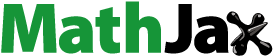
ABSTRACT
Cheddar cheese is a source of a variety of biologically active peptides and free amino acids (FAAs), which contribute to the cheese aroma, flavors, and textures. The FAA liberation and angiotensin-converting enzyme inhibition potential of cheddar cheese made from cow and buffalo milk before and after in vitro digestion by gastric and duodenal enzymes with ripening were investigated. The cheese samples were stored under refrigeration condition (4°C) for ripening for 3, 6, and 9 months. The possible differences existing in the level of FAAs were explored before and after in vitro digestion of ripened cheddar cheese made from cow and buffalo milk. The lower level of angiotensin-converting enzyme inhibition value 62.4 ± 1.04% was observed in gastric digested cheese samples after 9 months of ripening, while the highest angiotensin-converting enzyme inhibition value 93.1 ± 1.29% was observed in duodenal digested cheese samples after 6 months of ripening. This study showed that before and after in vitro digestion, highest angiotensin-converting enzyme inhibitory potential of cheddar cheese was observed between 6 and 9 months of ripening. In 9-month ripened cheese, a significant increase in the contents of glutamic, glutamine, valine, leucine, and lysine was observed after duodenal digestion in case of both cow and buffalo milk Cheddar cheeses. The contents of valine, ornithine, and tryptophan were negligible before digestion and significantly increased afterward.
Introduction
Proteolysis is considered as fundamental biochemical method that occurs throughout ripening process of cheese. Different enzymes present in milk, such as plasmin, pepsin, and chymosin, are responsible for the proteolysis of casein. The proteolytic enzymes are involved in the degradation of casein proteins (as1-, as2-, b-, and k-caseins) into various smaller peptides and free amino acids (FAAs), which are accountable for cheddar cheese flavor development.[Citation1] Different FAAs and their catabolites impart specific flavors which, along with other compounds (e.g., peptides, fat, free fatty acids (FFAs), and salt) contribute to the overall characteristic flavor of different cheese varieties.[Citation2]
Ripening process of cheddar cheese includes the enzymatic digestion of the curd constituents and is known to be very complex biochemical and microbiological process.[Citation3–5] The duration of maturation and variations in temperature are also the key factors that controls the production of flavor compounds, which are responsible for rich flavor in cheddar cheese. The flavors of cheddar cheese are also affected by the composition of the microflora during the ripening process.[Citation6,Citation7]
Milk enzymes, starter lactic acid bacteria (LAB), coagulant, and nonstarter lactic acid bacteria (NSLAB) catalyze the ripening process of cheese.[Citation8,Citation9] Milk constituents present in curd are involved in cheese maturation process during ripening, which leads to the enzymatic degradation of proteins. Chymosin or rennet substitutes, starter bacteria and their enzymes, regular milk enzymes, and enzymes from secondary starter cultures and molds are the major agents involved in cheese ripening.[Citation10,Citation11]
The ripening process results in a particular flavor and textural structures to the various styles of cheese. Cheese are predominantly manufactured by the coagulation of casein proteins by rennet enzymes, which are also known to get involved in the ripening process.[Citation12] The leading biochemical changes in cheese ripening are results of proteolysis, particularly degradation of the casein matrix to amino acids (AAs) and peptides, AA catabolism and subsequent AA degradation, residual lactose metabolism (into citrate and lactate), and also lipolysis, which includes liberation of FFAs.[Citation13] The AA catabolic metabolites directly contribute to the aroma and taste of the final cheese.
