ABSTRACT
The mechanisms underlying the functional link between autophagy and plant innate immunity remain largely unknown. In this study, we investigated the autophagy-mediated plant defense responses against Verticillium dahliae (V. dahliae) infection by comparative proteomics and cellular analyses. An assessment of the autophagy activity and disease development showed that autophagic processes were tightly related to the tolerance of Arabidopsis plant to Verticillium wilt. An isobaric tags for relative and absolute quantification (iTRAQ)-based proteomics analysis was performed, and we identified a total of 780 differentially accumulated proteins (DAPs) between wild-type and mutant atg10-1 Arabidopsis plants upon V. dahliae infection, of which, 193 ATG8-family-interacting proteins were identified in silico and their associations with autophagy were verified for several selected proteins. Three important aspects of autophagy-mediated defense against V. dahliae infection were revealed: 1) autophagy is required for the activation of upstream defense responses; 2) autophagy-mediated mitochondrial degradation (mitophagy) occurs and is an important player in the defense process; and 3) autophagy promotes the transdifferentiation of perivascular cells and the formation of xylem hyperplasia, which are crucial for protection against this vascular disease. Together, our results provide several novel insights for understanding the functional association between autophagy and plant immune responses.
Abbreviations
AIM | = | ATG8-interacting motif |
AT1G02930/GSTF6 | = | glutathione s-transferase 6 |
AT1G17750/PEPR | = | elicitor peptide receptor |
AT1G21100/IGMT1 | = | indole glucosinolate o-methyltransferase 1 |
AT1G33030, AT1G77520/OMT | = | O-methyltransferase protein |
AT1G62820/CML14 | = | calmodulin-like protein 14 |
AT1G64160/DIR5 | = | dirigent protein 5 |
AT1G71930/VND7 | = | vascular related NAC-domain protein 7 |
AT1G73080/PEPR1 | = | PEP1 receptor 1 |
AT1G77120/ADH | = | alcohol dehydrogenase |
AT1G78570/RHM1 | = | rhamnose biosynthesis 1 |
AT1G78870/UBC13A | = | ubiquitin-conjugating enzyme 13A |
AT2G34690/ACD11 | = | accelerated cell death 11 |
AT2G35980/NHL10 | = | NDR1/HIN1-like 10 |
AT2G46370/JAR1 | = | jasmonate-resistant 1 |
AT3G07390/AIR12 | = | auxin-induced in root cultures 12 |
AT3G12500/PR3 | = | pathogenesis-related 3 |
AT3G17910/SURF1 | = | surfeit 1 |
AT3G24503/ALDH1a | = | aldehyde dehydrogenase 1a |
AT3G27280/PHB4 | = | prohibitin 4 |
AT3G28940/AIG2-like | = | avirulence induced gene2-like family protein |
AT3G46010/ADF1 | = | actin-depolymerizing factor 1 |
AT3G49110/PRXCA | = | peroxidase CA |
AT3G50740/ UGT72E1 | = | UDP-glycosyltransferase 72E1 |
AT3G53260/PAL2 | = | phenylalanine ammonia-lyase 2 |
AT3G54640/TRP3 | = | tryptophan-requiring 3 |
AT4G04780/MED21 | = | mediator 21 |
AT4G23100/PAD2 | = | phytoalexin deficient 2 |
AT4G23680, AT4G14060, AT3G26460/MLPs | = | major latex proteins |
AT4G24690/NBR1 | = | next to brca1 gene 1 |
AT4G26840/SUMO1 | = | small ubiquitin-like modifier 1 |
AT4G32260/ PDE334 | = | pigment defective 334 |
AT4G32360/NADPH:AO | = | NADPH:adrenodoxin oxidoreductase |
AT4G32880/HB8 | = | homeobox gene 8 |
AT4G35310/CPK5 | = | calmodulin-domain protein kinase 5 |
AT4G36220/F5H | = | ferulate 5-hydroxylase |
AT4G37390/AUR3 | = | auxin upregulated 3 |
AT5G05730/ASA1 | = | anthranilate synthase alpha subunit 1 |
AT5G06320/ NHL3 | = | NDR1/HIN1-like 3 |
AT5G08640/FLS1 | = | flavonol synthase 1 |
AT5G09978/PEP7 | = | elicitor peptide 7 precursor |
AT5G13370/GH3 | = | auxin-responsive GH3 family protein |
AT5G13930/CHS | = | chalcone synthase |
AT5G24770/VSP2 | = | vegetative storage protein 2 |
AT5G47910/RBOHD | = | respiratory burst oxidase homologue D |
AT5G47990/CYP705A5 | = | cytochrome P450 705A5 |
AT5G57220/CYP81F2 | = | cytochrome p450, family 81, subfamily, polypeptide 2 |
AT5G58430/EXO70B1 | = | exocyst subunit EXO70 family protein B1 |
AT5G66690/UGT72E2 | = | UDPG:coniferyl alcohol glucosyltransferase 72E2 |
ATG | = | autophagy-related |
DAPs | = | differentially accumulated proteins |
dpi | = | days post inoculation |
FDR | = | false discovery rate |
GFP | = | green fluorescent protein |
GO | = | Gene Ontology |
Hai | = | hours after inoculation |
HR | = | hypersensitive response |
iTRAQ | = | isobaric tags for relative and absolute quantitation |
KEGG | = | Kyoto Encyclopedia of Genes and Genomes |
LIR | = | LC3-interacting region |
RABG3B | = | RAB FTPase homolog G3B |
ROS | = | reactive oxygen species |
TEM | = | transmission electron microscopy |
YFP | = | yellow fluorescent protein |
Introduction
Autophagy is an intracellular degradation process that is conserved in a range of eukaryotic cells, from yeasts to higher plants. This process mediates the sequestration of cytosolic macromolecules or disused organelles within a compartment and the subsequent delivery of the contents to the lysosomes or vacuole for degradation [Citation1]. In plants, autophagy acts in many critical biological processes during normal growth and development, and in responses to biotic and abiotic stresses [Citation2-4].
Over the past decade, evidence for the involvement of autophagy in plant immune responses has been accumulating. Liu et al. were the first to find that autophagy is involved in plant immunity in tobacco mosaic virus-inoculated tissues expressing the N resistance gene [Citation5]. Subsequently, an increasing number of studies have reported the association between autophagy and plant innate immunity. However, the action mechanisms appear perplexing and complex [Citation6-8]. It is reported that autophagy functions as either a “prosurvival” factor or a “prodeath” factor in some well-characterized pathogen-plant interactions, depending on the nature of the pathogens. In biotrophic plant-pathogen interactions, autophagy mediates cell death signaling in a receptor-dependent manner or negatively controls salicylic acid (SA) signaling, thus contributing to hypersensitive response (HR)-cell death at infected sites or restricting cell death spreading to uninfected areas [Citation9-14]. In necrotrophic pathogen-plant interactions, autophagy eliminates toxic reactive oxygen species (ROS) accumulation and restricts the ROS-induced spread of necrotic cell death, thus enhancing disease tolerance [Citation7,Citation15,Citation16]. In addition, interactions of defense regulators with autophagy components are important contributors to plant immunity [Citation15,Citation17]. These studies indicate that autophagy plays an essential role in plant innate immune responses to invading microbes with complex action mechanisms. To better elucidate the link between autophagy and plant innate immunity, further investigations are required, including studies focusing on the high-throughput identification of components and cellular processes affected by autophagy during plant defense against pathogen infections [Citation8,Citation18]. Vascular wilt is primarily caused by an infection of soil-borne pathogens, such as fungi and bacteria, and it represents one of the most destructive plant diseases and causes severe crop yield losses worldwide [Citation19]. In recent years, studies on the interactions between vascular pathogens and their plant hosts have uncovered several intriguing features of host defense systems. Specifically, plants may have developed strategies to defend against vascular pathogens. For example, the bacterial pathogen Xanthomonas campestris can trigger vascular-specific HR in Arabidopsis [Citation20] and the Ve disease resistance genes [Citation21] participate in vascular defense against the fungus pathogen Verticillium dahliae (V. dahliae) in tomato and possibly in tobacco [Citation22]. In addition, several receptor proteins act in the recognition of vascular wilt pathogens and the activation of defense responses against the vascular pathogens, including the formation of tyloses, callose and secondary cell wall deposition and the accumulation of different proteins and secondary metabolites, such as pathogenesis-related proteins (PRs), peroxidases and lignin-like components in the xylem sap [Citation19]. To date, the involvement of autophagy in vascular defense has not been reported. Based on the important roles of autophagy in the defense responses of aerial plant parts, investigations of the role of autophagy and the cellular processes associated with its function in root organ may provide new insights and a comprehensive understanding of the molecular mechanisms of plant immune responses.
In this study, we investigated the roles of autophagy in vascular immunity using the model plant Arabidopsis and the fungal pathogen V. dahliae Kleb, a semi-biotrophic soil-borne fungus belonging to the ascomycete family that causes destructive vascular disease in many important crops, such as cotton, peanuts and potatoes worldwide [Citation23-26]. Data obtained from isobaric tags for relative and absolute quantification (iTRAQ)-based proteomics analysis and molecular cell biology investigations have shown that autophagy plays a critical role for host defense against the vascular pathogen V. dahliae in Arabidopsis, and this process has a special association with the activation of defense responses, the degradation of mitochondria and the hyperplasia of xylem cells. Importantly, we identified in silico, a number of ATG8-family-interacting proteins that were responsive to V. dahliae infection, which may provide new candidates for further studies on autophagy-mediated plant immunity.
Results
Activation of autophagy in response to V. dahliae infection in Arabidopsis
As a first step to investigating the role of autophagy in the defense against V. dahliae infection in Arabidopsis, we measured the expression level of the autophagy-related (ATG) gene and the formation of autophagosomes in mock and infected wild-type plants. Because Verticillium wilt is a soil-born vascular disease and the pathogen infects and colonizes from root, the first barrier for defense against this pathogen, we chose root as the organ for the investigation. The expression of all tested ATG genes, including ATG4A, ATG4B, ATG5, ATG7, ATG8A, ATG8E, ATG9, and ATG10, could be induced within a range of 2- to 10-fold in root tissue after V. dahliae (strain V592) infection (A). As ATG8-family proteins have been widely used as a marker to visualize autophagosomes in plant cells [Citation11, Citation27-29] we examined the formation of autophagosomal-like structures in GFP-ATG8E-labeled wild-type plant in response to V. dahliae infection, using the atg10-1 mutant (deficient in autophagosome formation) as a negative control (B). In the wild type (WT), the fluorescence of GFP-ATG8E was hardly detected in the mock-inoculated roots, and autophagosomal-like structures emerged in the root cells at 24 h after inoculation (Hai), with a maximum accumulation occurring at 48 Hai. In contrast, there was no obvious presence of autophagosomal-like structures in both mock and infected roots of the atg10-1 mutant. The data illustrated in C showed this difference quantitatively.
