ABSTRACT
Chilling injury is the dominant factor for quality deterioration and marketability suffers of cucumber fruit during low-temperature storage. Nitric oxide (NO) is a kind of endogenous signaling molecule that significantly regulates the abiotic stress response. In the current investigation, the membrane lipid and energy metabolism were investigated after cucumber fruit was exposed to a sodium nitropruside (SNP) solution that acts as a NO donor before being kept at 4°C for 12 days. The results illustrated that SNP treatment reduced chilling injury and lightened the increase in membrane permeability under cold stress, maintaining a better quality. Subsequently, SNP regulated lipid metabolism by reducing the expression of genes encoding PLA, PLD, lipase, and LOX and decreasing the activities. Compared to the control, the SNP-treated fruit exhibited higher ATP and EC (energy charge) levels. Moreover, SNP-treated suppressed the decrease of H+-ATPase, Ca2+-ATPase, SDH, and CCO activity, which is involved in energy metabolism. The above results showed that exogenous nitric oxide might be an effective method to alleviate the chilling damage in postharvest cucumber fruit by modifying membrane lipids and energy metabolism.
Introduction
Cucumber (Cucumis sativus L.) is popular with consumers for its crisp taste, unique aroma, and high nutritional value. Low-temperature storage is one of the most efficient ways to preserve the quality and extend the storage time of fruit and vegetables.[Citation1] However, cucumber is sensitive to cold, resulting in improper cold storage below 7 ~ 10°C, namely chilling injury.[Citation2] Therefore, it is crucial to improve chilling resistance and postpone chilling injury in postharvest cucumber fruit with proper treatments.
Generally, the cell membrane was found to be the first to sense cold and undergo a phase change when plants are subjected to low-temperature stress[Citation3]; as a result, the phase changed from liquid crystal to a gelatinous state, followed by a series of physiological disorders due to membrane damage. Cold stress leads to the increase and destruction of membrane permeability and the exosmosis of electrolytes in plant cells, while chilling injury leads to the degradation of phospholipids. Phospholipase D (PLD) can hydrolyze phospholipids to phosphatidic acid (PA), resulting in abnormal membrane curvature and dysfunction.[Citation4] Phospholipases PLC and PLA can also catalyze the degradation of glycerophospholipids at low temperature, causing irreparable membrane damage.[Citation4] In addition, phospholipase and lipoxygenase (LOX) closely interact with membrane lipid peroxidation. LOX, in particular, primarily uses the polyunsaturated fatty acids of the plasma membrane as substrates to produce a peroxide with conjugated double bonds from linoleic acid, linolenic acid, and arachidonic acid. The unsaturated fatty acid in fruit and vegetables can maintain cell membrane stability under low temperature, protect cell structure and reduce chilling injury. The lowering in unsaturation of fatty acids will influence the fluidity and structural integrity of the cell membrane, resulting in membrane lipid phase transition and reduced stress resistance.[Citation5]
Energy metabolism plays a key role in respiration, which involves fruit and vegetable ripening and senescence after harvest, and quality decline during storage.[Citation6] Even so, membrane lipid phase transition leads to a disorder of mitochondrial function, which decreases energy utilization efficiency under low-temperature stress. Therefore, it is considered that energy deficit is the direct cause of the chilling injury of fruit.[Citation5] Previous works have reported that changes in energy status are generally detected by ATP, ADP, AMP concentration, and energy charge (EC). Such changes proved to enhance the chilling tolerance of nectarine,[Citation7] zucchini,[Citation8] and mango[Citation9] by improvement of energy status. Moreover, exogenous ATP treatment inhibited browning “Nanguo” pears in relation to an increase in ATP content and energy charge.[Citation10] H+-ATPase, Ca2+-ATPase, succinic dehydrogenase (SDH), and cytochrome c oxidase (CCO) are critical enzymes in mitochondrial energy metabolism and participate in energy synthesis and supply. Studies also indicated that several postharvest applications had been examined to boost the activity and gene expression of energy metabolism-related enzymes, which may account for eased chilling injury, as demonstrated in pears[Citation10] and bananas.[Citation11]
Nitric oxide (NO), an important signaling molecule in plants, is involved in growth and development, fruit ripening and senescence, and stress resistance.[Citation12] The application of endogenous NO can shield plant cells from oxidative stress and prevent the structural damage of cell membranes by suppressing the accumulation of reactive oxygen species (ROS). Yang et al.[Citation13] found that NO slowed the membrane lipid peroxidation by improving the antioxidant system, and the chilling resistance of cucumber fruit. Furthermore, NO exposure reduced CI and enhanced catabolism of polyamines (PAs), γ-aminobutyric acid (GABA), and proline in bananas.[Citation14] NO also could inhibit the development of CI by sustaining the energy status at a high level and stimulating enzyme activity involved in the process of energy metabolism during chilling storage.[Citation15] It has been reported that NO treatment alleviated CI symptoms in fruit like peach,[Citation16] zucchini,[Citation17] orange,[Citation18] and banana.[Citation14] However, there is a paucity of information on the mechanism by which nitric oxide regulates the metabolism of energy and membrane lipids in cucumber fruit. Hence, the current investigation built upon earlier work to furnish more physiological support for this idea and shed more light on the mechanism by which nitric oxide initiates low-temperature tolerance.
Materials and methods
Fruit materials and treatments
Cucumber fruit (Cucumis sativus L. cv Fengshou) were plucked in the morning from a local farm in Hezhou, Guangxi, China. All fruit were delivered to the lab in a vehicle with air-conditioning in less than two hours. Fruit that were uniform in size, color, and absence of defects, mechanical damages, and illness symptoms were chosen. With 150 fruit each, the cucumbers were randomly separated into two groups.
The first group received a spray with 1.0 μM SNP (sodium nitroprusside dihydrate, nitric oxide donor) solution, which was chosen based on our previous research.[Citation19] Simultaneously, the second group applied distilled water (DW, control) in the same way. All cucumbers were allowed to fumigate at ambient temperature for 6 h, wrapped in polyethylene bags, and dry. The fruits were then packaged in polyethylene bags (250 × 170 mm, thickness 0.6 mm) and stored at 4 ± 1°C for 12 d in the dark before being moved to 20°C for 2 d to observe the shelf life and determine the degree of the severity of the chilling injury. Peel tissues were collected every 3 days, frozen in liquid nitrogen immediately, and then preserved at −80°C for further use. There were three replications of each treatment.