On the ripening of cheese, there is an accumulating body of knowledge on the fact that bioactive peptides have been reported to be liberated by the fermentation of dairy products, such as cheese with starter cultures, proteolytic enzymatic hydrolysis, and gastrointestinal digestions of milk .[Citation14] As a main part of rennin angiotensin system, Angiotensin-I-converting enzyme (ACE) helps in regulation of cardiovascular functions and blood pressure.[Citation14] The ACE (peptidyldipeptide hydrolase, EC 3.4.15.1) is considered important in the regulation of blood pressure and cardiovascular function in the rennin-angiotensin system. Angiotensin II (potent vasoconstrictor) was formed when ACE transforms the inactive decapeptide angiotensin I by splitting dipeptide from the C-terminus. The aldosterone (sodium-retentive steroid) was released from adrenal cortex system by the action of Angiotensin II. On the one hand, degradation of bradykinin (vasodilatory nonapeptide) is catalyzed by the activity of ACE, and on the other hand, it was also involved in the degradation of substance P, ekephalins, and neurotensin, which may perhaps interact with the cardiovascular system.[Citation15] Rennin-angiotensin-aldosterone system is one of the several biological pathways that involved in blood pressure regulation in the human body. Association concerning dairy products intake and decrease in blood pressure was reported by several studies.[Citation16] Specific derived compounds with ACE-inhibitory activity from milk proteins of several dairy products were targeted and categorized according to consequent work.[Citation17]
Correlation among proteolysis and ACE inhibition was investigated by Pripp et al.[Citation18] in different cheese varieties, such as Kesam, Gamalost, Norvegia Castello, Pultost, Brie ,and Port Salut, and their results indicated an increase in ACE-inhibition activity during ripening period of cheeses;[Citation18] therefore, it was suggested from the results that cheese is considered as a natural source of ACE inhibitory peptide and undoubtedly other biologically active peptides. According to an investigation, the WSE of cheese comprise most important peptides made throughout ripening.[Citation19] Numerous types of peptides were isolated and identified from the Cheddar cheeses; however, specific peptides were identified to exhibit bioactivities, such as blood pressure lowering properties.[Citation20]
In the complex microbial niche of cheese, LAB, yeast, and some molds are present. These microbes play an important role in the development of the sensory characteristics of cheese and in the technological aspects of cheese production.[Citation21] During the cheese-making process, LAB can be added as a starter culture, contributing to the coagulation of caseins.[Citation22] On the other hand, at least one group of nonstarter LAB (NSLAB; e.g., lactobacilli, pediococci, enterococci, and Leuconostoc) can also be present naturally in cheese. The main source of NSLAB is raw milk, although other cheese ingredients or equipment can also be a source and therefore enhance their concentration in the final product. The NSLAB grow at a very low rate during the first weeks of ripening but eventually dominate the cheese microbiota after the death phase of the starter culture.[Citation23] Both groups of LAB are important for the development of the biochemical characteristics of fresh cheese and for cheese ripening. During the ripening process, LAB release bioactive peptides, EPS, vitamins, CLA, GABA, and oligosaccharides.[Citation24]
This research was conducted to determine the effect of in vitro digestion on the development of bioactive components in Cheddar cheese made from cow and buffalo milk over ripening periods in relation to ACE-inhibition potential as a health promoting bioactivity from functional components of Cheddar cheese.
Materials and methods
Procurement of raw milk and lab chemicals
Cow and buffalo milk were used for Cheddar cheese manufacturing. Cow and buffalo milk were collected from the local farm at Sargodha, Pakistan. Milk from both sources (cow and buffalo) were standardized to 3.5% fat content for cheese manufacturing. Chemicals for analysis were purchased from Sigma Aldrich (Seelze, Germany) and Lab-Scan [Dublin, Ireland) available at local market in Sargodha, Pakistan.
Manufacturing and storage of cheddar cheese
The procedure described by Lawrence et al.,[Citation25] with some modifications, was adapted to make Cheddar cheese. The cheese samples were wrapped in plastic films used for sustaining quality of cheese for the period of storage in order to prevent water loss by dehydration and to preclude the development and occurrence of detrimental microorganisms. The packaging of cheese was done under the supervision of expertise in packaging section at Achha Dairy Industries, Lahore, by means of wrapping films with minimum water vapor diffusion with the use of semi-barrier (low density polyethylene or polypropylene). The storage of cheddar cheese was done in cheese cellar at 4°C for 3, 6, and 9 months at Achha Dairy Industries-Pvt-Ltd., Lahore.
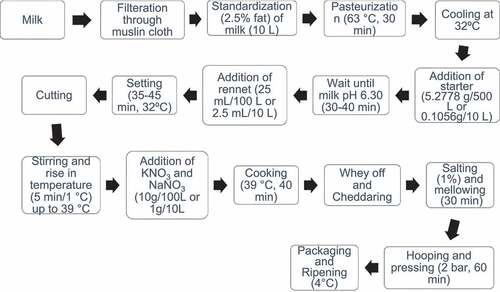
Experimental design of cheddar cheese samples prepared from cow and buffalo milk
Cheeses from batch 1 and batch 2 were prepared from two types of milk, cow and buffalo, respectively. Cheeses from each batch were cut into four identical pieces, and ripening was done for 3, 6, and 9 months at 4°C. One piece was kept fresh in the freezer. Cheese samples from each batch [batch 1 and batch 2) were used for the analysis.