Figure 1. Transcriptional expression and autophagy activity were significantly enhanced after V. dahliae (V592) challenge in wild-type Arabidopsis. (A) Time-course gene expression profile of selected autophagy genes. Hai, hours after inoculation. (B) GFP-ATG8E-labeled autophagosome-like structures at 24, 48, 72 and 96 Hai in wild-type and atg10-1 plants. Bar: 10 μm. (C) Quantification of GFP-ATG8E-labeled autophagosome-like structures. Mean and standard error (SE) were calculated from 15 primary roots from GFP-ATG8E transgenic seedling per time point, and similar results were obtained in 3 independent experiments with roughly 150 investigated cells per time point. WT, wild type. Mock-inoculated controls at 48 Hai were shown as representatives for (B) and (C) because no obvious difference was observed between the mock controls at 24, 48 and 72 Hai. “*” and “**” indicate statistically significant (P ≤ 0.05 or P ≤ 0.01 vs mock), measured by the Student t test.
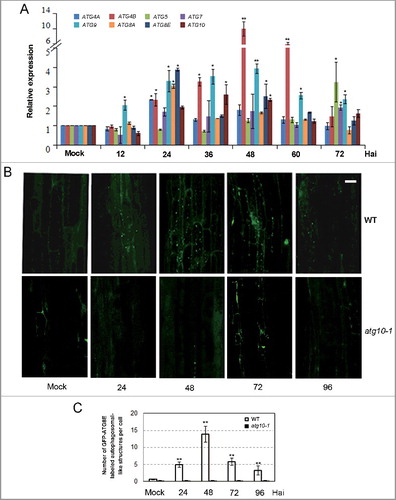
The involvement of autophagy in defending against V. dahliae was further assessed by comparing the disease tolerance of a wild-type Arabidopsis plant and autophagy-deficient mutant plants (atg5, atg7, and atg10-1). The plants were mock-inoculated with water or root-dip inoculated with a V. dahliae (strain V592) spore suspension, and the disease symptoms were assessed. As shown in , growth of all genotypes appeared stunted and presented yellow leaves that finally wilted after V. dahliae inoculation; however, the disease progression varied. For the mutant lines, the yellow leaves appeared 7 to 10 days post inoculation (dpi) and 2 to 5 bottom leaves were totally wilted or dried at 14 dpi, whereas for the wild-type plants, the leaves became yellow approximately 5 d later and wilted leaves were not observed until 14 dpi (A). Consistent with the symptoms, the relative leaf area of the mutant plants was decreased and the fungal DNA amount in the rosette leaves was significantly increased compared with that of the wild-type control (B,C).
Figure 2. Defective autophagy caused higher susceptibility of Arabidopsis plants to V. dahliae (V592) infection. (A) The disease symptom was more serious in autophagy mutants atg5, atg7 and atg10-1 compared with WT. Bar: 1 cm. (B) Comparison of healthy leaf areas for the WT and atg10-1 mutant Arabidopsis plants. “*” and “**” indicate statistically significant (P ≤ 0.05 or P ≤ 0.01 vs mock). (C) Comparison of the V. dahliae DNA levels in V. dahliae-infected WT and autophagy mutants atg5, atg7 and atg10-1. WT, wild type. “*” and “**” indicate statistically significant (P ≤ 0.05 or P ≤ 0.01 vs WT), measured by the Student t test.
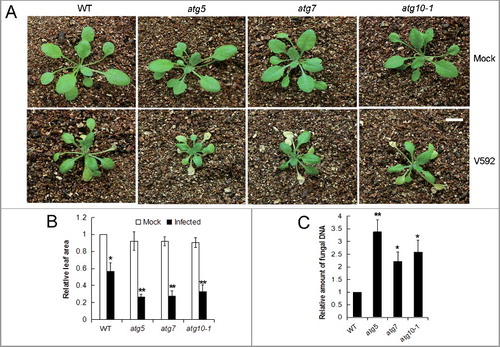
The induction of ATG gene expression and the formation of autophagosomal-like structures in the wild-type Arabidopsis plant and the increased disease susceptibility of the ATG mutants indicated that autophagy plays an important role in the defense against V. dahliae infection in Arabidopsis.
iTRAQ-based identification of V. dahliae-responsive proteins
A comparative proteomics analysis was performed via a multiplex iTRAQ technique to identify autophagy-related proteins and cellular processes/pathways involved in the defense against V. dahliae infection using the wild type (WT) and autophagy mutant atg10-1. ATG10 is a protein that acts as an E2-like enzyme for conjugation between ATG12 and ATG5. The conjugate further forms a protein complex with ATG16, and ATG12-ATG5-ATG16 complex plays an essential role for autophagosome formation [Citation27,Citation30]. We chose atg10-1 for the analysis because it showed a significant attenuation of tolerance upon pathogen attack, but has a weaker phenotype compared to other atg mutants during its growth and development under normal condition. The total proteins were extracted from the roots of the WT and atg10-1 mutant plants at 24, 48 and 72 h after mock or V. dahliae inoculation (based on the time of autophagosome formation). Following labeling, fractionation and separation, a data search identified a total of 5,961 proteins (29% of the total estimated Arabidopsis proteome) from 3 independent biological repeats (5,277, 5,812 and 5,443 proteins, respectively). Of these proteins, 5,669 (95.10%) were identified from at least 2 replicates and 4,940 (82.87%) contained all 3 repeats, thus reflecting the high confidence of the identified proteins (A, Table S2).
Figure 3. iTRAQ-based proteomics identification of proteins in response to V. dahliae infection in the wild-type and atg10-1 Arabidopsis plants. (A) Venn diagram showing the distribution of the number of proteins that were identified from 3 biological repeats. (B) Venn diagram showing the overlap of up- and down-accumulated proteins identified in the WT and atg10-1 mutant. (C and D) Comparison of the number distributions of upaccumulated (C) and downaccumulated (D) proteins identified from the WT and atg10-1 according to the time course. w+/m−, w−/m+ and w+/m+D indicate the same meaning mentioned in the text. w+/m+D (w) indicate proteins both changed, and preferentially changed in WT at more time-points or with higher abandance; w+/m+D (m) indicate proteins both changed, and preferentially changed in mutant at more time-points or with higher abundance. WT, wild type.
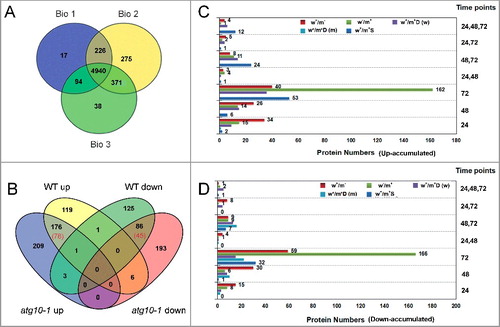
By merging the data obtained from the 3 biological replicates, a total of 919 proteins with log2 ratios of either (≥0.38) or (≤−0.38) were quantified as significant changes in the accumulation levels relative to their respective controls from at least 2 independent experiments (Table S3A to D). As shown in B, 119 upregulated and 125 downregulated proteins were identified only from the WT (indicated as w+/m−); 209 upregulated and 193 downregulated proteins were identified only from the atg10-1 mutant (indicated as w−/m+); 176 upregulated and 86 downregulated proteins were identified from both the WT and atg10-1 mutant, including proteins that displayed different changes in abundance (indicated as w+/m+D, with 78 up, 45 down), and the remaining proteins with similar changes in abundance (indicated as w+/m+S, with 98 up and 41 down). Additionally, there was a small portion (11) of proteins with opposite changes mainly including 6 proteins upregulated in WT and downregulated in atg10-1, 3 proteins downregulated in WT and unregulated in atg10-1. In total, there were 780 differentially accumulated proteins (DAPs) between WT and the mutant upon V. dahliae challenge. Besides, DAPs were also identified between the WT and the atg10-1 mutant under mock inoculation condition (Table S3E), but the numbers were much less (20 up, 30 down). These results indicated that the atg10-1 mutation had a dramatic effect on the V. dahliae-responsive proteome change, thereby causing the alternative accumulation of a large number of proteins. To investigate the time course of proteome changes in response to V. dahliae, we analyzed the numbers of upregulated and downregulated proteins that were identified at 3 different time points. At 24 and 48 Hai (C,D), there were far lower numbers of proteins identified in atg10-1 than in the WT, indicating that the V. dahliae-responsive proteome changes were impacted in the autophagy mutation. At 72 Hai, both the numbers of upregulated and downregulated proteins showed a clear increase (>2-4 X) in the mutant relative to the WT (C,D). These data indicate that the V. dahliae-responsive proteome change delayed in the autophagy mutation and more intensive changes occurred in the mutant at 72 Hai. The turning point was at ∼48 Hai, which is the peak time for autophagosome generation (B).
Verification of iTRAQ data
To verify the changes of protein accumulation measured by iTRAQ analysis, we performed western blot analysis for some selected proteins. As shown in A,B, protein accumulation of 4 proteins (AT1G77120/ADH, AT4G24690/NBR1, AT3G46010/ADF1 and AT4G26840/SUMO1) from iTRAQ analysis was well consistent with the western blot data at each time-point. The iTRAQ data were also verified at the transcription level through qRT-PCR analysis, and trend correlations between protein and mRNA expression levels were detected for most of the tested proteins (). As seen in B–F, proteins and transcripts of 4 genes (AT1G02930/GSTF6, AT5G06320/NHL3, AT3G53260/PAL2 and AT3G28940/AIG2-like) were all upaccumulated at one or multiple time points, and upregulation of their transcriptions was at similar range between the 2 genotypes, as detected at protein levels; transcripts of AT3G54640/TRP3 and AT3G07390/AIR12 were preferentially upaccumulated in WT at one or multiple time points (G,H while those of AT4G37390/AUR3 and AT5G05730/ASA1 were preferentially upaccumulated in atg10-1 mutant (I,J), these trends of changes in transcriptional patterns were similar with those of the protein accumulation patterns; transcripts and proteins of AT5G47990/CYP705A5 were all downregulated, but a more obvious decrease was detected at the protein level in the atg10-1 mutant (K). Only 2 genes (AT3G12500/PR3 and AT5G24770/VSP2) have weak or no correlation of abundance changes between transcription and protein levels (L,M), which was likely because of post-transcriptional regulation as documented in other proteomic studies [Citation31,Citation32].
Figure 4. Verification of the accumulation patterns of iTRAQ identified proteins. (A) Western blot analysis of several V. dahliae-responsive proteins in the roots of wild-type and atg10-1 Arabidopsis plants. CBB, Coomassie Brilliant Blue staining of the gel to show equal loading of proteins. (B) Heat map showing the accumulation changes of selected proteins. Blank spaces are representative of a protein that was identified but did not have a significant change in accumulation relative to the control, M indicated comparison of the 2 mock controls, the fold change was obtained relative to the WT control. (C to M) qRT-PCR analysis of the transcription levels of some selected proteins. The vertical axis in the panels indicates the relative expression change versus mock control of WT. Standard deviation (n = 3) was estimated. “*” and “**” indicate statistically significant (P ≤ 0.05 or P ≤ 0.01 versus mock), “#” and “##” indicate statistically significant (P ≤ 0.05 or P ≤ 0.01 vs atg10-1), measured by the Student t test.