Evaluation of severity of chilling injury
Cucumber chilling injury (CI) is characterized by surface pitting and dark watery patches.[Citation19] The CI index, measured from a scale of 0 to 4 [(0, no CI; 1, mild injury (<20%); 2, moderate injury (20%-35%); 3, severe injury (35%-50%); extremely injury (>50%)], was calculated from the size of CI spot area on the epicarp surface that develops during storage. The formula used to determine the CI index was as follows:
CI index (%) = [Σ (CI grade × number of cucumbers on that grade)/(4 × total number of cucumbers in each group)] × 100%
Assessment of membrane permeability
Relative electrolyte leakage (REL), a measure of membrane permeability, was reflected using a method similar to the previously described[Citation20] with slight adjustments. Twenty discs (8 mm diameter, 1 mm thick) of cucumber peel tissue were taken for each replicate using a steel borer, rinsed with distilled water once, and then incubated in 25 mL deionized water at 25°C for 1 h. The initial electrical conductivity (E1) of the solution with discs was written down by a conductivity meter (DDS-307A, Shanghai Scientific Instruments, China). Thereafter, the incubated solutions with discs were in a boiling water bath for 20 min, cooled to 25°C, then read the total electrical conductivity (E2). The REL was calculated as the formula: The REL (%) = (E1/E2) × 100%.
Determination of lipid metabolic enzymes activity
Methods for measuring the activities of phospholipase A (PLA), phospholipase D (PLD), lipase, and lipoxygenase (LOX) were performed on one gram of powdered peel tissue using nearly identical protocols to those published in previous papers with a few changes.[Citation21]
For PLA activity, the peel sample was mixed in 5.0 mL of 50 mmol·L−1 pre-cold Tris-HCl buffer (pH 8.0) and centrifuged at 4°C 15,000 ×g for 15 min. 0.5 mL crude enzyme was added into 2.5 mL reaction solution (50 mmol·L−1 Tris-HCl buffer, pH 8.0, containing 0.3 g/L lecithin, 20 mmol·L−1 CaCl2, 0.2 mol·L−1 NaCl, 50 μmol·L−1 phenol red, 2% Triton X-100), the mixture was adjusted to pH = 8.5 with NaOH solution before further use. The reaction was stopped by adding 1 mL of 95% ethanol after the mixture had been incubated for 15 min at 40°C.
For PLD, peel powder was homogenized in 5.0 mL of 0.1 mol·L−1 ice-cold acetic acid buffer (pH 5.6), and centrifuged at 4°C 12,000 ×g for 15 min. To a reaction solution of the same volume, 3.0 mL of supernatant was added. The mixture was agitated at 28°C 180 r/min for 1 hour before being rinsed three times with petroleum ether and collecting the water layer. After adding 1.0 mL 1% Reinecke salt, the samples were centrifuged at 12,000 ×g for 20 min. The complete dissolution of the precipitate in 5 mL of acetone.
The LOX activity assay utilized 0.1 mol·L−1 sodium phosphate buffer (pH 6.8) to extract the well-ground samples. The mixture underwent a 30 min centrifugation at 4°C and 12,000 ×g. Mixing 2.7 mL of sodium phosphate (pH 6.8), 0.1 mL of sodium linoleate (10 mmol·L−1), and 0.2 mL of supernatant, were then incubated for 10 min at 30°C.
For the lipase activity, the peel sample was extracted with 5 mL of 0.2 mol·L−1 pre-cooled potassium phosphate buffer (pH 7.8) and centrifugated at 4°C 10000 ×g for 15 min. The supernatant was removed, and 0.2 mL it was combined with 0.5 mL 0.2 mmol·L−1 α- naphthalene acetate and 2.3 mL potassium phosphate buffer. The mixture was entirely reacted at 25°C for 30 min, then 0.1 mL of 0.15% solid blue B was added for color development.
One unit (U) of PLA was defined as the quantity of enzyme required to catalyze the formation of 1 nmol fatty acid at 559 nm. One U of PLD and lipase activities were defined as an absorbance variation of 0.1 at 520 nm per hour and per minute, respectively. One U of LOX is the amount of enzyme responsible for a rise in absorbance of 0.01 at 234 nm per minute. PLA, PLD, lipase, and LOX activity levels were reported in terms of units per gram of fresh weight (U g−1).
Assay of energy status
In a slightly modified version of the approach described by Wang et al.,[Citation21] to detect the levels of adenosine triphosphate (ATP), adenosine diphosphate (ADP), and adenosine monophosphate (AMP). Three grams of fruit peel powder with 6 mL of 0.6 mol·L−1 perchloric acid centrifuging at 12,000 ×g for 20 min at 4°C. To get the pH of the supernatant to 6.5 ~ 6.8, we used 1 mol·L−1 KOH for adjustment. Then, the solution was precipitated KClO4 in the ice bath for 30 min and centrifuged for 5 min with 8000 ×g. The supernatant was diluted to 4 mL before it was filtered using a 0.22 μm fiber filter (Tianjin Jinteng Experimental Equipment Co., Ltd, China). The concentrations of ATP, ADP, and AMP were determined using HPLC (LC-2030C 3D, Shimadzu (China) Co., Ltd) equipped with C18 reversed-phase column (5 μm, 4.6 mm × 250 mm; Shimadzu (China) Co., Ltd) and UV detector. The mobile phase was 50 mmol·L−1 K2HPO4-KH2PO4 buffer (pH 6.8) flowing at a rate of 1.0 mL·min−1. With a 20 μL injection volume, the detecting wavelength is set at 254 nm. The ATP, ADP, and AMP contents were represented as μg·g−1 of fresh weight.
The formula below was used to compute the energy charge (EC): EC = ([ATP] + 1/2 × [ADP])/([ATP] + [ADP] + [AMP]).
Determination of energy metabolic-related enzymes activity
The extraction of mitochondria and H+-ATPase, Ca2+-ATPase, succinate dehydrogenase (SDH), and cytochrome C oxidase (CCO) activities were assayed by a modified method.[Citation8] Cucumber peel powder (three-gram) was homogenized in pre-chilled Tris-HCl extraction buffer. After straining the homogenate through four layers of gauze, we centrifuged the supernatant at 15,000 ×g for 20 min at 4°C. Then, the precipitant was rinsed with 4 mL 10 mmol·L−1 Tris-HCl washing buffer (pH 7.2) and centrifuged at 4000 ×g for 10 min at 4°C. The resultant precipitate dissolved and suspended in 3 mL of the washing solution is mitochondrial crude enzyme solution, which could be stable at 4°C until further usage.