In vitro enzymatic digestion of cheddar cheese
To simulate human digestion in the stomach and the duodenum, an in vitro digestion was performed according to Minekus et al.[Citation26] with some modifications. A volume of 3.8 mL simulated gastric fluid (SGF) (electrolyte stock solution) + 0.2 mL pepsin (made up in SGF electrolyte stock solution) + 25 mL 0.3 M CaCl2 + 975 mL water and 5 mL SGF was used. Enzyme activities are given in units per mL of the final digestion mixture rather than secretion activity, unless stated otherwise. Soluble potato starch was used to measure the activity of amylase (EC 3.2.1.1), e.g. at pH of 6.8–6.9, 1 unit releases maltose (1.0 mg) from starch in 2–3 min at a temperature of 20–21°C. P-toluene-sulfonyl-L-arginine methyl ester (TAME) was used to measure the activity of porcine trypsin (EC 3.4.21.4), e.g. at pH 8.1–8.2, 1 unit hydrolyses 1 μM of TAME/minute at a temperature of 25–26°C. Hemoglobin of bovine blood was utilized as a substrate to measure the activity of porcine pepsin (EC 3.4.23.1), e.g. at pH 2.0–2.1, 1 unit produces a DA280 of 0.001/min at temperature range of 36–37°C (measured as TCA-soluble products). Nbenzoyl-L-tyrosine ethyl ester (BTEE) was used to measure the activity of bovine chymotrypsin (EC 3.4.21.1) e.g., at pH 7.8–7.9, 1 unit hydrolyses 1.0 μM of BTEE/minute at temperature range of 25–26°C. Bile salt concentrations were measured using a commercial kit (e.g., bile acid kit, Diagnostic System GmbH, Germany). Tributyrin was used as a substrate to measure the activity of porcine pancreatic lipase (EC 3.1.1.3), e.g., at pH 8.0, 1-unit releases 1 μM of butyric acid/minute at temperature range of 37–38°C.
Simulated digestion fluids
Electrolyte stock solutions (1.25 concentrated), water, enzymes, and CaCl2 were used for the formulation of simulated salivary fluid, SGF, and simulated intestinal fluid. For example, in the simulated digestion fluids, four portions of electrolyte stock solution + one portion water provide the accurate ionic composition [Adapted from Minekus et al.[Citation26]
Analysis of FAAs of digested and grated cheese samples
FAAs in cheddar cheese and digested cheese samples were determined using the method described by Qureshi et al.[Citation27] According to the procedure, 1.50 g of grated cheese sample was taken in falcon tube with 15 mL of 0.1 N HCl, containing 0.4 µmol/g L-Norvalin (C5H11NO2, Merck Schuchard OHG 85662 Hoheubrunn, Germany). Each cheese sample was mixed thoroughly using Ultra-Turrax machine for 5 min. Then, the samples were placed in sonicating machine (water bath) for 30 min at 37°C (Branson, Soest, Netherlands). After sonication, centrifugation was done at 3500 rpm for 40 min at 4°C (Beckman J2-MC, GMI Inc., MN, USA). After centrifugation, 1000 µL (1 mL) of supernatant was added into 1 mL of trichloroacetic acid (Merck, Darmstadt, Germany) into Eppendorf and mixed on vortex for 2 min (Gene 2, New York, USA). The samples were then placed on ice for 30 min. Centrifugation was done for 5 min at 5°C and 13000 rpm. Filtration was done using syringe filters, and samples were stored in glass vile tubes at −20°C until required for further analysis. FFA contents were determined using RP-HPLC method (Auto-injector 1200 series, Column oven series 200 (Perkin Elmer), Pump series 410 (Perkin Elmer), Thermostat 1200 series, Column (XTerra RP 150*4.6 mm 3.5 µm, Data system: EZChrom Elite Revision 3.3.2 [Agilent) with fluorenyl-methyl-oxy-carbonyl chloride derivatization and o-phthalaldehyde and was done at temperature 42°C.
ACE-Inhibition assay
The ACE-inhibition assay was performed by reversed-phase HPLC according to Qureshi et al.[Citation27] by following the method described by Hyun and Shin[Citation28] with some modifications. Hippuryl-histidyle-leucine (HHL, Sigma, 5 mmol/L] was dissolved in a 0.1 mol/L potassium phosphate buffer of pH 8.3 containing 0.4 molar sodium chloride. A mixture of HHL solution (225 µL) and sample (25 µL) was incubated for 5 min at 37°C. ACE solution (75 µL) was added in the mixture and incubated for 30 min. Sample was filtered with a 0.45 µm, 13-mm syringe filter. The liberated hippuric acid was determined using reversed-phase HPLC [Column oven series 200 (Perkin Elmer), Fluorescence detector series 200 (Perkin Elmer), pump series 200 (Perkin Elmer), Chromatography Interface series 200 (Perkin Elmer), and Auto-sampler series 200 (Perkin Elmer) on Novapak C8 column. The liberated hippuric acid as a result of the reaction between hippuryl-histidyle-leucine (HHL) (substrate) and ACE (enzyme) in the presence or absence (control) of sample was quantified. The percent ACE inhibition was calculated from the following equation:
where hippuric acid concentration was given as HA (control) and hippuric acid was liberated after reaction between substrate (without sample) and enzyme, while HA (sample) stands for hippuric acid released after enzyme and substrate (indicating the presence of sample) reaction.
