: 10.1016/j.foodhyd.2016.10.026.
- Adewumi, O. O.; Felix-Minnaar, J. V.; Jideani, V. A. Functional Properties and Amino Acid Profile of Bambara Groundnut and Moringa Oleifera Leaf Protein Complex. Processes. 2022, 10(2), 205. DOI: 10.3390/pr10020205.
- Boye, J.; Zare, F.; Pletch, A. Pulse Proteins: Processing, Characterization, Functional Properties and Applications in Food and Feed. Food Res. Int. 2010, 43(2), 414–431. DOI: 10.1016/j.foodres.2009.09.003.
- Porras, S. J.; Güémes, V. N.; Montañez, S. J. L.; Fernández, M. M. C. Comparative Study of Functional Properties of Protein Isolates Obtained from Three Lupinus Species. Adv. Biores. 2013, 4(4), 106–116. DOI: 10.1007/s13197-014-1298-6.
- Cattan, Y.; Patil, D.; Vaknin, Y.; Rytwo, G.; Lakemond, C.; Benjamin, O. Characterization of Moringa Oleifera Leaf and Seed Protein Extract Functionality in Emulsion Model System. Innovative Food Sci. Emerg. Technol. 2022, 75, 102903. DOI: 10.1016/j.ifset.2021.102903.
- Sun, C.; Wu, W.; Ma, Y.; Min, T.; Lai, F.; Wu, H. Physicochemical, Functional Properties, and Antioxidant Activities of Protein Fractions Obtained from Mulberry (Morus Atropurpurea Roxb.) Leaf. Int. J. Food Prop. 2017, 20(S3), S3311–S3325. DOI: 10.1080/10942912.2016.1238389.
- Aye, P. A.; Adegun, M. K. Chemical Composition and Some Functional Properties of Moringa, Leucaena and Gliricidia Leaf Meals. Agric. Biol. J. North Am. 2013, 4(1), 71–77. DOI: 10.5251/abjna.2013.4.1.71.77.
- Shevkani, K.; Singh, N.; Kaur, A.; Chand, R. J. Structural and Functional Characterization of Kidney Bean and Field Pea Protein Isolates: A Comparative Study. Food Hydrocolloids. 2015, 43, 679–689. DOI: 10.1016/j.foodhyd.2014.07.024.
- Euston, S. E.; Singh, M.; Munro, P. A.; Dalgleish, D. G. Competitive Absorption between Sodium Caseinate and Oil-soluble and Water-soluble Surfactants in Oil-in-water Emulsions. J. Food Sci. 1995, 60(5), 1124–1131. DOI: 10.1111/j.1365-2621.1995.tb06307.x.
- Benelhadj, S.; Gharsallaoui, A.; Degraeve, P.; Attia, H.; Ghorbel, D. Effect of pH on the Functional Properties of Arthrospira (Spirulina) Platensis Protein Isolate. Food Chem. 2016, 194(1), 1056–1063. DOI: 10.1016/j.foodchem.2015.08.133.
- Embaby, H. E.; Swailam, H. M.; Rayan, A. M. Preparation and Physicochemical Properties of Protein Concentrate and Isolate Produced from Acacia Tortilis (Forssk.) Hayne Ssp. Raddiana J. food sci. technol. 2018, 55(2), 489–495. DOI: 10.1007/s13197-017-2957-1.
- McClements, D. J. Protein-stabilized Emulsions. Curr. Opin. Colloid Interface Sci. 2004, 9(5), 305–313. DOI: 10.1016/j.cocis.2004.09.003.
- Feyzi, S.; Varidi, M.; Zare, F.; Varidi, M. J. Fenugreek (Trigonella Foenum Graecum) Seed Protein Isolate: Extraction Optimization, Amino Acid Composition, Thermo and Functional Properties. J. Sci. Food Agri. 2015, 95(15), 3165–3176. DOI: 10.1016/j.foodres.2019.108586.
- Aluko, R. E.; Yada, R. Y. Structure-function Relationships of Cowpea (Vigna Unguiculata) Globulin Isolate: Influence of pH and NaCl on Physicochemical and Functional Properties. Food Chem. 1995, 53(3), 259–265. DOI: 10.1016/0308-8146(95)93931-G.
- Adebowale, K. O.; Lawal, O. S. Foaming, Gelation and Electrophoretic Characteristics of Mucuna Bean (Mucuna Pruriens) Protein Concentrates. Food Chem. 2003, 83, 237–246. DOI: 10.1016/S0308-8146(03)00086-4.
- Zeidanloo, M. H.; Ghavidel, R. A.; Davoodi, M. G.; Arianfar, A. Functional Properties of Grass Pea Protein Concentrates Prepared Using Various Precipitation Methods. J. Food Sci. Technol. 2019, 56, 4799–4808. DOI: 10.1007/s13197-019-03930-3.
- Ogunsina, B. S.; Radha, C.; Govardhan, S. R. S. Physicochemical and Functional Properties of Full‐fat and Defatted Moringa Oleifera Kernel Flour. Int. J. Food Sci. Technol. 2010, 45(11), 2433–2439. DOI: 10.1111/j.1365-2621.2010.02423.x.
- Nicolai, T.; Chassenieux, C. Heat-induced Gelation of Plant Globulins. Curr. Opin. Food Sci. 2019, 27, 18–22. DOI: 10.1016/j.cofs.2019.04.005.
- Deng, Y.; Huang, L.; Zhang, C.; Xie, P.; Cheng, P.; Wang, X.; Li, S. Physicochemical and Functional Properties of Chinese Quince Seed Protein Isolate. Food Chem. 2019, 283, 539–548. DOI: 10.1016/j.foodchem.2019.01.083.
- Vogelsang-O’Dwyer, M.; Bez, J.; Petersen, I. L.; Joehnke, M. S.; Detzel, A.; Busch, M.; Krueger, M.; Ispiryan, L.; O’Mahony, J. A.; Arendt, E. K., et al. Techno-Functional, Nutritional and Environmental Performance of Protein Isolates from Blue Lupin and White Lupin. Foods. 2020, 9(2), 230–254. DOI: 10.3390/foods9020230.
- Al-Kahtani, H. A.; Abou-Arab, A. A. Comparison of Physical, Chemical, and Functional Properties of Moringa Peregrina (Al-yassar or Al-Ban) and Soybean Proteins. Cereal Chem. 1993, 70(6), 619-619.
- Stone, A. K.; Avarmenko, N. A.; Warkentin, T. D.; Nickerson, M. A. Functional Properties of Protein Isolates from Different Pea Cultivars. Food Sci. Biotechnol. 2015, 24, 827–833. DOI: 10.1007/s10068-015-0107-y.
- Xu, Y.; Obielodan, M.; Sismour, E.; Arnett, A.; Alzahrani, S.; Zhang, B. Physicochemical, Functional, Thermal and Structural Properties of Isolated Kabuli Chickpea Proteins as Affected by Processing Approaches. Int. J. Food Sci. Technol. 2017, 52(5), 1147–1154. DOI: 10.1111/ijfs.13400.