Regarding H+-ATPase activity, mitochondrial crude enzyme solution (0.5 mL) was combined with 2.5 mL 30 mmol·L−1 Tris-HCl buffer (pH 8.0, containing 3 mmol L−1 MgSO4, 50 mmol L−1 NaNO3, 0.1 mmol L−1 Na3VO4, 50 mmol L−1 KCl, 0.1 mmol L−1 (NH4)2MoO4). The reaction was kicked off with 0.1 mL 30 mmol L−1 ATP-Tris (pH 8.0), and then the mixture was left to incubate at 37°C for 20 min. At last, 1 mL TCA (trichloroacetic acid, at a concentration of 55%) was mixed to end the reaction. The Ca2+-ATPase activity was measured analogous to that of H+-ATPase, except that 3 mmol·L−1 Ca(NO3)2 was used in place of MgSO4 in the buffer. The absorption was determined immediately once 0.2 mL of 0.1 mol·L−1 SnCl2 was added to the reaction system. For H+-ATPase and Ca2+-ATPase, one U of activity is defined as the amount of enzyme necessary to produce 1 μmol phosphorus per min at 660 nm.
For SDH assay, 0.3 mL mitochondrial crude enzyme solution was mixed with 2.7 mL 30 mmol·L−1 potassium phosphate buffer (pH 7.4, comprising 1 mL 0.2 mol·L−1 sodium succinate and 1 mL 0.9 mmol·L−1 dichlorophenol-indophenol (DCPIP) after incubating at 30°C for 5 min and cooled at ambient temperature. 0.2 mL 10 mmol·L−1 phenazine methosulfate (PMS) was added before measuring the absorbance value at 600 nm of such mixture. The CCO activity was measured by mixing 0.2 mL of mitochondria crude enzyme solution, 0.04% cytochrome C water solution, and distilled water. After being preheated at 37°C for 2 min, 0.5 mL of 0.4% dimethyl p-phenylenediamine solution was added to observe the change of absorbance at 510 nm. The increase in absorbance by 0.01 per minute at 600 and 510 nm, respectively, was taken to be one U of SDH and CCO. These four enzymes were quantified in terms of their activities in units of U·g−1.
RNA extraction and cDNA synthesis
Total RNA of cucumber peel tissue was isolated using Quick RNA isolation Kit (GK0416–50, Huayueyang Biotechnology Beijing Co., Ltd) according to the manufacturer’s instructions, and quantified with a spectrophotometer and detected by 1% agarose gel electrophoresis to check RNA concentration and integrity. The synthesis of first strand of cDNA was synthesized using total RNA as the template, referring to SuperScript cDNA Synthesis Kit (WX2050, Huayueyang Biotechnology Beijing Co., Ltd).
Gene expression analysis
The measurement of the transcript levels of these genes and the cucumber Actin gene were analyzed using quantitative real-time PCR with BlasTaq™ 2× qPCR MasterMix (Richmond, BC, Canada) and CFX Connect™ Real-Time System (BIO-RAD, USA), according to the manufacturer’s instructions and the following conditions: 95°C for 3 min, 95°C for 10 s, 55°C for 30 s, 72°C for 40 s, 40 cycles. The primers of tested genes were designed based on the identified gene sequences via Primer Premier 6.0 software (PREMIER Biosoft International, Canada), of which sequences are listed in . Triplicate repeats were run for the real-time PCR analysis. All qRT-PCR reactions were normalized with the Ct value corresponding to the Actin gene. Relative expression levels of target genes were compared utilizing the 2−ΔΔCt method. The stated values are an average of three independent biological samples.
Table 1. Primers for real-time q-PCR analysis.
Statistical analysis
Statistics were performed using SPSS 26.0. An independent-sample t-test and one-way ANOVA test were conducted to compare the means and characterize the significant differences.
Results
CI index and REL of cucumber during cold storage
CI index increased fast in control fruit after 12 days of cold storage subjoin 2 days of shelf-life time, while it was lower in SNP-treated fruit (). SNP adequately reduced the CI index by 33% ~ 55% in cold-stored cucumber fruit relative to the control. The REL in the control fruit steadily augmented from an initial value of 19.7 ± 0.32 to a maximum of 39.77 ± 2.5% after 12 d of storage (), while SNP treatment reduced the REL in comparison with the control during the entirety of the storage.
Figure 1. CI index (a) and relative electrolyte leakage (b) in cold stored cucumber fruit. Cucumber fruits were subjected to NO donor SNP (1.0 μM) or water (control). Data presented are mean values ± SD (n = 3). The asterisk denotes statistically significant differences between the treatment and control groups at each time point (*P < .05).
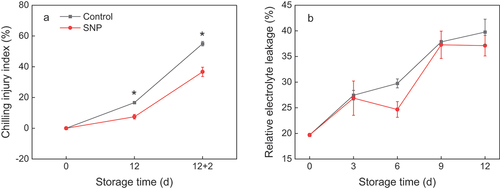
PLA, PLD, lipase and LOX enzyme activities, and gene expression of cucumber during cold storage
The changes in both PLA and PLD activities in the control fruit showed a similar rising trend during the cold storage period, in which SNP-treated fruit differed. As shown in , SNP treatment initially decreased the activities of PLA, reached the minimum on 9 d, and then increased until the end of storage. The PLD activity of SNP-treated fruit increased slightly on 3 d, then declined sharply to a lower level (). The lipase and LOX activity of the control fruit exhibited a general upward trend throughout storage. The SNP application on lipase activity in cucumber fruit displayed a rapid rise in the first 9 days and decreased on 12 d (). However, LOX maintained a stable activity in SNP-fruit during the storage duration ().
Figure 2. The activities of phospholipase A (PLA) (a), phospholipase D (PLD) (b), lipase (c), and lipoxygenase (LOX) (d) in cold stored cucumber fruit. Cucumber fruits were subjected to NO donor SNP (1.0 μM) or water (control). Data presented are mean values ± SD (n = 3). The asterisk denotes statistically significant differences between the treatment and control groups at each time point (*P < .05).