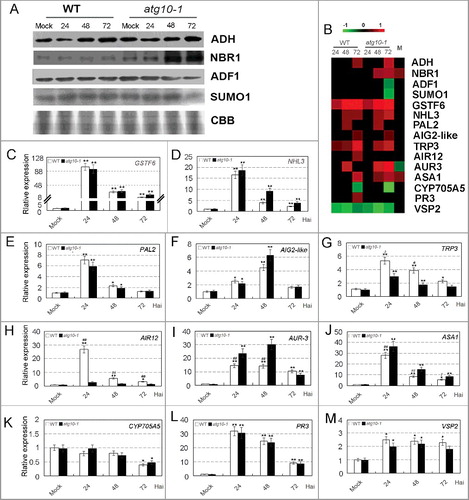
Functional annotation of identified proteins with differential accumulation between WT and atg10-1 mutant
A plant Gene Ontology (GO) slim type analysis was conducted to annotate the proteins with differential accumulation patterns (w+/m−, w−/m+, and w+/m+D) in the 2 plant varieties using the agriGO Single Enrichment Analysis (SEA) tool (http://bioinfo.cau.edu.cn/agriGO) (Q value ≤ 0.01). The results showed that 71 GO terms were enriched for biological processes (BP), 49 GO terms were enriched for cellular components (CC) and 24 GO terms were enriched for molecular functions (MF) (Table S4). Then, we conducted further analyses with significant terms at each level to obtain a brief graphic result, which led to the annotation of several major biological processes associated with autophagy, including defense responses, oxidative stress responses, phenylpropanoid metabolism and lignin metabolism, and an obvious autophagy-related subcellular localization in the mitochondria (A,B). In combination with a Kyoto Encyclopedia of Genes and Genomes (KEGG) analysis (Table S5A to C; Figure S2 and S3), 3 important aspects of the link between autophagy and Arabidopsis defense responses against V. dahliae infection can be highlighted and are outlined in the following sections.
Figure 5. Brief graphic result showing the GO annotation of proteins identified with the agriGO tool. (A) Biological process. (B) Cell component.
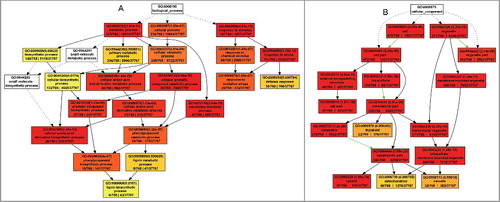
As to the 50 proteins identified between the WT and the mutant atg10-1 under mock inoculation conditions, we did not obtain significant GO terms by agriGO analysis. Thus, we searched the function of each protein from Tair database (http://www.arabidopsis.org). Functional categorization indicated that these proteins could be divided into 9 subclasses (Table S3F), including carbohydrate and energy metabolism, protein metabolism, signal transduction, stress response, transcription regulation and oxidation-reduction balance. These changes in protein abundance in the atg10-1 mutant indicated that autophagy defects already impacted the root proteome before infection.
Autophagy is involved in the activation of defense responses against V. dahliae infection
In our study, we identified a relatively large number of defense-related proteins, the majority of which belong to the subgroups for defense signaling, oxidation-reduction balance, lignin biosynthesis and PR proteins (Table S5A; ). The accumulation of 18 proteins (10 defense signaling, 3 oxidation-reduction balance-related, 1 PR, 1 lignin biosynthesis-related and 3 other proteins) failed to respond after the mutant was inoculated with the pathogen. These proteins included elicitor peptide precursor (AT5G09978/PEP7) and elicitor peptide receptor (AT1G73080/PEPR1); major latex-like proteins (AT4G23680, AT4G14060, AT3G26460/MLPs); calcium signaling-related proteins; and receptor and protein kinases. Thirteen out of 15 proteins (9 defense signaling-related, 3 oxidative stress-related, 2 PR and 1 lignin biosynthesis-related protein) were upregulated as DAPs upon pathogen infection from both the WT and atg10-1 mutant. Meanwhile, we also found that the induction of 4 defense signaling proteins, including AT5G47910/RBOHD, AT3G54640/TRP3, AT1G62820/CML14, AT2G35980/NHL10, were delayed in the mutant. For the proteins with absent or postponed V. dahliae responses due to defective autophagy, their functional cascade and subcellular localization are illustrated by a schematic representation (C). The picture clearly showed that the activation of defense-related processes was associated with autophagy. Interestingly, several proteins (AT5G47910/RBOHD, AT3G49110/PRXCA and AT1G73080/PEPR1) were reportedly localized in the extracellular apoplast, suggesting that autophagy may have a role in the apoplast, which is the first battlefield of plant defense against pathogen invasion, despite that autophagy is an intracellular degradation system. In addition, 10 defense-related proteins (one cell death-related, 5 defense signaling, one lignin synthesis, one PR protein, and 2 oxidative balance proteins) were identified only from the atg10-1 mutant. These proteins primarily included AT4G35310/CPK5, AT2G34690/ACD11, AT2G46370/JAR1, AT5G57220/CYP81F2, and AT4G32260/ PDE334. Seven of the 10 proteins were identified at 72 Hai, suggesting that autophagy defects led to other changes in the plant defense response to V. dahliae infection and the mutation caused changes after the peak appearance of autophagy activity. Thus, the data also indicated that autophagy is involved in the activation of plant immunity, and it is critical for normal defense against subsequent infection.
Figure 6. Autophagy is involved in the activation of defense responses against V. dahliae infection. (A) Classification of proteins that were annotated as defense response. (B) Heat map showing the accumulation change pattern of proteins that were annotated as defense response at different time points upon V. dahliae infection in comparison with mock inoculation. Blank space and letter M in this panel have the same meaning as that of B. (C) Schematic representation of the functional cascade and subcellular localization for the proteins whose V. dahliae responses were absent or postponed because of defective autophagy: ① When a pathogen infected a cell, an unknown PAMP activated its receptor, it triggered an ROS burst generated by RBOHD, and the burst signaling provoked HR cell death and cell wall reinforcement. ② The interaction of the elicitor peptide and its receptors activated calmodulin-like proteins and mediated NO generation that caused cell wall reinforcement. ③ MLP, Mediator 21 and RD19 mediated the transcriptional activation of the downstream defense response. ④ NHL10 mediated cell death. ⑤ WHY2 protein repaired DNA double-stranded breaks over an error-prone repair pathway in mitochondria. ⑥ thioredoxin, ATGSH1 and class III peroxidases mediated H2O2 homeostasis regulation in plant defense.
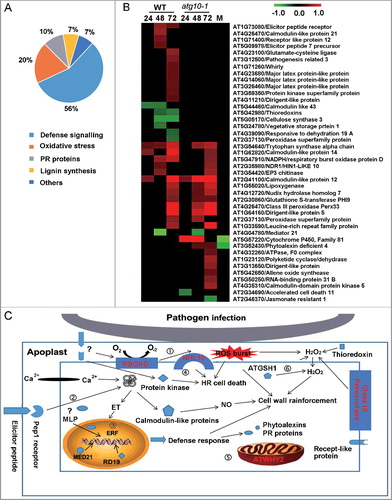
Mitophagy process occurred in defense against V. dahliae invasion
A number of the identified proteins are known to localize in mitochondria. These proteins and their accumulation profiles showed significant changes during V. dahliae invasion, and defective autophagy altered the patterns of such changes (Table S5B, A). We identified 15 mitochondria-localized proteins in the WT but not in the mutant (w+/m−), and 4 of them are components of a respiratory chain complex for proficient mitochondrial function or biogenesis (AT3G27280/PHB4, complex I; AT3G17910/SURF1, complex IV; AT1G15120/ubiquinol-cytochrome c reductase hinge protein, complex III; AT4G20150/an unknown protein, complex I). The accumulation profiles of these proteins indicated that mitochondrial respiration and turnover were associated with plant defense against V. dahliae invasion and were autophagy-dependent. The accumulation features of the other mitochondria-localized proteins identified as w+/m− and 5 proteins identified as w+/m+D provided evidence that protein synthesis and modification were repressed in the mitochondria during V. dahliae invasion. In the atg10-1 mutant, most of the changes were absent or postponed. Additionally, there were 26 mitochondria-localized proteins that were abnormally changed in atg10-1 (w−/m+) upon V. dahliae infection, of which, 11 proteins were associated with the removal of toxic ROS, including catalases, malate dehydrogenase, glutathione peroxidase and glutamate dehydrogenase, which were upregulated at 72 Hai. The enhancement of alternative respiration and impaired conversion of molecular oxygen to water in respiration complex IV were indicated by the accumulation change of isovaleryl-CoA dehydrogenase and cytochrome c oxidase. Together, these results indicate defective autophagy seriously affected the normal function of mitochondria upon V. dahliae infection.
Figure 7. Mitophagy-mediated plant defense against V. dahliae infection by removing dysfunctional mitochondria. (A) Heat map showing the annotated mitochondria-localized proteins. Blank space and letter M in this panel have the same meaning as that of B. (B) Colocalization of mitochondria and autophagosome-like structures in V. dahliae-infected transgenic Arabidopsis seedlings expressing both Mito-YFP and mCherry-ATG8E. The mature region of mock or inoculated primary root (upper 2 panels) or root hair (lower 2 panels) was imaged at 48 Hai by confocal fluorescence microscopy. Bar: 5 μm. (C) Quantification of colocalized puncta labeled by both Mito-YFP and mCherry-ATG8E. Mean and standard error (SE) were calculated from 15 mock or inoculated primary roots from transgenic seedlings, and similar results were obtained in 3 independent experiments which examined approximately 150 cells. “**” indicate statistically significant (P ≤ 0.01 vs mock), measured by the Student t test.
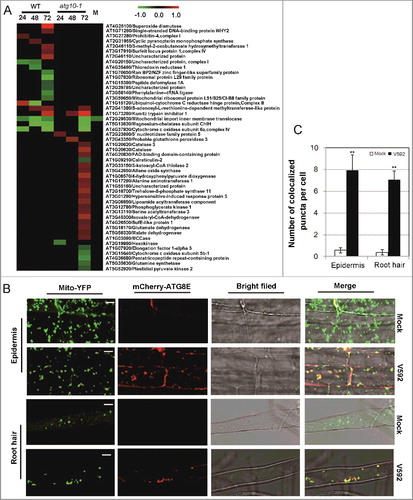
Since autophagy was associated with mitochondria turnover through participating in its degradation, the above data from proteomic analysis implied that mitophagy, which is one of the branch processes of autophagy, could have been activated for defense against V. dahliae invasion in Arabidopsis. To verify this event, we examined a transgenic line expressing both Mito-YFP and mCherry-ATG8E using fluorescence confocal microscopy in wild-type plants. Analysis of the root cells exposed to V. dahliae infection detected numerous puncta of mitophagosomal-like structures containing both fluorescent reporters, while such puncta were barely detected in the mock controls in which only Mito-YFP fluorescence was visualized (B,C). A transmission electron microscopy examination of infected roots confirmed the presence of mitophagosomal-like structures and showed its morphology clearly. As shown in A, mitophagy-indicative structures with double membranes were frequently observed around the degrading mitochondrial fragments in V. dahliae-infected wild-type root epidermis and cortex cells but not in the cells of the atg10-1 mutant, which contained accumulated and deformed mitochondria without presence of the mitophagy-indicative structures nearby. In the infected root perivascular cells of WT, intact mitochondria were found to be enclosed by double membranes (B). The quantitative data of mitophagy-indicative structures in the 2 kinds of cells from mock- and V592-inoculated roots at 48 Hai in WT and V. dahliae were shown in C.