Statistical analysis
Statistical analysis was performed using Minitab 16 software. The 3-factors factorial design was executed with repeat batches (random variable), age (static variable; with the supposition that the individual cheeses were measured independently from the same batch), and milk type (fixed variable) of Cheddar cheese. Similarly, the 3-factors factorial design was carried out with replicate block (random variable), digestion steps (fixed variable; including undigested samples), and milk type (fixed variable) of Cheddar cheese. Tukey’s test for pairwise comparison was used to test the differences between means.
Results and discussion
FAAs concentration before and after in vitro digestion of cheddar cheese
The effect of in vitro digestion and ripening was assessed on the concentration of FAAs in cheddar cheese. Statistically significant difference was observed for treatments and storage of cheddar cheese (cow and buffalo milk) before and after in vitro digestion from different ripening stages. In case of methionine, non-significant difference was observed for treatments and storage, and the interactive effect of treatment and storage was also non-significant for AA methionine and significant for AA serine.
In cow milk cheddar cheese at 0 day of ripening, the highest mean values were observed for leucine, lysine, tyrosine, and glutamic before digestion. The in vitro digestion affected the concentration of FAA in cheddar cheese, where the contents of glutamic acid, arginine (data not shown), leucine, and lysine were considerably increased, while alanine was significantly decrease after in vitro digestion ()). After 3 months of ripening period, the levels of histidine, citrulline, arginine, alanine, γ-aminobutyric acid (GABA), ornithine, and tryptophan were negligible, but after digestion, the contents of methionine and ornithine significantly increased (data not shown). The contents of glutamic acid, valine, leucine, and lysine significantly increased over the ripening period of 3 months and after digestion ()). After 6 months of ripening, the concentration of all AAs significantly decreased. After digestion, the contents of only glutamic acid, lysine, leucine ()), and valine ()) increased greatly in 6-month ripened cheese (). In 9-month ripened cheese, very low concentration of AAs free were observed; however, AA lysine increased significantly after duodenal digestion () and )).
Figure 1. Dominant (a and b) and minor (c and d) free amino acids of non-digested (a and c) and in vitro duodenal digested (b and d) Cheddar cheese of from cow milk over storage (ripening) of 9 months; values are means of duplicate measurements; legend for (c) and (d) are the same.
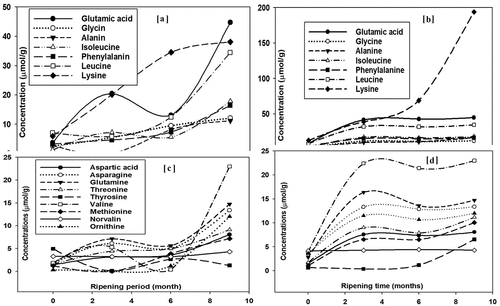
Figure 2. Dominant (a and b] and minor (c and d) free amino acids of non-digested (a and c) and in vitro duodenal digested (b and d) cheddar cheese of from Buffalo milk over storage (ripening) of 9 months; values are means of duplicate measurements; legends for (a) and (b), as well as (c) and (d) are the same.
![Figure 2. Dominant (a and b] and minor (c and d) free amino acids of non-digested (a and c) and in vitro duodenal digested (b and d) cheddar cheese of from Buffalo milk over storage (ripening) of 9 months; values are means of duplicate measurements; legends for (a) and (b), as well as (c) and (d) are the same.](/cms/asset/8fcade45-d2d6-4564-ae1c-9194e32ad4b3/ljfp_a_2070200_f0002_b.gif)
In the cheddar cheese from buffalo milk, before ripening, the highest mean values were observed for leucine, lysine, and glutamic before digestion. The in vitro duodenal digestion affected the concentration of FAA in cheddar cheese with ripening period. The maximum effect of duodenal digestion on increasing concentration of FAA was observed on the level of glutamic, leucine, lysine, and serine. The contents of valine and ornithine (); )), as well as tryptophan (data not shown), were negligible before digestion and significantly increased after digestion. After 3 months of ripening period, the concentration of most AAs prominently decreased except for lysine ()). After duodenal digestion, significant increase in the concentration of all AAs was observed, especially for glutamic acid, lysine, and leucine increasing up to the highest percentage (); )). After 6 months of ripening period, the concentration of AAs again increased in buffalo milk cheddar cheese. Visible effect of ripening was observed on contents of glutamic acid and lysine ()), as well as in glutamine ()). However, digestion significantly raised the content of glutamic acid, leucine, and lysine (); )), as well as valine and tyrosine () and )) over 6 months of ripening of buffalo cheddar cheese. After 9 months of ripening, significant increases in the contents of glutamic acid, leucine, and lysine ()), as well as glutamine and valine ()), were observed for the duodenal digested samples.