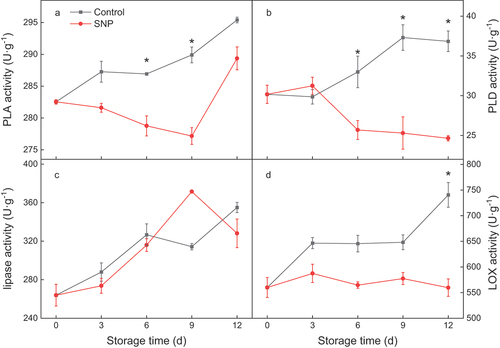
We further detected the expression of lipid metabolic-related enzymes during the storage time. The part of phospholipid degradation CsPLA and CsPLD gene expression was significantly inhibited up to 6 d of storage and consequently exhibited rapid increases during the remaining storage duration in treated fruit, while SNP treatment stimulated a higher transcript of CsPLD gene by 9 d of cold storage relative to the control (). Besides, cucumber lipase gene expression followed an analogous pattern to CsPLA, where treated fruit induced a lower expression level than the control fruit during the low temperature (). Regarding the relative expression of CsLOX, it followed a decreasing trend after 3 d of storage (); during the period, CsLOX expression of SNP-treated was dramatically lower than CK.
Figure 3. The relative expression of CsPLA (a), CsPLD (b), CsLipase (c), and CsLOX (d) in cold stored cucumber fruit. Cucumber fruits were subjected to NO donor SNP (1.0 μM) or water (control). Data presented are mean values ± SD (n = 3). The asterisk denotes statistically significant differences between the treatment and control groups at each time point (*P < .05, **P < .01).
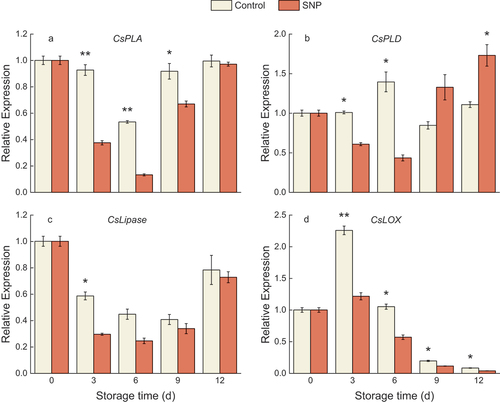
ATP, ADP and AMP contents, and EC
The ATP content of fruit peel tissue was increased at the early stage of the period; however, control fruit and SNP-treated fruit showed a sudden decrease after 6 d or 9 d of storage, respectively. SNP-treated fruit represented a higher and lower ATP content at different time course (). Control fruit manifested similar time courses change patterns in ADP and AMP contents during the whole storage period, which rose at first 6 d and then fluctuated to the peak value at 12 d (). SNP therapy suppressed the increments in ADP and AMP levels, which dropped marginally within 9 days and then rose slightly on 12 d compared to the control group (). EC appears to rise and then suddenly fall in both treatments, which in SNP-treated fruit did deviate from that of control fruit during the initial 6 d, meanwhile maintaining higher than control (). A similar trend to the alteration of ATP contents was seen in the EC of control fruit compared to that of SNP treated fruit throughout the latter stages of storage ().
Figure 4. Adenosine triphosphate (ATP) (a), adenosine diphosphate (ADP) (b) and adenosine monophosphate (AMP) content (c), and energy charge (EC) (d) in cold stored cucumber fruit. Cucumber fruits were subjected to NO donor SNP (1.0 μM) or water (control). Data presented are mean values ± SD (n = 3). The asterisk denotes statistically significant differences between the treatment and control groups at each time point (*P < .05, **P < .01).
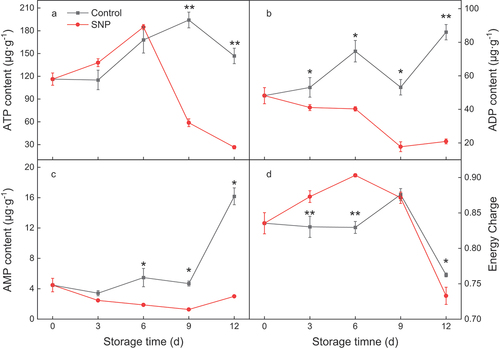
H+-ATPase, Ca2+-ATPase, SDH and CCO activities, and gene expression
The activities of H+-ATPase increased gradually during the assay period in either SNP-treated or control fruit (). SNP treatment considerably enhanced the activity of H+-ATPase in cucumber, while the control fruit maintained a steady trend at the end of storage (). During the storage time, Ca2+-ATPase activity in exogenous SNP-treated fruit was higher 9% to 18% than the control fruit (), although they tend to rise first and then fall slowly. Results of the current study indicated that both the SDH and CCO activities in the SNP-treated and control fruit decreased throughout the storage period. SNP treatment alleviated the declines in activities of SDH and CCO ().
Figure 5. The activities of H+-ATPase (a), Ca2+-ATPase (b), succinate dehydrogenase (SDH) (c), and cytochrome C oxidase (CCO) (d) in cold stored cucumber fruit. Cucumber fruits were subjected to NO donor SNP (1.0 μM) or water (control). Data presented are mean values ± SD (n = 3). The asterisk denotes statistically significant differences between the treatment and control groups at each time point (*P < .05, **P < .01).
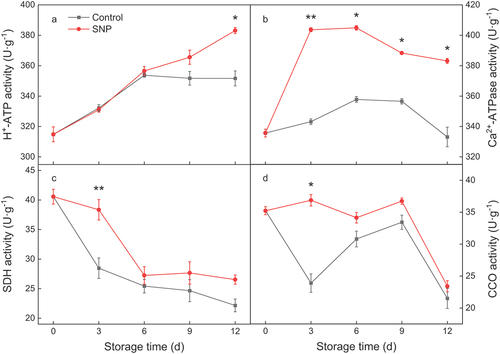
Figure 6. The relative expression of CsH+-ATPase (a), CsCa2+-ATPase (b), CsSDH (c), and CsCCO (d) in cold stored cucumber fruit. Cucumber fruits were subjected to NO donor SNP (1.0 μM) or water (control). Data presented are mean values ± SD (n = 3). The asterisk denotes statistically significant differences between the treatment and control groups at each time point (*P < .05, **P < .01).