Figure 8. TEM analysis of the mitophagy activation in Arabidopsis root cells challenged by V. dahliae. (A and B) Representative TEM images of the mock or inoculated root cells of WT and atg10-1 at 48 Hai. (A) Cortex cell. (B) Perivascular cell. a to d, enlarged images of the square in the middle panel. Arrows in “a” and “c” indicate mitophagosomal-like structures, arrow heads highlight the double membrane of the mitophagosomal-like structures. (C) Quantification of mitophagosome-like structures observed in TEM images of mock or V592 inoculated root cells in wild-type and atg10-1 plants. E/C, epidermis and cortex; P-V, perivascular. Bar = 0.5 µm for (A and B); bar: 0.2 µm for (a to d). WT, wild type. “**” indicate statistically significant (P ≤ 0.01 vs mock), measured by the Student t test, “##” indicate statistically significant (P ≤ 0.01 vs atg10-1), measured by the Student t test. Mean and standard error (SE) were calculated from 10 independent areas of 100 µm2.
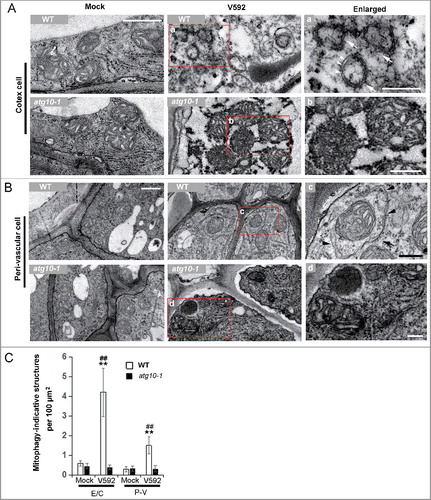
Autophagy is associated with the transdifferentiation and lignification of perivascular cells
The GO annotation for BP and KEGG analysis suggested that autophagy is related to phenylpropanoid metabolism and lignin biosynthesis (A, Fig. S3), and the accumulation profiles of the identified proteins were shown in more detail (Table S5C). Among them, accumulation change of proteins from GO: 0009699 named phenylpropanoid was found to be meaningful between WT and V. dahliae (A). In the WT, accumulation changes in the 5 lignin biosynthesis-related proteins, including the upregulation of lignin synthesis proteins (AT5G66690/UGT72E2, AT3G24503/ALDH1a and AT4G11210/ dirigent-like protein) and the downregulation of AT5G63590/flavonol synthesis-related proteins (2OG-Fe II) were detected after V. dahliae challenge; however, these proteins did not show responsive accumulation in the atg10-1 mutant. The responsive accumulation of another 5 proteins, including the upregulation of lignin biosynthesis-related proteins (AT1G33030/OMT, AT3G50740/UGT72E1, AT1G64160/DIR5) and the downregulation of AT5G08640/FLS1, were detected in both the WT and the mutant, although the changes were less obvious or delayed in the mutant. We also found that 10 proteins were abnormally changed upon V. dahliae infection in the atg10-1 mutant, and they consisted of 6 lignin- and 4 flavonoid-biosynthesis proteins, primarily AT1G76470/NAD(P)-binding Rossmann-fold superfamily protein, AT3G13650/dirigent-like protein, AT4G36220/F5H, AT4G16770/2-oxoglutarate (2OG)and Fe(II)-dependent oxygenase superfamily protein and AT5G13930/CHS. Most of the proteins involved in lignin synthesis were downregulated, including a critical enzyme for S-lignin synthesis (F5H), and 4 proteins involved in flavonoid biosynthesis were also downregulated. The accumulation features of these proteins indicated that the enhancement of lignin biosynthesis in response to V. dahliae infection was associated with autophagy.
Figure 9. Autophagy defects attenuate xylem cell lignification in response to V. dahliae infection in Arabidopsis. (A) Heat map showing the accumulation change of proteins annotated as phenylpropanoid metabolism process. Blank space and letter M have the same meaning as those in B. (B) Quantification of lignin content in WT and atg10-1 upon V. dahliae infection at 7, 14, 21 and 28 dpi. (C to E) Quantification of lignin intermediates in the WT and atg10-1 upon V. dahliae infection at 28 dpi. (F) Xylem hyperplasia was significantly attenuated in atg10-1 compared with those of the WT at 28 dpi, BF, bright field, AU, autofluorescence, Bar: 200 μm. (G and H) qRT-PCR quantification of the transcriptional level of VND 7 (G) and HB8 (H) corresponded to the enhancement of xylem formation. WT, wild type. “*” and “**” indicate statistically significant (P ≤ 0.05 or P ≤ 0.01 vs mock), “#” and “##” indicate statistically significant (P ≤ 0.05 or P ≤ 0.01 vs atg10-1) measured by the Student t test.
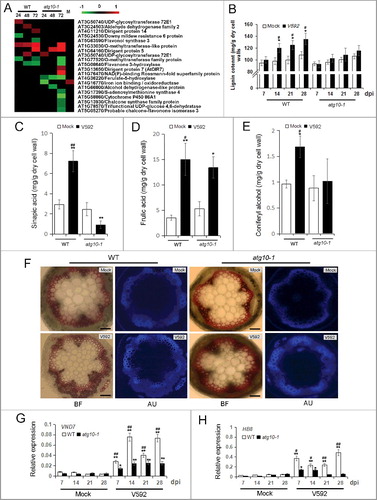
As stem is an ideal organ for observation of vascular structure and lignin deposition, we analyzed the contents of lignin and its intermediates in this organ. As shown in B, there was a clear increase of lignin content upon V. dahliae infection in WT. The time course measurement at 4 time-points (7/14/21/28 dpi) indicated that increase of lignin content was already started at 7 dpi, and continued afterwards. Compared to the WT, the lignin content in the atg10-1 mock was lower, and there was only a slight increase after infection. Further analysis indicated that the abundance of lignin intermediates at 28 dpi, when lignin content showed the highest increase among the 4 time-points, changed accordingly. As shown in C–E, the products of the phenylpropanoid pathway, such as sinapic acid (precursor of S-lignin), ferulic acid, and coniferryl alcohol, displayed different changes in their abundance upon V. dahliae infection in the WT and atg10-1. In the mock control of the 2 genotypes, these products were nearly equivalent, but upon pathogen infection, the amounts of sinapic acid increased in the WT and decreased in atg10-1 while the ferulic acid and coniferryl alcohol showed consistent changes but at different levels between the 2 genotypes.
We employed Phloroglucinol-HCl to stain the lignin on stem cross-sections to examine the changes in lignin deposition at the cellular level. In the wild-type plant, the vascular bundles developed into xylem hyperplasia via the transdifferentiation of adaxial bundle sheath cells; however, this structural change was repressed in the atg10-1 mutant (F). As a previous study indicated that the Verticillium-induced expression of the transcription factor gene AT1G71930/VND7 and the cambial marker gene AT4G32880/HB8 corresponded to the enhancement of xylem formation; [Citation33] thus, we determined the transcriptional expression of these 2 genes in the stems of the wild-type and atg10-1 plants that had been infected with V. dahliae. As shown in G,H, the transcription of both the VND7 and HB8 genes was induced by V. dahliae infection in the WT, but VND7 and HB8 genes was much less induced in the atg10-1 mutant.
Screening of potential proteins directly targeted/regulated by autophagy
The above analysis revealed the strong link between autophagy and defense responses against V. dahliae infection, suggesting that some identified proteins may be involved directly in the autophagy process. Looking for these proteins could provide insights for understanding the mechanisms of autophagy-mediated plant immunity. Since ATG8 proteins anchor in autophagosome membranes and interact with potential autophagy components/receptor/substrates containing the ATG8-interacting motif (AIM), also known as LC3-interacting region (LIR), we performed AIM motif screening to look for ATG8-family-interacting proteins. After protein amino acid sequence analysis by hfAIM [Citation34], iLIR [Citation35], and PONDR-FIT software [Citation36], 193 proteins, out of 780 identified proteins, were identified as putative ATG8-family-interacting proteins, including a few known ATG8-family-interacting proteins such as NBR1 and EXO70B1 (Table S6 and 7). A heatmap was then generated to analysis their abundance changes (A). It shows that the predicted ATG8-family-interacting proteins accumulated differently between WT and atg10-1 and distributed similarly in each of the 3 types of DAPs (w+/m−, w+/m−D and w−/m+). These data indicated that immune responses were directly linked with autophagy, and defective autophagy caused abnormal accumulation of many ATG8-family-interacting proteins under V. dahliae attack, which may disrupt the normal defense systems.
Figure 10. In silico prediction and experimental validation of representative ATG8-family-interacting proteins from the differentially accumulated proteins. (A) Heat map showing the accumulation changes of the predicted ATG8-family-interacting proteins upon V. dahliae infection, numbers of the predicted ATG8-family-interacting proteins and their proportion in total w+/m−, w+/m+D and w−/m+ proteins were shown in the colored rectangle; w+/m−, w−/m+ and w+/m+D indicate the same meaning mentioned in the text. Blank space and letter M have the same meaning as those in B. (B to H) Transient coexpression of GFP-ATG8A/8E (B, D to H, GFP-ATG8A; C, GFP-ATG8E) with mCherry-labeled ATG8-family-interacting proteins in N. benthamiana. (B) AT4G24690 (NBR1, Q9SB64). (C) AT5G58430 (EXO70B1, Q9FGH9). (D) AT3G06035 (Unknown protein, Q0WSE0). (E) AT1G21100 (IGMT1, Q9LPU5). (F) AT4G32360 (NADPH:AO, Q8W3L1). (G) AT5G13370 (GH3, Q8GZ29). (H) AT1G78870 (UBC13A, Q94A97). ATG8 proteins labeled by GFP (green); tested proteins labeled by mCherry (red); colocalization (yellow). Bar: 10 μm. (I) Quantification of numbers of fluorescence-labeled puncta for proteins colocalized with ATG8. “*” and “**” indicate statistically significant (P ≤ 0.05 or P ≤ 0.01 vs mock), measured by the Student t test.
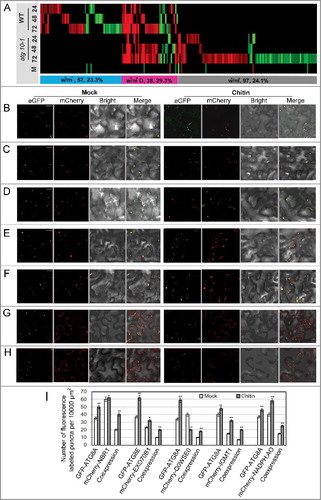
Next, agriGO Single Enrichment Analysis (SEA, Q value ≤ 0.01) was conducted for these putative proteins to investigate their contribution in defense against V. dahliae infection (Table S8, Figure S4). The GO enrichment results suggest that these proteins are involved in multiple biological processes, including phenylpropanoid biosynthetic process, response to fungus and sulfur metabolic process (GO: 0009699, 0009620 and 0006790), and their molecular functions are more related to oxidoreductase and methyltransferase activities (GO: 0016491 and 0008168). These results suggest that some proteins we identified may act as important players in autophagy-mediated immune responses against V. dahliae infection.
To verify the participation of the predicted ATG8-family-interacting proteins in autophagy-mediated plant immunity, we transiently coexpressed 7 mCherry-tagged proteins (Figure S5) including NBR1 and EXO70B1 with GFP-tagged ATG8A or ATG8E in Nicotiana benthamiana using the Agrobacterium tumefaciens GV3101 strain, then assessed their colocalization and response to chitin (a typical fugal pathogen-associated molecular pattern, PAMP) treatment. As a result, we found that 5 tested proteins with the AIM/LIR motif were colocalized with ATG8 puncta and the colocalization ratio was affected by chitin treatment (B–F), while the other 2 could not form puncta (G,H). This suggested that a large portion of putative ATG8-family-interacting proteins were indeed directly associated with autophagy.