Figure 3. Shifts in dominant (a) and minor (b) free amino acids (FAAs) from fresh through three months of ripening all the way to 6–9 months of storage and digestion (DDC); (a) for buffalo and (b) for cow milk cheddar cheeses.
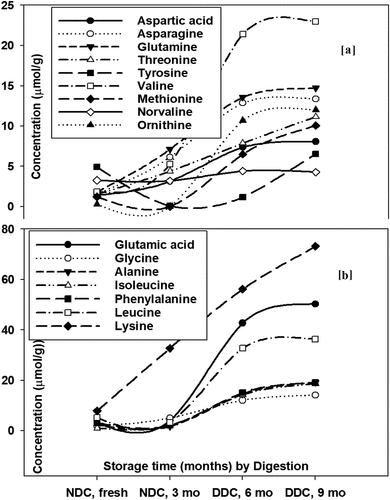
The results from the current investigation were in agreements with Qureshi et al.[Citation29] They studied the concentration of FAAs in Norwegian cheeses (Gamalost and Norvegia) during ripening. It was reported that FAAs, e.g., negligible concentrations of citrulline and γ-aminobutyric acid (GABA), were observed in Norvegia and Gamalost cheeses. The influence of gastric digestion on AA contents was not significant; however, after duodenal digestion, the content of FAA increased significantly. The results from the present study were in line with earlier reports, where the AAs: tyrosine, tryptophan, phenylalanine, arginine, and lysine of Norvegia cheese increased after duodenal digestion.[Citation29] Similarly, tryptophan, phenylalanine, and tyrosine concentrations were enhanced by the gastrointestinal human digestion in Norvegia and Gamalost cheese types in recent studies.[Citation30,Citation31]
ACE-Inhibition and IC50 values of cheddar cheese
The inhibitory activity of ACE was estimated after in vitro gastric and duodenal enzymatic digestions in cow and buffalo milk cheddar cheeses over a ripening period of up to 9 months. In cow milk gastric digested cheddar cheese (GDC), the inhibition (%) values for ACE were 44.2 ± 2.07% for the fresh cheese and significantly increased over ripening to 52.3 ± 1.90%, 57.2 ± 1.30%, and 62.4 ± 1.04% after 3, 6, and 9 months of ripening, respectively (). The highest ACE inhibition value was observed in the samples ripened for 9 months after gastric digestion (GDC). The IC50 values were seen to decrease with increasing percent of ACE inhibition. The IC50 were 2.07 ± 0.01 for the fresh cheddar cheese from cow milk and significantly reduced to 1.90 ± 0.02, 1.30 ± 0.01, and 1.04 ± 0.02 (mg/ml) after 3, 6, and 9 months of ripening, respectively (). After in vitro duodenal digestion (DDC), the ACE inhibition values (%) were 86.2 ± 0.82% for the fresh cheddar and significantly increased to 88.3 ± 1.11%, 93.1 ± 1.29%, and 91.1 ± 1.36% after 3, 6, and 9 months of ripening, respectively, for duodenal digested cheese (DDC) samples. The highest ACE inhibition value was recorded for the samples ripened for 6 months and duodenal digested samples. The IC50 values decreased with increasing percent inhibition of ACE, where it was significantly shifted from 1.42 ± 0.02 for the fresh to 1.25 ± 0.03, 0.85 ± 0.03, and 1.00 ± 0.03 (mg/ml) for the samples ripened for 3, 6, and 9 months, respectively ().
Figure 4. Angiotensin-I-converting enzyme (ACE) inhibition (A) and in vitro gastric digested cheese (GDC) and duodenal digested cheese (DDC) and IC50 (b) Cheddar cheese from cow and Buffalo milk over storage (ripening) of 9-month period; values are means of duplicate measurements; nodes with different connecting letters signify statistically different means and error bars represent standard deviations.