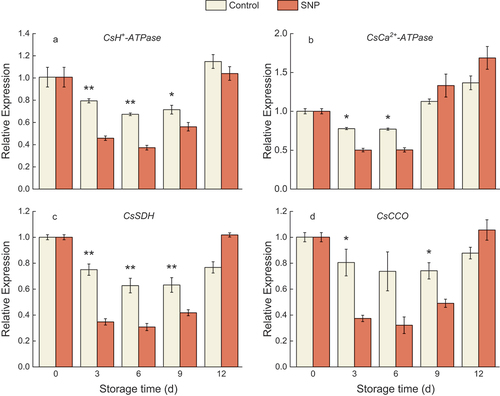
Treated fruit trended lower in CsH+-ATPase relative expression than the control fruit after days of storage. However, it was slightly up-regulated by the end (). Whereas the CsCa2+-ATPase gene expression gradually increased during storage (). As shown in , both CsSDH and CsCCO genes showed decreased transcription levels in SNP-treated fruit during storage except for the 12 d.
Discussion
Low-temperature storage is one of the effective methods to extend the shelf life of fresh produce at present.[Citation22] However, cucumber is a typical cold-sensitive vegetable that can cause CI in an uncomfortable or long-time low-temperature environment. Additionally, the symptoms of CI were not obvious if the cucumber was stored at temperatures lower than 10°C, so it needed to be transferred to room temperature for a while before they appeared, which were characterized by surface water-stained and softening, mostly developed as white lesions on the surface and yellowing, followed by pitting corrosion and infection by pathogenic bacteria.[Citation22] Therefore, it is necessary to alternate a viable strategy to prevent and mitigate the chilling injury of cucumber fruit post-harvest during cold storage to maintain high quality and maximize commodity value. NO, a ubiquitous gas molecule in plants, is an important signal regulator involved in various physiological processes. The previous results showed that NO could improve cell permeability, inhibit chilling injury and delay the browning and aging of fruit and vegetables.[Citation23]
CI onset was delayed and symptoms were alleviated in SNP-treated cucumbers, suggesting that the application of exogenous NO slowed the progression of CI in this study (). Generally accepted as the fundamental cause of chilling injury, the imbalance of membrane lipid metabolism leads to chilling injury.[Citation24] The damage to cell membrane structure is mainly in decreased membrane-binding enzyme ability and increased membrane permeability.[Citation25] Since nitric oxide treatment has a direct impact on cucumber fruit CI, we employed electrolyte leakage to characterize this effect. Exogenous treatment of nitric oxide on cucumber can slow down the rate of electrolyte leakage (), presumably due to maintaining the membrane integrity via adjustment of lipid component and content. However, the relative electrolyte leakage did not show significant differences, except for an obvious reduction on the 6th day after storage, probably due to the large individual variation in cucumber fruit. Similarly, the CI incidence and electrolyte conductivity were sharply decreased during the CI development in NO-treated bamboo shoots,[Citation26] melon,[Citation27] and pomegranate fruit.[Citation28]
Plant phospholipases can catalyze the hydrolysis of glycerophospholipids, resulting in phospholipid degradation and cell membrane damage. Moreover, they are divided into different types according to the different hydrolysis sites. PLA and PLD are commonly activated during phospholipid degradation when the plant or fruit is in the course of cold stress; they play a crucial role in preserving the structure and function of the cell membrane.[Citation29,Citation30] PLAs cleave the sn-1 and/or sn-2 position of glycerophospholipids to release free fatty acids (FFA) and lysolipids, whereas PLDs hydrolyze the phosphodiester bond at the head group side to produce PA and free head groups.[Citation31] PA can be broken down even further to become FFAs with the assistance of lipase.[Citation32] Double bonds in polyunsaturated fatty acids like linoleic acid, linolenic acid, and arachidonic acid are easily oxidized by LOX, forming hydroperoxides and free radicals that can further harm the membrane system.[Citation21] PLA, PLD, lipase, and LOX activated by chilling might promote corresponding physiological processes appertaining to deterioration and senescence over the storage period, and the induction of CI is accompanied by increases in these four enzymes. In this study, cucumbers treated with SNP showed a moderate rise in PLA, PLD, lipase, and LOX activities during storage (). Especially for PLD and LOX, their activities were even suppressed by SNP (), which maintained a better membrane integrity in SNP-treated fruits.
Furthermore, the induction of CsPLA and CsPLD in SNP-treated cucumbers at the initial of storage was repressed (), resulting in decreased PLA and PLD activity. Thus, it can be speculated that NO may alleviate the degradation of cell membrane glycerophospholipid under cold stress, which also reported in NO-treated peaches that the membrane stability is probably enhanced by inducing the gene expression associated with the biosynthesis of fatty acids.[Citation16] In plants, LOX activity increases in response to cold stress; therefore, LOX has been regarded as an imperative factor in free fatty acid metabolism and lipid peroxidation under cold stress, which reveals the reason for membrane damage mainly caused by membrane lipid peroxidation.[Citation33] Showed, as a result, higher lipase and LOX activities were demonstrated to correlate with their gene expression; hence, reducing lipase and LOX activity and expression using SNP () will enhance unsaturated fatty acid levels, retain membrane integrity, and mitigate CI in postharvest cucumber fruit. Similarly, Hu et al.[Citation34] indicated that 24-Epibrassinolide improved chilling tolerance of peach by decreasing the activity and transcription of PLD and LOX. Interestingly, the lipid-related enzymes and genes presented an inconsistent upward or downward trend. It may owe to the post-translation modification from gene to protein.