Discussion
Proteomic study reveals the crucial roles of autophagy throughout the defense process against the semi-biotrophis fungus pathogen V. dahliae
Proteomics analysis is a powerful tool that can provide high-throughput information for the study of proteins involved in different biological processes [Citation32,Citation37] and this technique has been employed to investigate the function of autophagy, such as for suberoylanilide hydroxamic acid (SAHA)-triggered autophagy in Jurkat T-leukemia cells and the upregulation of basal autophagy in Ras-driven cancer cells [Citation38,Citation39]. To date, proteomics study in the field of autophagy-mediated plant immunity is rare. In this study, we conducted a comparative root proteomics analysis between a WT and an autophagy mutant in response to infection by the fungal pathogen V. dahliae in Arabidopsis. As previous studies indicated that autophagy-mediated plant immunity is pathogen-type dependent [Citation7] and the root is an ideal organ for studying interactions between vascular pathogens and plant hosts [Citation40], our work could provide insights for understanding the roles of autophagy in plant innate immunity to semi-biotrophic root-invading fungus pathogen. To minimize the proteome difference between WT and autophagy deficient Arabidopsis mutant under normal condition, we chose the atg10-1 mutant that grows similar as the WT at the 2-wk-old seedling stage. Indeed, only 50 DAPs were identified under mock-inoculation condition in comparison with the number of 780 identified after V. dahliae infection. The limited number of differential proteins should be due to the relatively healthy growing status of V. dahliae seedlings under normal condition. On the other hand, more than one-half of such proteins (36 out of 50) were responsive to V. dahliae infection in WT or the atg10-1 mutant. For example, NBR1 protein (a substrate and receptor of autophagy) was significantly upregulated upon infection. This result also reflected a link between autophagy and plant immune responses. Compared with the mock control, we obtained 15 folds more DAPs upon V. dahliae infection, and the proteins could be enriched in several biological processes or cell compartments. These data indicated a tight association of autophagy and plant immunity during V. dahliae infection. Moreover, we analyzed the autophagy-dependent defense-related proteins throughout the process of pathogen infection, propagation and colonization, and identified a number of important proteins that are involved in the early signaling transduction of PAMPs and damage-associated molecular patterns (DAMPs), which triggered plant immunity, as well as in signaling for hormone-mediated defenses and those participating in late defense processes.
Autophagy is required for upstream defense events against V. dahliae infection
In our study, a batch of V. dahliae-induced proteins that were identified in the WT was found to be either absent or induced at a slower speed in the atg10-1 mutant at the early stage of infection. Of these proteins, near half are known regulators of plant defense reactions. For example, the interaction of elicitor peptide and its receptor was found to contribute to plant immunity against pathogen attacks by inducing defense responses similar to pattern-triggered immunity [Citation41–43]. Here, we found that an elicitor peptide and an elicitor peptide receptor were upregulated in the WT, and this change was absent in the atg10-1 mutant, indicating that the inductions of both the elicitor peptide and its receptor were repressed in the mutant. Moreover, several important plant defense signaling proteins, including respiratory burst oxidase homolog protein D, RNA-binding protein CP31B (a substrate of the effector HopU1) and a tryptophan synthase alpha chain protein (auxin biosynthetic process), were also identified as V. dahliae-responsive proteins in the WT and showed postponed induction in the mutant. These results indicated that the autophagy process is required during the early events of plant immune responses.
Autophagy plays contradictory roles in restricting or generating HR in plant immune responses against different pathogens [Citation5,Citation9]. Here, we found that RBOHD, which is associated with the ROS burst and HR cell death upon fungal pathogen infection in the apoplast [Citation44,Citation45] was induced by V. dahliae infection at early stage, and the change was retarded in the atg10-1 mutant. ACD11, which is required for preventing cell death triggered by the R-protein LAZ5 [Citation46], was downaccumulated in the atg10-1 mutant. This suggested the possibility of inappropriate repression or activation of HR-related proteins in atg10-1 mutant. Previously, vascular HR was observed during the interaction between Xanthomonas and Arabidopsis [Citation20]. Our data also supported the idea that vascular HR could have occurred during the defense against V. dahliae infection in Arabidopsis and that autophagy was involved.
Mitophagy occurs and contributes to defenses against V. dahliae infection
Mitochondria are crucial organelles in energy conversion, metabolism and signaling amplification. The by-products from complexes I and III of the mitochondrial electron transport chain (mETC) during metabolic processes are the primary sources of cellular ROS, which have a double-edged effect: [Citation47] mitochondrial-generated ROS can serve as a signal to regulate cellular processes, including responses to biotic stress, whereas excess ROS could damage mitochondria by oxidizing the mitochondrial lipids, proteins and DNA [Citation48-52]. As a form of selective autophagy, mitophagy can eliminate superfluous or ROS-damaged mitochondria [Citation48,Citation53]. In this study, we found that 15 proteins localized in mitochondria (4 in respiratory complex) failed to respond V. dahliae infection due to defective autophagy. This included the protein PHB4, its homologue PHB2 was very recently revealed to be a crucial mitophagy receptor required for targeting mitochondria for autophagic degradation in C. elegans [Citation54]. Consistent with the protein abundance changes, we found that mitophagy indeed mediates plant defense by affecting the mitochondrial degradation upon V. dahliae infection.
Mitophagy is very important for maintenance of normal mitochondrial function for the overall cell fitness [Citation47]. Here, we observed mitophagy-indicative structures with different morphologies in the infected root cells of the WT, with some exhibiting fission to small bodies and others appearing intact and parceled by the 2-layer autophagosome membranes (). We also found that the former mitophagy-indicative structures usually occur in epidermis and cortex cells undergoing programmed cell death (PCD) according to the PCD morphology described in previous studies [Citation55], and the latter occurs in the perivascular cells. In the atg10-1 mutant, defective autophagy resulted in swelling or aberrant mitochondrial accumulation in root cells without typical mitophagosomal-like structures. The defect associated with removing aberrant mitochondria could cause oxidative toxicity and subsequently affect the normal function of mitochondria and increase the disease susceptibility of the mutant. To the best of our knowledge, this is the first report indicating that the presence of mitophagy is related to the disease tolerance of an infected plant.
Autophagy plays a novel role in structural defense against vascular disease
Lignins are formed by the oxidative coupling of 3 p-hydroxycinnamyl alcohols (monolignols), p-coumaryl, coniferyl, and sinapyl, to form 3-dimensional, amorphous and heteropolymer structures. This reaction is catalyzed by peroxidases and depends on H2O2 [Citation56]. During plant defense against soil-borne pathogens, cell wall lignification is particularly important. Several studies have reported that the phenylpropanoid pathway and peroxidase and phenylalanine-ammonia lyase (PAL) activity were essential for plant structural defense against V. dahliae infection in cotton [Citation57,Citation58]. In Arabidopsis, xylem hyperplasia is triggered by V. longisporum infection [Citation33]. During this process, the reinitiation of cambial activity and the transdifferentiation of xylem parenchyma generate xylem hyperplasia within the vasculature of Arabidopsis leaves, hypocotyls, and roots, and it partially restores the water transport and storage capacity of vessels clogged by pathogens, thus triggering a tissue-specific plant developmental program to enhance plant tolerance to the pathogen. Importantly, we found that these changes also occurred after V. dahliae infection, and according to a comparative proteomics study between the WT and the atg10-1 mutant in our study, we observed that the changes were related to autophagy. In a parallel experiment with V. dahliae-infected stems, we also observed the associations of xylem hyperplasia, the increase of xylem content and synthesis of S-lignin with autophagy. Additional analyses indicated that autophagy mediated the Verticillium-induced enhancement of xylem formation, which is dependent on upstream signaling components that regulate the reinitiation of cambial activity and the transdifferentiation of xylem parenchyma. As Kwon et al. report, cell death signals may activate autophagy via the activation of RABG3B and the expression of ATGs in tracheary element (TE)-differentiating cells to form xylem cells [Citation59]. We speculate that possible vascular HR cell death signaling that appears tightly correlated with autophagy caused by V. dahliae infection may positively regulate the transdifferentiation of the perivascular cells that contribute to structural defense. This finding reveals an important new role of autophagy in mediating plant immunity against vascular pathogens.
The prediction of ATG8-family-interacting proteins provides novel insight for understanding autophagy-mediated plant immunity
ATG8-family-interacting proteins help to target cargo receptors to autophagosomes as well as to act in autophagosome formation, transport and maturation. Since their important roles in autophagy process, several studies focused on the studies of reliable identification of ATG8-family-interacting proteins, and a few bioinformatics tools were developed accordingly [Citation34-36]. As an important part of our study, we used these developed tools or methods for screening ATG8-family-interacting proteins from the DAPs between WT and atg10-1 under V. dahliae infection. Since these tools or approaches have respective advantages, we used each of them to identify the putative AIM/LIR motifs, including calculation of their Position Specific Scoring Matrices (PSSM) value and determination of their mapped region by PONDR-FIT. Subsequent verification about the direct involvement of these proteins in autophagy-mediated immunity on several selected proteins demonstrated the reliability of our prediction data.
In silico identification of LIR-motif-containing proteins based on proteomic data is suggested by Xie et al. in a recently published study [Citation34]. In our study, we found various autophagy-mediated immunity responses as well as the putative proteins directly involved in autophagy-related immune responses. For instance, AT1G78570/ RHM1, At1g21100/IGMT1, AT1G77520/OMT, AT4G23100/PAD2 and AT4G04780/MED21, were identified as ATG8-family-binding proteins involved in phenylpropanoid metabolism and defense response, respectively. Previous studies indicated these proteins were important players in plant immunity, prediction of their link with autophagy can further recognize their functions.
Summary
Based on the comparative iTRAQ protein profile and GO analyses, we characterized several functional associations between autophagy and defense responses against V. dahliae infections in Arabidopsis. Our data implied that autophagy is involved throughout the entire defense process, from the early PAMP/DAMP-triggered defense signaling in apoplasts to the late response of phenylpropanoid and lignin biosynthesis in the cell wall. Furthermore, we observed the formation of mitophagosomes during the defense process, indicating that mitophagy is also an important defense process in plant cells, as it is in animal cells. In addition, we found that autophagy is tightly associated with xylem hyperplasia, which may represent an important strategy in plant defense against the vascular pathogens. Importantly, we identified a number of ATG8-family-interacting proteins that may include important players in autophagy-related defense, therefore deserving further studies.
Materials and methods
Plant material and growth conditions
Columbia (Col-0), autophagy mutants atg5 (SALK_020601), atg7 (SAIL_11_H07), and atg10-1 (SALK_084434), and Mito-YFP (CS16264) [Citation60] were from the Arabidopsis Biological Resource Center at Ohio State University. The GFP-ATG8E transgenic line [Citation11] was kindly provided by Prof. Ding-Zhong Tang (Institute of Genetics and Developmental Biology, Chinese Academy of Science). The atg10-1 mutant expressing GFP-ATG8E was generated by crossing atg10-1 mutant and GFP-ATG8E transgenic Arabidopsis plants. Arabidopsis plants expressing both Mito-YFP and mCherry-ATG8E reporters were generated by transforming mCherry-ATG8E construct to a stable transgenic line of Mito-YFP. The seeds of Arabidopsis plants were germinated on agar plates and vernalized at 4°C for 3 d. The seedlings or plants were grown in an illuminated growth chamber at 22°C under 16 h/8 h light/dark conditions.