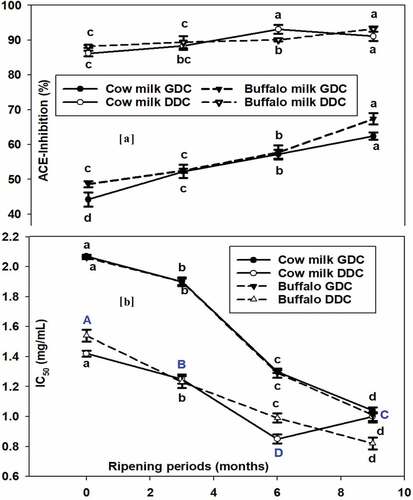
In buffalo milk GDC, the inhibition values for ACE were 48.6 ± 0.87% for the fresh samples and significantly shifted to 52.5 ± 0.55%, 57.7 ± 2.0%, and 67.4 ± 1.59% after ripening for 3, 6, and 9 months, respectively. On the contrary, the IC50 values declined with increasing percent inhibition of ACE (2.06 ± 0.01 to 1.90 ± 0.03, 1.29 ± 0.03, 1.01 ± 0.05 (mg/ml)) over the 3, 6, and 9 months of ripening, respectively. However, in duodenal digested (DDC) buffalo milk cheddar cheese, the inhibition values for ACE were 88.2 ± 0.37% when fresh and slightly, but significantly, increased to 89.4 ± 1.73%, 90.1 ± 0.47%, and 93.2 ± 0.73% after 3, 6, and 9 months of ripening, respectively. The IC50 values decreased with increasing percentage inhibition of ACE from 1.54 ± 0.04 for the fresh cheese to 1.23 ± 0.04, 0.99 ± 0.03, and 0.82 ± 0.04 (mg/ml) for the ripened counterparts for 3, 6, and 9 months, respectively.
The results from the current work were in agreement with that reported by Qureshi et al.[Citation29] The overall implication is that higher values for ACE inhibition are due to the production of more peptides (with potent ACE inhibitory potential) after gastro-duodenal digestion. More peptides are generated after digestion with human gastric and duodenal secretions, which possess additional activities compared with undigested peptides, resulting also in lower IC50 values.
Limited effect of proteolysis was observed on ACE inhibition potential of fresh cheddar cheese.[Citation32] Less extensive proteolysis in products (Quarg) leads to the lower ACE inhibition potential. Ripening over extended periods results in higher dynamism of the peptides and FAA profiles. For instance, it was reported that peptide profiles and the concentrations of individual and total FAAs were influenced by both the adjunct cultures and ripening temperatures.[Citation33] It is obvious that ripening of cheese is affected by numerous processing conditions (cultures, temperature, durations, and cheese styles) and optimal settings, resulting in the highest bioactive components and potent bioactivity, while maintaining the desirable sensorial properties (taste, aroma and texture) is of paramount importance. In line with this, the current work revealed that the ACE-inhibition and IC50 as well as the individual FAA composition of cheddar cheese from cow and buffalo milk are greatly influenced by ripening, and there is a significant shift in the measured parameters before and after gastro-duodenal digestions.
Conclusion
The ripening of cheddar cheese over 9 months resulted in an increase in ACE inhibition and a reduction in IC50 levels in samples from both cow and buffalo milk. There was also a significant shift in the individual FAAs over the ripening and for the GDC and DDC samples. It was pointed out that the ACE -inhibitory potential was highest between 6 and 9 months of ripening of cheddar before and after in vitro digestion. The maximum effect of duodenal digestion on increasing concentration of FAA was observed on the level of glutamic, leucine, lysine, and serine. The observed dynamism presents great potentials of functional cheddar cheese styles as sources of bioactive peptides and FAAs in cardiovascular health in addition to their contributions to the sensorial properties of the products. Research endeavors looking for optimal settings resulting in the highest bioactive components and potent bioactivity, while maintaining the desirable sensorial properties (taste, aroma, and texture) is of paramount importance as ripening of cheese is affected by numerous processing conditions (cultures, temperature, durations, and cheese styles).
Data Availability Statement
Data used in this article will be available on request to the corresponding authors. The dataset supporting the conclusions of this article is included in the manuscript.
Disclosure statement
No potential conflict of interest was reported by the author(s).
Additional information
Funding
References
- Ong, L.; Shah, N. P. Release and Identification of Angiotensin-converting Enzyme-inhibitory Peptides as Influenced by Ripening Temperatures and Probiotic Adjuncts in Cheddar Cheeses. LWT - Food Sci. Technol. 2008, 41, 1555–1566. DOI: 10.1016/j.lwt.2007.11.026.
- McCarthy, C. M.; Kelly, P. M.; Wilkinson, M. G.; Guinee, T. P. Effect of Fat and Salt Reduction on the Changes in the Concentrations of Free Amino Acids and Free Fatty Acids in Cheddar-style Cheeses during Maturation. J. Food Compos. Anal. 2017, 59, 132–140. DOI: 10.1016/j.jfca.2017.01.007.
- Fox, P. F.; Uniacke-Lowe, T.; McSweeney, P. L. H.; O’Mahony, J. A. Chemistry and Biochemistry of Cheese. Dairy Chem. Biochem 2015, 499–546. DOI: 10.1007/978-3-319-14892-2_12.