Previous evidence indicated that energy metabolism is closely referred to the chilling injury of fruits and vegetables after harvest. A higher energy level benefits to the synthesis and repair of cell membrane lipids slows the degree of membrane lipid peroxidation, and inhibits the activities of membrane lipid degrading enzymes.[Citation35,Citation36] In the ongoing study, SNP treatment synthesized ATP at the early stage of cryopreservation and rapidly decomposed (); and kept the high level of EC, then inhibited the increase of ADP and AMP content during low-temperature storage (). It indicates that ATP produced in high amounts in the early stage of low temperature and rapidly degraded at the later stage of storage might be due to the provision of energy material for resistance to low temperature. Lower ADP and AMP content in SNP-treated fruit is related to the less decomposition of ATP leading to a higher EC value. Similar to our findings, exogenous chemical application could higher the energy level, maintain the integrity of cell membrane, and improve the cold resistance of different fruit.[Citation7,Citation10,Citation37]
Not only do the composition and quantity of energy substances alter, but the energy activities involved in energy metabolism, such as ATPase, SDH, and CCO, also fluctuate or decrease when the ambient temperature drops, leading to low energy status.[Citation38] Therefore, it would be advantageous for postharvest fruit quality and CI reduction to express membrane energy-metabolizing enzymes. The ATPase is responsible for decomposing ATP into ADP and free phosphate ions and the subsequent release of energy. Specifically, the H+-ATPase is mainly accountable for hydrolyzing ATP to sustain electrochemical proton potential stability and stop cytosol acidification. Ca2+-ATPase is a Ca2+ transporter driven by ATP hydrolysis, which plays an essential role in regulating cellular Ca2+ homeostasis and effectively protecting the structural integrity of cell membranes under stress.[Citation39] The tricarboxylic acid cycle relies on SDH to oxidize succinate to fumarate and deliver an electron for the respiratory chain, ultimately leading to the generation of ATP inside the mitochondria.[Citation40] CCO serves as the terminal electron acceptor and last enzyme in the mitochondrial respiratory electron transport chain, which is responsible for energy production in the mitochondria through oxidative phosphorylation.[Citation37] In this study, the variations in activity and gene expression are correlated with the shifts in cellular energy and the occurrence of CI. High activities of H+-ATPase and Ca2+-ATPase occurred in SNP-treated cucumber fruit but accompanied the low expression of both CsATPase (). A later onset of CI and more severe CI symptoms were related to lowering SDH and CCO activity and expression (). The results of those enzyme activities in cucumber fruit during cold storage observed in this work indicated postharvest SNP treatment might enhance ATPase activities but alleviate SDH and CCO activities declined, which shows the improvement of chilling tolerance by the increased ATP production and energy release. A comparable pattern has been secured in other fruit including zucchini[Citation8] and nectarine[Citation7] fruit. Recent research showed that, NO treatment effectively alleviated the CI symptoms in bananas, which could be ascribed to the increased activities of H+-ATPase, Ca2+-ATPase, SDH, and CCO. Moreover, a similar result was previously documented in pear fruit, where the exogenous utilization of ATP triggered an increased ATP level and energy charge as well as the up-regulation of expression and activities of ATPase, resulting in reduced CI.[Citation10]
Conclusion
A speculated model illustrates that exogenous nitric oxide alleviated the development of chilling injury by regulating membrane lipid metabolism and energy status (). Nitric oxide alleviated CI, and lipid and energy metabolism are intimately connected to the CI found in cucumber fruit. Nitric oxide treatment might reduce the degradation of phospholipids by reducing the activity of PLA, PLD, lipase, and LOX. At the same time, they exhibited lower expression levels of CsPLA, CsPLD, CsLipase, and CsLOX. Furthermore, the application of nitric oxide increased energy status, possibly owing to the higher activity and transcriptional levels of enzymes participate in energy metabolism, counting H+-ATPase and Ca2+-ATPase, except for SDH and CCO. Therefore, maintaining a high energy level and cellular membrane integrity is crucial to fruit during cold storage. However, more research is required to illuminate the molecular mechanisms of nitric oxide that alleviate the chilling injury in cucumber fruit.
Author contributions
Conceptualization, X.Lu., Y.Liu. and L.Shuai.; methodology, X.Lu. and F.Yin.; software, F.Yin.; validation, X.Lu; formal analysis, X.Lu. and Y.Liang.; investigation, X.Lu., F.Yin. and Y.Liang; resources, L.Shuai.; data curation, Y.Liu. * and L.Shuai.; writing – original draft preparation, X.Lu. and F.Yin.; writing – review and editing, C.Liu., Y.Liu. and L.Shuai.; visualization, X.Lu.; supervision, C.Liu., M.Song. and F.Shang.; project administration, Y.Liu.; funding acquisition, Y.Liu. All authors have read and agreed to the published version of the manuscript.
Disclosure statement
The authors declare that they have no potential conflict of interests or personal relationships that could have appeared to influence the work reported in this paper.
Additional information
Funding
References
- Sharfi, Y.; Aghdam, M. S.; Luo, Z.; Jannatizadeh, A.; Razavi, F.; Fard, J. R.; Farmani, B. Melatonin Treatment Promotes Endogenous Melatonin Accumulation and Triggers GABA Shunt Pathway Activity in Tomato Fruits During Cold Storage. Sci. Hortic. 2019, 254, 222–227. doi: 10.1016/j.scienta.2019.04.056
- Wang, B.; Wu, C.; Wang, G.; He, J.; Zhu, S. Transcriptomic Analysis Reveals a Role of Phenylpropanoid Pathway in the Enhancement of Chilling Tolerance by Pre-Storage Cold Acclimation in Cucumber Fruit. Sci. Hortic. 2021, 288, 110282. doi: 10.1016/j.scienta.2021.110282
- Aghdam, M. S.; Luo, Z.; Li, L.; Jannatizadeh, A.; Fard, J. R.; Pirzad, F. Melatonin Treatment Maintains Nutraceutical Properties of Pomegranate Fruits During Cold Storage. Food Chem. 2020, 303, 125385. doi: 10.1016/j.foodchem.2019.1253854
- Wang, X. Plant Phospholipases. Ann. Rev. Plant Physiol Plant Molecul. Biol. 2001, 52, 1 211–231. doi: 10.1146/annurev.arplant.52.1.211
- Jin, P.; Zhu, H.; Wang, L.; Shan, T.; Zheng, Y. Oxalic Acid Alleviates Chilling Injury in Peach Fruit by Regulating Energy Metabolism and Fatty Acid Contents. Food Chem. 2014, 161, 87–93. doi: 10.1016/j.foodchem.2014.03.103
- Li, D.; Limwachiranon, J.; Li, L.; Du, R.; Luo, Z. Involvement of Energy Metabolism to Chilling Tolerance Induced by Hydrogen Sulfide in Cold-Stored Banana Fruit. Food Chem. 2016, 208, 272–278. doi: 10.1016/j.foodchem.2016.03.113
- Zhang, W.; Zhao, H.; Jiang, H.; Xu, Y.; Cao, J.; Jiang, W. Multiple 1-MCP Treatment More Effectively Alleviated Postharvest Nectarine Chilling Injury Than Conventional One-Time 1-MCP Treatment by Regulating ROS and Energy Metabolism. Food Chem. 2020, 330, 127256. doi: 10.1016/j.foodchem.2020.127256
- Zuo, X.; Cao, S.; Jia, W.; Zhao, Z.; Jin, P.; Zheng, Y. Near-Saturated Relative Humidity Alleviates Chilling Injury in Zucchini Fruit Through Its Regulation of Antioxidant Response and Energy Metabolism. Food Chem. 2021, 351, 129336. doi: 10.1016/j.foodchem.2021.129336
- Li, P.; Zheng, X.; Liu, Y.; Zhu, Y. Pre-Storage Application of Oxalic Acid Alleviates Chilling Injury in Mango Fruit by Modulating Proline Metabolism and Energy Status Under Chilling Stress. Food Chem. 2014, 142, 72–78. doi: 10.1016/j.foodchem.2013.06.132
- Zhang, L.; Wang, J. -W.; Zhou, X.; Shi, F.; Fu, W. -W.; Ji, S. -J. Effect of ATP Treatment on Enzymes Involved in Energy and Lipid Metabolisms Accompany Peel Browning of ‘Nanguo’ Pears During Shelf Life After Low Temperature Storage. Sci. Hortic. 2018, 240, 446–452. doi: 10.1016/j.scienta.2018.06.036.