V. dahliae inoculum preparation and inoculation
The V. dahliae isolate V592 was used as the inoculum. For conidial production, the fungus was grown on Czapek medium at 26°C for 4 days. A conidial suspension (of 1 × 106 conidia/mL) in distilled and deionized water was prepared for inoculation. For the disease tolerance assessment, the lignin analysis and the stem cross-section staining, one-wk-old seedlings were removed and the roots were then dip-inoculated in either the V. dahliae conidial suspension or mock solution for 5 min and cultivated in the vermiculite before sample collection. For the iTRAQ experiment, 2 mL of conidial suspension or mock solution was inoculated onto the roots of 2-wk-old plants on vertical plates for 15 min and then cultivated until sampling. For the confocal microscopy and TEM analysis, 5-d-old wild-type seedlings were dip-inoculated with V. dahliae conidia for 5 min and then placed onto vertical plates before sampling.
Leaf surface measurement
Healthy leaves were cut and pasted on paper together with a black square object region as a reference. Photographs were taken with a digital camera and saved in JPEG format, and the leaf area was measured by ImageJ software. The principle of the measurement has been clearly described by Chaudhary et al [Citation61].
Protein extraction, digestion, and iTRAQ-8plex labeling LC-MS/MS
Root proteins were extracted and quantified according to a previous method with minor modifications [Citation62]. Briefly, fresh roots were powdered in liquid nitrogen and homogenized in extraction buffer (50 mM phosphate-buffered saline, 100 mM NaCl, 1 mM PMSF, and 1 mM EDTA). After methanol-chloroform precipitation, 100 μg of the proteins was dissolved in TEAB buffer (Sigma-Aldrich, 17902). The samples were then reduced, alkylated, trypsin-digested, and labeled following the manufacturer's instructions for the iTRAQ Reagents 8-plex kit (AB Sciex, Framingham, MA, 4390812). For each biological replicate, 2 mock-inoculated samples from the WT and atg10-1 (pooled from equal amounts of samples at 24, 48, and 72 Hai) were labeled with iTRAQ tags 113 and 117, and the V. dahliae-inoculated lines (WT from 24, 48, and 72 Hai, and atg10-1 at 24, 48, and 72 Hai) were labeled with tags 114, 115, 116, 118, 119, and 121, respectively (Figure S1). After the 8 labeled samples were combined, the iTRAQ-labeled peptides were fractionated by strong-cation exchange (SCX) chromatography on an Ekspert TM ultraLC 100 system (AB Sciex). Peptide samples were resuspended in buffer A (20 mM ammonium formate at pH 10) and eluted with a 5% to 90% linear gradient of buffer B (20 mM ammonium formate, 80% acetonitrile, pH 10) over 60 min with a flow rate of 1 mL/min. Twenty fractions were collected according to the peak area and concentrated by desalting and lyophilization. The fractions were resuspended in 20 μL of formic acid (0.1%) and centrifuged. The samples were separated on a Thermo Scientific™ EASY-Spray™ PepMap™ C18 column with a Thermo Scientific™ EASY-nLC™ 1000 HPLC system (San Jose, CA) and analyzed using a high-resolution Orbitrap Fusion mass spectrometer (Thermo Fisher Scientific, Waltham, MA).
Database search and quantitative proteome analysis
Tandem mass spectra were extracted, charge state-deconvoluted and deisotoped by MASCOT Distiller version 2.5.1. All MS/MS samples were analyzed using MASCOT, which was set up to search the Arabidopsis TAIR 10 database assuming that the digestion enzyme was trypsin. The MASCOT program was used to search with a fragment ion mass tolerance of 0.020 Da and a parent ion tolerance of 10 PPM. The methylthio of cysteine, the iTRAQ-8-plex of lysine and the N terminus were specified in MASCOT as fixed modifications. The oxidation of methionine and the iTRAQ-8-plex of tyrosine were specified in MASCOT as variable modifications.
Scaffold (Scaffold 4.4.5) was used to validate and quantify the MS/MS-based peptide and protein identifications. According to the Scaffold Local FDR algorithm, peptide identifications were accepted if they could be established at an FDR of less than 0.1%. For protein identification, their acceptance was based on an FDR of less than 5% and with at least 2 unique peptides. Protein probabilities were assigned by the Protein Prophet algorithm [Citation63]. Proteins that contained similar peptides and could not be differentiated based on the MS/MS analysis alone were grouped to satisfy the principles of parsimony. Proteins sharing significant peptide evidence were grouped into clusters. Channels were corrected according to the algorithm described by Shadforth et al [Citation64]. The acquired intensities in the experiment were globally normalized across all acquisition runs. Individual quantitative samples were normalized within each acquisition run. The intensities for identification of each peptide were normalized within the assigned protein. The reference channels were normalized to produce a 1:1-fold change. All the normalization calculations were performed using medians to normalize the data in a multiplicative fashion. DAPs with a Log2-fold change ≥0.38 or ≤−0.38 compared with the control were selected, and Mann-Whitney Test results with P ≤ 0.05 were chosen as significantly changed proteins.
GO annotation and bioinformatic analysis
For the functional classification, the identified protein were analyzed according to GO terms using the SEA tool (http://bioinfo.cau.edu.cn/agriGO/) [Citation65] for BP, CC and MF classifications based on a background database list of Arabidopsis TAIR 9. Selected significant terms were used to generate graphical results to simplify and outline the data. The KEGG encyclopedia was used for the identification of pathways (http://www.genome.jp/kegg) [Citation66]. The identified proteins that were excluded from the agriGO and KEGG analyses were searched in the TAIR database, and their GO information was then obtained.
Western blot analysis
Twenty-five micrograms of proteins from Arabidopsis roots were resolved in 8% or 12% SDS-PAGE gels and transferred to PVDF membranes (PALL life sciences, 66543). The filter was blocked overnight with 5% w/v milk powder in 20 mM Tris-HCl, pH 7.5, 0.15 M NaCl, and then incubated with the polyclonal primary antibodies from Agrisera (NBR1, 1:2000, AS142805; ADH, 1:1000, AS10685) or Abcam (SUMO1, 1:1000, ab5316) or actin depolymerizing factor (ADF, 1:1000) [Citation67] for 2 h at room temperature. After 3 washes, the membranes were incubated for 1 h with anti-rabbit IgG HRP-conjugated antibody (1:2500; Abcam, ab6721), and the protein signals were detected using a chemiluminescent kit (Bio-Rad, 1705060).
Quantitative real-time PCR (qRT-PCR) analysis
The qRT-PCR assay was conducted using SYBR Green Real-Time PCR Master Mix (Toyobo, QPK-201) and the DNA Engine Opticon 2 Real-Time PCR Detection System (MJ Research, Waltham, MA). To measure the transcript levels of selected genes in the roots, total RNA was extracted using TRIzol reagent (Invitrogen, 15596026) according to the manufacturer's protocol. Two mock-inoculated samples from the WT and atg10-1 (pooled with equal amounts of samples at 24, 48 and 72 Hai) were used as mock control. The AT1G50010/TUA2 gene was amplified for normalization. A fungal biomass quantification was performed according to a previous method [Citation68] with minor modifications to allow for quantification by obtaining data relative to the control. Primers used are illustrated in Table S1.
Confocal and electron microscopy
A scanning confocal microscope (Leica TCS SP8, Heidelberg, Germany) was used for the visualization of fluorescence in the plants. The cell images of GFP-ATG8E [Citation11], GFP-ATG8A and Mito YFP [Citation69] were obtained with 488-nm light combined with the emission from 500 to 540 nm. The cell images of mCherry-labeled proteins were obtained with 561 nm light combined with the emission from 580 to 650 nm.
For transmission electron microscopy (TEM) to visualize autophagy-related structures [Citation70], the maturation zone of Arabidopsis primary roots, which was 0.5 cm away from the root tip, was cut into 1-cm sections and fixed in 2.0% paraformaldehyde and 2.5% glutaraldehyde in 0.1 M phosphate buffer (pH 7.0) overnight at 4°C. After washing in buffer, the sections were postfixed for 2 h at room temperature in buffered 1% OsO4, washed and dehydrated using a series of ethanol and acetone, and then embedded in Spurr resin using a SPI-Chem low viscosity kit (SPI, 02690-AB). Thin sections of 60 to 80 nm were cut by an ultramicrotome (Leica EM UC7, Wetzlar, Germany), stained with uranyl acetate and lead citrate, then examined and photographed under a JEM-1400 transmission electron microscope (JEOL, Japan). The quantitation of mitophagy-like structures in TEM sections was performed following the method for quantitation of autolysosome and autophagosome structures described by Liu et al [Citation5].
Phloroglucinol-HCl staining
Stem sections were obtained by hand-cutting from the base of the inflorescence stems, then were stained with 2% phloroglucinol (Sigma, P3502) in 95% ethanol and concentrated HCl (v:v, 2:1) for 5 min. All samples were observed under a Zeiss Axio observer Z1 inverted fluorescence microscope (ZEISS, Oberkochen, Germany) and photographed using an Axiocam IcC5 digital camera (ZEISS, Oberkochen, Germany). The images were obtained under bright field and a fluorescence filter (Filter set 49 DAPI shift free (E) EX G 365, BS FT 395, EM BP 445/50) for detection of autofluorescence. Photos of autofluorescence were taken with an exposure time of 1.5 sec.
Cell wall preparation and quantification of lignin
The cell wall preparation and lignin quantification were performed via the acetyl bromide method as described previously [Citation71]. To quantify the lignin content by the acetyl bromide method, each cell wall sample (20 mg) was mixed with 0.5 ml of 25% acetyl bromide (v:v in glacial acetic acid) and incubated at 70°C for 30 min, quickly cooled in an ice bath, and then mixed with 0.9 ml of 2 M NaOH, 0.1 mL of 5 M hydroxylamine-HCl, and 4.5 mL of glacial acetic acid for the complete solubilization of the lignin extract. After centrifugation (14,006 g, 5 min), the absorbance of the supernatant was measured at 280 nm. A standard curve was generated with alkali lignin, and the obtained lignin content was expressed as mg lignin/g dry cell wall.
LC-MS analysis of lignin compositions by thioglycolate lignin phenolics
Cell wall powder from Arabidopsis stems (200 mg) was used for lignin extraction via the thioglycolate method to obtain thioglycolate lignin phenolics as described by Han et al [Citation72]. An Agilent 1260 HPLC coupled with an AB SCIEX QTRAP 4500 system (AB SCIEX, Foster, CA, USA) was used for the LC-MS analysis. The compounds were separated on an Agilent ZORBAX SB C18 column (5 μm, 4.6 × 250 mm) under a 25°C column temperature. The elution solvent system consisted of ultra-pure water (solvent A) and acetonitrile (solvent B). The injection volume of the autosampler was 5 μL. The gradient elution program was applied at a flow rate of 0.4 mL/min as follows: 0 min, 5% B; 5 min, 10% B; 20 min, 12% B; 30 min, 25% B; 35 min, 35% B; 40 min, 40% B; 55 min, 50% B; 65 min, 100% B; 75 min, 100%; 78 min, 5% B; and 85 min, 5% B.