- Mamo, A. Cheddar Cheese Characterization and Its Biochemical Change during Ripening. Int. J. Adv. Sci. Res. Manag. 2017, 2
- Rahim, M. A.; Khalid, W.; Ranjha, M. M. A. N.; Ambreen, S.; Fizza, C.; Tariq, A.; Ali, M.; Hasan, A.; Rauf, A.; Aziz, A. Nutritional Composition and Medicinal Properties of Camel Milk, and Cheese Processing. Int. J. Biosci. 2020, 17, 83–98. DOI: 10.12692/ijb/17.4.83-98.
- Mayo, B.; Rodríguez, J.; Vázquez, L.; Flórez, A. B. Microbial Interactions within the Cheese Ecosystem and Their Application to Improve Quality and Safety. Foods. 2021, 10, 602. DOI: 10.3390/FOODS10030602.
- Quijada, N. M.; Schmitz-Esser, S.; Zwirzitz, B.; Guse, C.; Strachan, C. R.; Wagner, M.; Wetzels, S. U.; Selberherr, E.; Dzieciol, M. Austrian Raw-Milk Hard-Cheese Ripening Involves Successional Dynamics of Non-Inoculated Bacteria and Fungi. Foods. 2020, 1851, 9, 18519. DOI: 10.3390/FOODS9121851.
- Levante, A.; De Filippis, F.; La Storia, A.; Gatti, M.; Neviani, E.; Ercolini, D.; Lazzi, C. Metabolic Gene-targeted Monitoring of Non-starter Lactic Acid Bacteria during Cheese Ripening. Int. J. Food Microbiol. 2017, 257, 276–284. DOI: 10.1016/j.ijfoodmicro.2017.07.002.
- Ranjha, M. M.; Shafique, B.; Batool, M.; Kowalczewski, P. Ł.; Shehzad, Q.; Usman, M.; Manzoor, M. F.; Zahra, S. M.; Yaqub, S.; Aadil, R. M. Nutritional and Health Potential of Probiotics: A Review. Appl. Sci. 2021. DOI: 10.3390/app112311204.
- Fox, P. F.; Guinee, T. P.; Cogan, T. M.; McSweeney, P. L. H. Fundamentals of Cheese Science. In Biochemistry of Cheese Ripening; Springer: Boston:MA, 2017; pp 391–442. DOI:10.1007/978-1-4899-7681-9_12.
- Nayik, G. A.; Jagdale, Y. D.; Gaikwad, S. A.; Devkatte, A. N.; Dar, A. H.; Dezmirean, D. S.; Bobis, O.; Ranjha, M. M. A. N.; Ansari, M. J.; Hemeg, H. A., et al. Recent Insights into Processing Approaches and Potential Health Benefits of Goat Milk and Its Products: A Review. Front. Nutr. 2021, 8, 789117. DOI: 10.3389/fnut.2021.789117.
- Britten, M.; Giroux, H. J. Rennet Coagulation of Heated Milk: A Review. Int. Dairy J. 2021, 105179, DOI:10.1016/j.idairyj.2021.105179. in Press.
- Blaya, J.; Barzideh, Z.; LaPointe, G. Symposium Review: Interaction of Starter Cultures and Nonstarter Lactic Acid Bacteria in the Cheese Environment1. J. Dairy Sci. 2018, 101, 3611–3629. DOI: 10.3168/jds.2017-13345.
- Wu, C. H.; Mohammadmoradi, S.; Chen, J. Z.; Sawada, H.; Daugherty, A.; Lu, H. S. Renin-angiotensin System and Cardiovascular Functions. Arterioscler. Thromb. Vasc. Biol. 2018, 38. DOI: 10.1161/ATVBAHA.118.311282.
- Li, G. H.; Le, G. W.; Shi, Y. H.; Shrestha, S. Angiotensin I–converting Enzyme Inhibitory Peptides Derived from Food Proteins and Their Physiological and Pharmacological Effects. Nutr. Res. 2004, 24(7), 469–486. DOI: 10.1016/S0271-5317(04)00058-2.
- López-Fandiño, R.; Otte, J.; Van Camp, J. Physiological, Chemical and Technological Aspects of Milk-protein-derived Peptides with Antihypertensive and ACE-inhibitory Activity. Int. Dairy J. 2006, 16(11), 1277–1293. DOI: 10.1016/j.idairyj.2006.06.004.
- Korhonen, H.; Pihlanto, A. Bioactive Peptides: Production and Functionality. Int. Dairy J. 2006, 16(9), 945–960. DOI: 10.1016/j.idairyj.2005.10.012.
- Pripp, A. H.; Sørensen, R.; Stepaniak, L.; Sørhaug, T. Relationship between Proteolysis and angiotensin-I-converting Enzyme Inhibition in Different Cheeses. LWT Food Sci. Technol. 2006, 39(6), 677–683. DOI: 10.1016/j.lwt.2005.03.018.