- Liu, J.; Li, F.; Li, T.; Yun, Z.; Duan, X.; Jiang, Y. Fibroin Treatment Inhibits Chilling Injury of Banana Fruit via Energy Regulation. Sci. Hortic. 2019, 248, 8–13. doi: 10.1016/j.scienta.2018.12.052
- Diao, Q. N.; Song, Y. J.; Shi, D. M.; Qi, H. Y. Nitric Oxide Induced by Polyamines Involves Antioxidant Systems Against Chilling Stress in Tomato (Lycopersicon Esculentum Mill.) Seedling. J. Zhejiang Univ. Sci. B. 2016, 17, 916–930. doi: 10.1631/jzus.B1600102
- Yang, H.; Wu, F.; Cheng, J. Reduced Chilling Injury in Cucumber by Nitric Oxide and the Antioxidant Response. Food Chem. 2011, 127 3, 1237–1242. doi: 10.1016/j.foodchem.2011.02.011
- Wang, Y.; Luo, Z.; Mao, L.; Ying, T. Contribution of Polyamines Metabolism and GABA Shunt to Chilling Tolerance Induced by Nitric Oxide in Cold-Stored Banana Fruit. Food Chem. 2016, 197, 333–339. doi: 10.1016/j.foodchem.2015.10.118
- Wang, Y.; Luo, Z.; Khan, Z. U.; Mao, L.; Ying, T. Effect of Nitric Oxide on Energy Metabolism in Postharvest Banana Fruit in Response to Chilling Stress. Postharvest. Biol. Technol. 2015, 108, 21–27. doi: 10.1016/j.postharvbio.2015.05.007
- Zhao, Y.; Tang, J.; Song, C.; Qi, S.; Lin, Q.; Cui, Y.; Ling, J.; Duan, Y. Nitric Oxide Alleviates Chilling Injury by Regulating the Metabolism of Lipid and Cell Wall in Cold-Storage Peach Fruit. Plant Physiol. Biochem. 2021, 169, 63–69. doi: 10.1016/j.plaphy.2021.10.039
- Jiménez-Muñoz, R.; Palma, F.; Carvajal, F.; Castro-Cegrí, A.; Pulido, A.; Jamilena, M.; Romero-Puertas, M. C.; Garrido, D. Pre-Storage Nitric Oxide Treatment Enhances Chilling Tolerance of Zucchini Fruit (Cucurbita Pepo L.) by S-Nitrosylation of Proteins and Modulation of the Antioxidant Response. Postharvest. Biol. Technol. 2021, 171, 111345. doi: 10.1016/j.postharvbio.2020.111345
- Ghorbani, B.; Pakkish, Z.; Khezri, M. Nitric Oxide Increases Antioxidant Enzyme Activity and Reduces Chilling Injury in Orange Fruit During Storage. N. Z. J. Crop Hortic. Sci. 2017, 2 46, 101–116. doi: 10.1080/01140671.2017.1345764
- Liu, Y.; Yang, X.; Zhu, S.; Wang, Y. Postharvest Application of MeJa and NO Reduced Chilling Injury in Cucumber (Cucumis sativus) Through Inhibition of H2O2 Accumulation. Postharvest. Biol. Technol. 2016, 119, 77–83. doi: 10.1016/j.postharvbio.2016.04.003
- Madebo, M. P.; Luo, S. M.; Wang, L.; Zheng, Y. H.; Peng, J. Melatonin Treatment Induces Chilling Tolerance by Regulating the Contents of Polyamine, γ-Aminobutyric Acid, and Proline in Cucumber Fruit. J. Integr. Agric. 2021, 20 11, 3060–3074. doi: 10.1016/S2095-3119(20)63485-2
- Wang, T.; Hu, M.; Yuan, D.; Yun, Z.; Gao, Z.; Su, Z.; Zhang, Z. Melatonin Alleviates Pericarp Browning in Litchi Fruit by Regulating Membrane Lipid and Energy Metabolisms. Postharvest. Biol. Technol. 2020, 160: 111066. doi: 10.1016/j.postharvbio.2019.111066
- Luo, Z.; Li, D.; Du, R.; Mou, W. Hydrogen Sulfide Alleviates Chilling Injury of Banana Fruit by Enhanced Antioxidant System and Proline Content. Sci. Hortic. 2015, 183, 144–151. doi: 10.1016/j.scienta.2014.12.021
- Kaya, C.; Ashraf, M.; Alyemeni, M. N.; Ahmad, P. The Role of Endogenous Nitric Oxide in Salicylic Acid-Induced Up-Regulation of Ascorbate-Glutathione Cycle Involved in Salinity Tolerance of Pepper (Capsicum Annuum L.) Plants. Plant Physiol. Biochem. 2020, 147, 10–20. doi: 10.1016/j.plaphy.2019.11.040
- Lyons, J. M. Chilling Injury in Plants. Annu. Rev. Plant Biol. 1973, 24, 1 445–466. doi: 10.1146/annurev.pp.24.060173.002305
- Lu, W. J.; Chen, J. Y.; Shan, W.; Min, T.; Deng, W.; Chen, Q. F.; Ji, S. J.; Kuang, J. F.; Liang, S. M. The Membrane Lipid Metabolism in Horticultural Products Suffering Chilling Injury. Food Qual. Saf. 2020, 4, 1 9–14. doi: 10.1093/fqsafe/fyaa001