The mass spectrometry was performed with electrospray ionization (ESI). MS analysis was conducted in negative-ion mode. The operating parameters were optimized as follows: collision gas (CUR): 20.0; collision gas (CAD): medium; IonSpray voltage (IS): −4500 V; temperature: 550°C; ion source gas 1 (GS1): 60.0; ion source gas 2 (GS2): 60.0; declustering potential (DP): −80.0; entrance potential (EP): −10; collision energy (CE): −20; and collision cell exit potential (CXE): −18.0. Ion detection was performed in multiple reaction monitoring (MRM) mode. The scanning time for the ion pair was 150 ms. The monitored MRM transitions were 222.9→148.8 for sinapic acid, 192.8→133.9 for ferulic acid and 178.8→134.8 for coniferyl alcohol).
Identification of ATG8-family-interacting proteins with an AIM/LIR motif
Amino acid sequences of proteins were downloaded from the UniProt database (http://www.uniprot.org/uni-prot). ATG8-family-interacting proteins were predicted by the following procedure: 1) prediction of proteins with LIR/AIM motif by hfAIM and (http://bioinformatics.psb.ugent.be/hfAIM/); [Citation34] 2) selection of proteins with LIR/AIM motif by confining Position Specific Scoring Matrices (PSSM) value (≥10) calculated using iLIR online program (http://repeat.biol.ucy.ac.cy/iLIR/); [Citation35] 3) further selection of proteins with LIR/AIM motif mapped onto an intrinsically disordered protein region (IDPR), which shows a characteristic PONDR-FIT profile consisting of 30 or more amino acid residues with high scores [Citation36] (near or above 0.5, http://www.disprot.org/pondr-fit.php).
Transient expression assay in N. benthamiana
The vector pCAMBIA1300-35S-mCherry-mcs-Nos was used for expression of mCherry tagged proteins [Citation73]. The vector pCAMBIA1300-35S-GFP-mcs-Nos was constructed by replacing mCherry with GFP, to express GFP-ATG8 proteins. The coding sequences of putative ATG8-family-interacting proteins or ATG8-family genes were PCR amplified and introduced into vectors between Spe I and Xho I sites. For the transient coexpression assay, each pair of A. tumefaciens GV3101 strains containing 2 different constructs were mixed in 1:1 ratio in agroinfiltration medium (10 mM MgCl2, 5 mM 2-[N-morpholine]-ethanesulfonic acid [MES], pH 5.6) to a final OD600 of 0.2, and injected into 3 to 4-week-old N. benthamiana plants as described previously [Citation74]. The bacterial solutions were divided into 2 equal parts for mock or chitin treatment (5 μM; Sigma-Aldrich, C9752).
Statistical analyses
For quantitative proteome analysis, Scaffold 4.4.5 software was used to calculate the relative protein levels and the P values (Mann-Whitney Test). For statistical significance analyses of other experiments, Microsoft Excel (Office 2010 edition) was used to calculate the mean and the standard deviation, and the P value (≤ 0.05 or 0.01) was determined by Student t test. All data are representatives of three independent experiments.
Disclosure of potential conflicts of interest
There were no potential conflicts of interest to be disclosed.
supp_data_1423438.zip
Download Zip (7.9 MB)Acknowledgments
We thank Prof. Ding-Zhong Tang (Institute of Genetics and Developmental Biology, Chinese Academy of Sciences) for providing atg-5, atg-7, atg-10-1 and GFP-ATG8E seeds, Prof. Richard-D Vierstra (Department of Genetics, University of Wisconsin-Madison) for Mito-YFP seeds. We are grateful to Prof. Rong-Xiang Fang for providing pCAMBIA1300-35S-mCherry-mcs-Nos vector, Lei Su (Institute of Microbiology, Chinese Academy of Sciences) for technical assistance with confocal microscopy analysis, Dr. Jie Zhang (Institute of Microbiology, Chinese Academy of Sciences) for critical reading of the manuscript and helpful discussion.
Additional information
Funding
References
- Klionsky DJ, Emr SD. Autophagy as a regulated pathway of cellular degradation. Science. 2000;290:1717–1721. doi:10.1126/science.290.5497.1717. PMID:11099404
- Thompson AR, Vierstra RD. Autophagic recycling: lessons from yeast help define the process in plants. Curr Opin Plant Biol. 2005;8:165–173. doi:10.1016/j.pbi.2005.01.013. PMID:15752997
- Han S, Yu B, Wang Y, et al. Role of plant autophagy in stress response. Protein Cell. 2011;2:784–791. doi:10.1007/s13238-011-1104-4. PMID:22058033
- Liu Y, Bassham DC. Autophagy: pathways for self-eating in plant cells. Annu Rev Plant Biol. 2012;63:215–237. doi:10.1146/annurev-arplant-042811-105441. PMID:22242963
- Liu Y, Schiff M, Czymmek K, et al. Autophagy regulates programmed cell death during the plant innate immune response. Cell. 2005;121:567–577. doi:10.1016/j.cell.2005.03.007. PMID:15907470
- Andrew PH, Dinesh-Kumar SP. What can plant autophagy do for an innate immune response? Annu Rev Phytopathol. 2011;49:557–576. doi:10.1146/annurev-phyto-072910-095333. PMID:21370973
- Lenz HD, Haller E, Melzer E, et al. Autophagy controls plant basal immunity in a pathogenic lifestyle-dependent manner. Autophagy. 2011;7:773–774. doi:10.4161/auto.7.7.15535. PMID:21460628
- Zhou J, Yu J-Q, Chen Z. The perplexing role of autophagy in plant innate immune responses. Mol Plant Pathol. 2014;15:637–645. doi:10.1111/mpp.12118. PMID:24405524
- Hofius D, Schultz-Larsen T, Joensen J, et al. Autophagic components contribute to hypersensitive cell death in Arabidopsis. Cell. 2009;137:773–783. doi:10.1016/j.cell.2009.02.036. PMID:19450522
- Wang Y, Wu Y, Tang D. The autophagy gene, ATG18a, plays a negative role in powdery milde resistance and mildew-induced cell death in Arabidopsis. Plant Signal Behav. 2011;6:1408–1410. doi:10.4161/psb.6.9.16967. PMID:21847024
- Wang Y, Nishimura MT, Zhao T, et al. ATG2, an autophagy-related protein, negatively affects powdery mildew resistance and mildew-induced cell death in Arabidopsis. Plant J. 2011;68:74–87. doi:10.1111/j.1365-313X.2011.04669.x. PMID:21645148
- Yoshimoto K. Plant autophagy puts the brakes on cell death by controlling salicylic acid signaling. Autophagy. 2010;6:192–193. doi:10.4161/auto.6.1.10843. PMID:20023431
- Yoshimoto K, Jikumaru Y, Kamiya Y, et al. Autophagy negatively regulates cell death by controlling NPR-dependent salicylic acid signaling during senescence and the innate immune response in Arabidopsis. Plant Cell. 2009;21:2914–2927. doi:10.1105/tpc.109.068635. PMID:19773385
- Patel S, Dinesh-Kumar SP. Arabidopsis ATG6 is required to limit the pathogen-associated cell death response. Autophagy. 2008;4:20–27. doi:10.4161/auto.5056. PMID:17932459
- Lai Z, Wang F, Zheng Z, et al. A critical role of autophagy in plant resistance to necrotrophic fungal pathogens. Plant J. 2011;66:953–968. doi:10.1111/j.1365-313X.2011.04553.x. PMID:21395886
- Lenz HD, Haller E, Melzer E, et al Autophagy differentially controls plant basal immunity to biotrophic and necrotrophic pathogens. Plant J. 2011;66:818–830. doi:10.1111/j.1365-313X.2011.04546.x. PMID:21332848
- Han S, Wang Y, Zheng X, et al. Cytoplastic glyceraldehyde-3-phosphate dehydrogenases interact with ATG3 to negatively regulate Autophagy and immunity in Nicotiana benthamiana. Plant Cell. 2015;27:1316–1331. doi:10.1105/tpc.114.134692. PMID:25829441
- Zimmermann AC, Zarei M, Eiselein S, et al. Quantitative proteomics for the analysis of spatio-temporal protein dynamics during autophagy. Autophagy. 2010;6:1009–1016. doi:10.4161/auto.6.8.12786. PMID:20603599
- Yadeta K, Thomma B. The xylem as battleground for plant hosts and vascular wilt pathogens. Front Plant Sci. 2013;4:97. doi:10.3389/fpls.2013.00097. PMID:23630534
- Xu RQ, Blanvillain S, Feng JX, et al. AvrAC(Xcc8004), a type III Effector with a leucine-rich repeat domain from Xanthomonas campestris pathovar campestris confers avirulence in vascular tissues of Arabidopsis thaliana ecotype Col-0. J Bacteriol. 2008;190:343–355. doi:10.1128/JB.00978-07. PMID:17951377
- Kawchuk LM, Hachey J, Lynch DR, et al. Tomato Ve disease resistance genes encode cell surface-like receptors. Proc Natl Acad Sci USA. 2001;98:6511–6515. doi:10.1073/pnas.091114198. PMID:11331751
- Zhang Z, Fradin E, de Jonge R, et al. Optimized agroinfiltration and virus-induced gene silencing to study Ve1-mediated Verticillium resistance in tobacco. Mol Plant Microbe Interact. 2013;26:182–190. doi:10.1094/MPMI-06-12-0161-R. PMID:22991998
- Klosterman SJ, Atallah ZK, Vallad GE, et al. Diversity, pathogenicity, and management of Verticillium species. Annu Rev Phytopathol. 2009;47:39–62. doi:10.1146/annurev-phyto-080508-081748. PMID:19385730
- Pegg G, Brady B. Verticillium wilts. Wallingford (Oxfordshire): CABI Publishing; 2002.
- Schnathorst WC. Life cycle and epidemiology of Verticillium. In: Mace ME, Bell AA, Beckman CH, editors. Fungal wilt diseases of plants. New York (NY): Academic Press; 1981. p. 81–111.