- Gupta, A.; Mann, B.; Kumar, R.; Sangwan, R. B. ACE-inhibitory Activity of Cheddar Cheeses Made with Adjunct Cultures at Different Stages of Ripening. Adv Dairy Res. 2013, 1(1), 102.
- Fitzgerald, R. J.; Murray, B. A. Bioactive Peptides and Lactic Fermentations. Int. J. Dairy Technol. 2006, 59(2), 118–125. DOI: 10.1111/j.1471-0307.2006.00250.x.
- Irlinger, F.; Mounier, J. Microbial Interactions in Cheese: Implications for Cheese Quality and Safety. Curr. Opin. Biotechnol. 2009, 20, 142–148. DOI: 10.1016/j.copbio.2009.02.016.
- Yang, E.; Fan, L.; Jiang, Y.; Doucette, C.; Fillmore, S. Antimicrobial Activity of Bacteriocin-producing Lactic Acid Bacteria Isolated from Cheeses and Yogurts. AMB Express. 2012, 2, 48. DOI: 10.1186/2191-0855-2-48.
- Zuljan, F. A.; Mortera, P.; Alarcón, S. H.; Blancato, V. S.; Espariz, M.; Magni, C. Lactic Acid Bacteria Decarboxylation Reactions in Cheese. Int. Dairy J. 2016, 62, 53–62. DOI: 10.1016/j.idairyj.2016.07.007.
- Beermann, C.; Hartung, J. Physiological Properties of Milk Ingredients Released by Fermentation. Food Funct. 2013, 4, 185–199. DOI: 10.1039/C2FO30153A.
- Lawrence, R. C.; Gilles, J.; Creamer, L. K.; Crow, V. L.; Heap, H. A.; Honoré, C. G.; Johnston, K. A.; Samal, P. K. Cheddar Cheese and Related Dry-salted Cheese Varieties. Cheese Chem. Phys. Microbiol. 2004, 2, 71–102. DOI: 10.1016/S1874-558X(04)80040-X.
- Minekus, M.; Alminger, M.; Alvito, P.; Ballance, S.; Bohn, T.; Bourlieu, C.; Carrière, F.; Boutrou, R.; Corredig, M.; Dupont, D., et al. A Standardised Static in Vitro Digestion Method Suitable for Food-an International Consensus. Food Funct. 2014, 5, 1113–1124. DOI: 10.1039/c3fo60702j.
- Qureshi, T. M.; Vegarud, G. E.; Abrahamsen, R. K.; Skeie, S. Characterization of the Norwegian Autochthonous Cheese Gamalost and Its Angiotensin I-converting Enzyme (ACE) Inhibitory Activity during Ripening. Dairy Sci. Technol. 2012, 92, 613–625. DOI: 10.1007/s13594-012-0078-1.
- Hyun, C. K.; Shin, H. K. Utilization of Bovine Blood Plasma Proteins for the Production of Angiotensin I Converting Enzyme Inhibitory Peptides. Process Biochem. 2000, 36, 65–71. DOI: 10.1016/S0032-9592(00)00176-X.
- Qureshi, T. M.; Vegarud, G. E.; Abrahamsen, R. K.; Skeie, S. Angiotensin I-converting Enzyme-inhibitory Activity of the Norwegian Autochthonous Cheeses Gamalost and Norvegia after in Vitro Human Gastrointestinal Digestion. J. Dairy Sci. 2013, 96, 838–853. DOI: 10.3168/jds.2012-5993.
- Baptista, D. P.; Gigante, M. L. Bioactive Peptides in Ripened Cheeses: Release during Technological Processes and Resistance to the Gastrointestinal Tract. J. Sci. Food Agric. 2021, 101, 4010–4017. DOI: 10.1002/JSFA.11143.
- Martini, S.; Conte, A.; Tagliazucchi, D. Effect of Ripening and in Vitro Digestion on the Evolution and Fate of Bioactive Peptides in Parmigiano-Reggiano Cheese. Int. Dairy J. 2020, 105, 104668. DOI: 10.1016/J.IDAIRYJ.2020.104668.
- Stuknyte, M.; Cattaneo, S.; Masotti, F.; De Noni, I. Occurrence and Fate of ACE-inhibitor Peptides in Cheeses and in Their Digestates following in Vitro Static Gastrointestinal Digestion. Food Chem. 2015, 168, 27–33. DOI: 10.1016/j.foodchem.2014.07.045.
- Sahingil, D.; Hayaloglu, A. A.; Kirmaci, H. A.; Özer, B.; Simsek, O. Changes of Proteolysis and angiotensin-I Converting Enzyme-inhibitory Activity in White-brined Cheese as Affected by Adjunct Culture and Ripening Temperature. J. Dairy Res. 2014, 81, 394–402. DOI: 10.1017/S0022029914000326.