- Wang, D.; Li, L.; Xu, Y.; Limwachiranon, J.; Li, D.; Ban, Z.; Luo, Z. Effect of Exogenous Nitro Oxide on Chilling Tolerance, Polyamine, Proline, and Gamma-Aminobutyric Acid in Bamboo Shoots (Phyllostachys Praecox F. Prevernalis). J. Agric. Food Chem. 2017, 65, 28 5607–5613. doi: 10.1021/acs.jafc.7b02091
- Zhang, T.; Che, F.; Zhang, H.; Pan, Y.; Xu, M.; Ban, Q.; Han, Y.; Rao, J. Effect of Nitric Oxide Treatment on Chilling Injury, Antioxidant Enzymes and Expression of the CmCbf1 and CmCbf3 Genes in Cold-Stored Hami Melon (Cucumis Melo L.) Fruit. Postharvest. Biol. Technol. 2017, 127, 88–98. doi: 10.1016/j.postharvbio.2017.01.005
- Ranjbari, F.; Moradinezhad, F.; Khayyat, M. Effect of Nitric Oxide and Film Wrapping on Quality Maintenance and Alleviation of Chilling Injury on Pomegranate Fruit. J. agricultural sci. technol. 2018, 20, 1025–1036.
- Kong, X. M.; Ge, W. Y.; Wei, B. D.; Zhou, Q.; Zhou, X.; Zhao, Y. B.; Ji, S. J. Melatonin Ameliorates Chilling Injury in Green Bell Peppers During Storage by Regulating Membrane Lipid Metabolism and Antioxidant Capacity. Postharvest. Biol. Technol. 2020, 170, 111315. doi: 10.1016/j.postharvbio.2020.111315
- Li, Z. G.; Zeng, H. Z.; Ao, P. X.; Gong, M. Lipid Response to Short-Term Chilling Shock and Long-Term Chill Hardening in Jatropha Curcas L. Seedlings. Acta Physiologiae Plant. 2014, 36, 10 2803–2814. doi: 10.1007/s11738-014-1653-2
- Ali, U.; Lu, S.; Fadlalla, T.; Iqbal, S.; Yue, H.; Yang, B.; Hong, Y.; Wang, X.; Guo, L. The Functions of Phospholipases and Their Hydrolysis Products in Plant Growth, Development and Stress Responses. Prog. lipid res. 2022, 86, 101158. doi: 10.1016/j.plipres.2022.101158
- Paliyath, G.; Droillard, M. J. The Mechanisms of Membrane Deterioration and Disassembly During Senescence. Plant Physiol. Biochem. 1992, 30, 789–812.
- Jiao, C.; Chai, Y.; Duan, Y. Inositol 1,4,5-Trisphosphate Mediates Nitric-Oxide-Induced Chilling Tolerance and Defense Response in Postharvest Peach Fruit. J. Agric. Food Chem. 2019, 67 17, 4764–4773. doi: 10.1021/acs.jafc.9b00153
- Hu, S.; Ma, Y.; Xie, B.; Hou, Y.; Jia, Z.; Zhao, L.; Zheng, Y.; Jin, P. 24-Epibrassinolide Improves Chilling Tolerance by Regulating PpCbf5-Mediated Membrane Lipid Metabolism in Peach Fruit. Postharvest. Biol. Technol. 2022, 186, 111844. doi: 10.1016/j.postharvbio.2022.111844.
- Wang, J.; Jiang, Y.; Li, G.; Lv, M.; Zhou, X.; Zhou, Q.; Fu, W.; Zhang, L.; Chen, Y.; Ji, S. Effect of Low Temperature Storage on Energy and Lipid Metabolisms Accompanying Peel Browning of ‘Nanguo’ Pears During Shelf Life. Postharvest. Biol. Technol. 2018, 139, 75–81. doi: 10.1016/j.postharvbio.2018.01.020
- Pan, Y. G.; Yuan, M. Q.; Zhang, W. M.; Zhang, Z. K. Effect of Low Temperatures on Chilling Injury in Relation to Energy Status in Papaya Fruit During Storage. Postharvest. Biol. Technol. 2017, 125, 181–187. doi: 10.1016/j.postharvbio.2016.11.016
- Pan, Y.; Zhang, S.; Yuan, M.; Song, H.; Wang, T.; Zhang, W.; Zhang, Z. Effect of Glycine Betaine on Chilling Injury in Relation to Energy Metabolism in Papaya Fruit During Cold Storage. Food Sci. Nutr. 2019, 7(3), 1123–1130. doi: 10.1002/fsn3.957
- Zhang, W.; Jiang, H.; Cao, J.; Jiang, W. Advances in Biochemical Mechanisms and Control Technologies to Treat Chilling Injury in Postharvest Fruits and Vegetables. Trends Food Sci. Technol. 2021, 113, 355–365. doi: 10.1016/j.tifs.2021.05.009
- Aghdam, M. S.; Jannatizadeh, A.; Luo, Z.; Paliyath, G. Ensuring Sufficient Intracellular ATP Supplying and Friendly Extracellular ATP Signaling Attenuates Stresses, Delays Senescence and Maintains Quality in Horticultural Crops During Postharvest Life. Trends Food Sci. Technol. 2018, 76, 67–81. doi: 10.1016/j.tifs.2018.04.003
- Liu, Z.; Li, L.; Luo, Z.; Zeng, F.; Jiang, L.; Tang, K. Effect of Brassinolide on Energy Status and Proline Metabolism in Postharvest Bamboo Shoot During Chilling Stress. Postharvest. Biol. Technol. 2016, 111, 240–246. doi: 10.1016/j.postharvbio.2015.09.016.