- Fradin EF, Thomma BPHJ. Physiology and molecular aspects of Verticillium wilt diseases caused by V. dahliae and V. albo-atrum. Mol Plant Pathol. 2006;7:71–86. doi:10.1111/j.1364-3703.2006.00323.x. PMID:20507429
- Phillips AR, Suttangkakul A, Vierstra RD. The ATG12-conjugating enzyme ATG10 is essential for autophagic vesicle formation in Arabidopsis thaliana. Genetics. 2008;178:1339–1353. doi:10.1534/genetics.107.086199. PMID:18245858
- Yoshimoto K, Hanaoka H, Sato S, et al. Processing of ATG8s, ubiquitin-like proteins, and their deconjugation by ATG4s are essential for plant autophagy. Plant Cell. 2004;16:2967–2983. doi:10.1105/tpc.104.025395. PMID:15494556
- Contento AL, Xiong Y, Bassham DC. Visualization of autophagy in Arabidopsis using the fluorescent dye monodansylcadaverine and a GFP-AtATG8e fusion protein. Plant J. 2005;42:598–608. doi:10.1111/j.1365-313X.2005.02396.x. PMID:15860017
- Walczak M, Martens S. Dissecting the role of the Atg12-Atg5-Atg16 complex during autophagosome formation. Autophagy. 2013;9:424–425. doi:10.4161/auto.22931. PMID:23321721
- Wang FX, Ma YP, Yang CL, et al. Proteomic analysis of the sea-island cotton roots infected by wilt pathogen Verticillium dahliae. Proteomics. 2011;11:4296–4309. doi:10.1002/pmic.201100062. PMID:21928292
- Parker J, Koh J, Yoo MJ, et al. Quantitative proteomics of tomato defense against Pseudomonas syringae infection. Proteomics. 2013;13:1934–1946. doi:10.1002/pmic.201200402. PMID:23533086
- Reusche M, Thole K, Janz D, et al. Verticillium infection triggers VASCULAR-RELATED NAC DOMAIN7-dependent de novo xylem formation and enhances drought tolerance in Arabidopsis. Plant Cell. 2012;24:3823–3837. doi:10.1105/tpc.112.103374. PMID:23023171
- Xie Q, Tzfadia O, Levy M, et al. hfAIM: A reliable bioinformatics approach for in silico genome-wide identification of autophagy-associated Atg8-interacting motifs in various organisms. Autophagy. 2016;12:876–887. doi:10.1080/15548627.2016.1147668. PMID:27071037
- Kalvari I, Tsompanis S, Mulakkal NC, et al. iLIR: A web resource for prediction of Atg8-family interacting proteins. Autophagy. 2014;10:913–925. doi:10.4161/auto.28260. PMID:24589857
- Popelka H, Klionsky DJ. Analysis of the native conformation of the LIR/AIM motif in the Atg8/LC3/GABARAP-binding proteins. Autophagy. 2015;11:2153–2159. doi:10.1080/15548627.2015.1111503. PMID:26565669
- Wu S, Xu Y, Feng Z, et al. Multiple-platform data integration method with application to combined analysis of microarray and proteomic data. BMC Bioinformatics. 2012;13:320. doi:10.1186/1471-2105-13-320. PMID:23198695
- Mathew R, Khor S, Hackett SR, et al. Functional role of autophagy-mediated proteome remodeling in cell survival signaling and innate immunity. Mol Cell. 2014;55:916–930. doi:10.1016/j.molcel.2014.07.019. PMID:25175026
- Li J, Liu R, Lei Y, et al. Proteomic analysis revealed association of aberrant ROS signaling with suberoylanilide hydroxamic acid-induced autophagy in Jurkat T-leukemia cells. Autophagy. 2015;6:711–724. doi:10.4161/auto.6.6.12397. PMID:20543569
- Mehta A, Magalhães BS, Souza DS, et al. Rooteomics: the challenge of discovering plant defense-related proteins in roots. Curr Protein Pept Sci. 2008;9:108–116. doi:10.2174/138920308783955225. PMID:18393883
- Koers S, Guzel-Deger A, Marten I, et al. Barley mildew and its elicitor chitosan promote closed stomata by stimulating guard-cell S-type anion channels. Plant J. 2010;68:670–680. doi:10.1111/j.1365-313X.2011.04719.x. PMID:21781196
- Bartels S, Lori M, Mbengue M, et al. The family of Peps and their precursors in Arabidopsis: differential expression and localization but similar induction of pattern-triggered immune responses. J Exp Bot. 2013;64:5309–5321. doi:10.1093/jxb/ert330. PMID:24151300
- Bartels S, Boller T. Quo vadis, Pep? Plant elicitor peptides at the crossroads of immunity, stress, and development. J Exp Bot. 2015;66:5183–5193. doi:10.1093/jxb/erv180. PMID:25911744
- Kadota Y, Sklenar J, Derbyshire P, et al. Direct regulation of the NADPH oxidase RBOHD by the PRR-associated kinase BIK1 during plant immunity. Mol Cell. 2014;54:43–55. doi:10.1016/j.molcel.2014.02.021. PMID:24630626
- Straus MR, Rietz S, Ver Loren van Themaat E, et al. Salicylic acid antagonism of EDS1-driven cell death is important for immune and oxidative stress responses in Arabidopsis. Plant J. 2010;62:628–640. doi:10.1111/j.1365-313X.2010.04178.x. PMID:20163553
- Palma K, Thorgrimsen S, Malinovsky FG, et al. Autoimmunity in Arabidopsis acd11 is mediated by epigenetic regulation of an immune receptor. Plos Pathogens. 2010;6:e1001137. doi:10.1371/journal.ppat.1001137. PMID:20949080
- Gomes LC, Scorrano L. Mitochondrial morphology in mitophagy and macroautophagy. Biochim Biophys Acta. 2013;1833:205–212. doi:10.1016/j.bbamcr.2012.02.012. PMID:22406072
- Jones A. Does the plant mitochondrion integrate cellular stress and regulate programmed cell death? Trends Plant Sci. 2000;5:225–230. doi:10.1016/S1360-1385(00)01605-8. PMID:10785669
- Maxwell DP, Nickels R, McIntosh L. Evidence of mitochondrial involvement in the transduction of signals required for the induction of genes associated with pathogen attack and senescence. Plant J. 2002;29:269–279. doi:10.1046/j.1365-313X.2002.01216.x. PMID:11844105
- Dutilleul C, Garmier M, Noctor G, et al. Leaf mitochondria modulate whole cell redox homeostasis, set antioxidant capacity, and determine stress resistance through altered signaling and diurnal regulation. Plant Cell. 2003;15:1212–1226. doi:10.1105/tpc.009464. PMID:12724545
- Swidzinski JA, Leaver CJ, Sweetlove LJ. A proteomic analysis of plant programmed cell death. Phytochemistry. 2004;65:1829–1838. doi:10.1016/j.phytochem.2004.04.020. PMID:15276441
- Cvetkovska M, Vanlerberghe GC. Alternative oxidase impacts the plant response to biotic stress by influencing the mitochondrial generation of reactive oxygen species. Plant Cell Environ. 2013;36:721–732. doi:10.1111/pce.12009. PMID:22978428
- Twig G, Hyde B, Shirihai OS. Mitochondrial fusion, fission and autophagy as a quality control axis: the bioenergetic view. Biochim Biophys Acta. 2008;1777:1092–1097. doi:10.1016/j.bbabio.2008.05.001. PMID:18519024
- Wei Y, Chiang WC, Sumpter R Jr, et al. Prohibitin 2 is an inner mitochondrial membrane mitophagy receptor. Cell. 2017;168:224–238. doi:10.1016/j.cell.2016.11.042. PMID:28017329
- van Doorn WG. Classes of programmed cell death in plants, compared to those in animals. J Exp Bot. 2011;62:4749–4761. doi:10.1093/jxb/err196. PMID:21778180
- Barceló AR. Lignification in plant cell walls. Int Rev Cytol. 1997;176:87–132. doi:10.1016/S0074-7696(08)61609-5. PMID:9394918
- Xu L, Zhu L, Tu L, Liu L, et al. Lignin metabolism has a central role in the resistance of cotton to the wilt fungus Verticillium dahliae as revealed by RNA-Seq-dependent transcriptional analysis and histochemistry. J Exp Bot. 2011;62:5607–5621. doi:10.1093/jxb/err245. PMID:21862479
- Pomar F, Novo M, Bernal MA, et al. Changes in stem lignins (monomer composition and crosslinking) and peroxidase are related with the maintenance of leaf photosynthetic integrity during Verticillium wilt in Capsicum annuum. New Phytol. 2004;163:111–123. doi:10.1111/j.1469-8137.2004.01092.x.
- Kwon SI, Cho HJ, Kim SR, et al. The Rab GTPase RabG3b positively regulates autophagy and immunity-associated hypersensitive cell death in Arabidopsis. Plant Physiol. 2013;161:1722–1736. doi:10.1104/pp.112.208108. PMID:23404918
- Nelson BK, Cai X, Nebenführ A. A multicolored set of in vivo organelle markers for co-localization studies in Arabidopsis and other plants. Plant J. 2007;51:1126–1136. doi:10.1111/j.1365-313X.2007.03212.x. PMID:17666025
- Chaudhary P, Godara S, Cheeran AN, et al. Fast and accurate method for leaf area measurement. IJCA. 2012;49:22–25. doi:10.5120/7655-0757.
- Wang ZQ, Xu XY, Gong QQ, et al. Root proteome of rice studied by iTRAQ provides integrated insight into aluminum stress tolerance mechanisms in plants. J Proteomics. 2014;98:189–205. doi:10.1016/j.jprot.2013.12.023. PMID:24412201
- Nesvizhskii AI, Keller A, Kolker E, et al. A statistical model for identifying proteins by tandem mass spectrometry. Anal Chem. 2003;75:4646–4658. doi:10.1021/ac0341261. PMID:14632076
- Shadforth IP, Dunkley TPJ, Lilley KS, et al. i-Tracker: For quantitative proteomics using iTRAQ (TM). BMC Genomics. 2005;6:145. doi:10.1186/1471-2164-6-145. PMID:16242023
- Du Z, Zhou X, Ling Y, et al. agriGO: a GO analysis toolkit for the agricultural community. Nucleic Acids Res. 2010;38:W64–W70. doi:10.1093/nar/gkq310. PMID:20435677
- Kanehisa M, Goto S, Sato Y, et al. KEGG for integration and interpretation of large-scale molecular data sets. Nucleic Acids Res. 2012;40:D109–D114. doi:10.1093/nar/gkr988. PMID:22080510
- Wang HY, Wang J, Gao P, et al. Down-regulation of GhADF1 gene expression affects cotton fibre properties. Plant Biotechnol J. 2009;7:13–23. doi:10.1111/j.1467-7652.2008.00367.x. PMID:18761653
- Pantelides IS, Tjamos SE, Paplomatas EJ. Ethylene perception via ETR1 is required in Arabidopsis infection by Verticillium dahliae. Mol Plant Pathol. 2010;11:191–202. doi:10.1111/j.1364-3703.2009.00592.x. PMID:20447269
- Li F, Vierstra RD. Arabidopsis ATG11, a scaffold that links the ATG1-ATG13 kinase complex to general autophagy and selective mitophagy. Autophagy. 2014;10:1466–1467. doi:10.4161/auto.29320. PMID:24991832
- Klionsky DJ, Abdelmohsen K, Abe A, et al. Guidelines for the use and interpretation of assays for monitoring autophagy (3rd edition). Autophagy. 2016;12:1–222. doi:10.1080/15548627.2015.1100356. PMID:26799652
- Moreira-Vilar FC, Siqueira-Soares RdC, Finger-Teixeira A, et al. The acetyl bromide method is faster, simpler and presents best recovery of lignin in different herbaceous tissues than Klason and thioglycolic acid methods. PLoS One. 2014;9:e110000. doi:10.1371/journal.pone.0110000. PMID:25330077
- Han LB, Li YB, Wang HY, et al. The dual functions of WLIM1a in cell elongation and secondary wall formation in developing cotton fibers. Plant Cell. 2013;25:4421–4438. doi:10.1105/tpc.113.116970. PMID:24220634
- Song X, Guo H, Zhang G, et al. OsPRA2 fine-tunes rice brassinosteroid receptor. Plant Sign Behav. 2017;4:e1257455. doi:10.1080/15592324.2016.1257455. PMID:28402719
- Dagdas YF, Belhaj K, Maqbool A, et al. An effector of the Irish potato famine pathogen antagonizes a host autophagy cargo receptor. eLife. 2016;5:e10856. doi:10.7554/eLife.10856. PMID:26765567