ABSTRACT
The present study describes the response of Zea mays to hematite nanoparticles (NPs) using five concentrations ranged from 500 to 8000 mg kg−1, and Cd2+ concentrations (110 or 130 mg kg−1 Cd2+) only or combined with 500 mg kg−1 NPs, using soil culture. The endpoints measured were root/shoot growth, elements uptake, ultra-structural alterations, lipid peroxidation, some antioxidant enzyme activities, and their relative gene expressions. The results indicated that hematite NPs exhibited a dual behavior, in which 500 mg kg−1 NPs significantly enhanced maize growth, while 4000 and 8000 mg kg−1 NPs significantly increased lipid peroxidation and superoxide dismutase (SOD) activity displaying positive correlation with SOD expression in shoots (r = +0.472, p < .05). Ultrastructure micrographs revealed the appearance of aggregated NPs inside the vacuoles. The stunted growth, perturbed ultra-structure, and high lipid peroxidation were used as toxicity biomarkers for Cd2+. However, combined treatments of 500 mg kg−1 NPs and Cd2+ significantly stimulated growth and glutathione reductase activity, while significantly reduced catalase (CAT) activity displaying positive correlation with CAT expression in roots (r = +0.694, p < .01). In conclusion, hematite NPs could alleviate Cd2+ toxicity not at the level of antioxidant defense, but by affecting mechanisms of Cd2+ detoxification.
1. Introduction
Nanoparticles (NPs) are particles having at least one dimension between 1 and 100 nm in diameter. The major factor that makes NPs more technically interesting than bulk materials are their small size which provides a high surface area to volume ratio leading to their high reactivity (Buzea et al. Citation2007). Hence, NPs became highly attractive for the implementation in different fields such as photocatalysis, energy, and environment (Kailasam et al. Citation2011; Ashokkumar et al. Citation2014).
Iron deficiency is one of the major factors affecting crop yields, food quality, and human nutrition, since iron (Fe) is considered to participate in many physiological processes such as respiration, chlorophyll biosynthesis, and redox reactions (Zuo and Zhang Citation2011). Interstingly, iron oxide NPs such as hematite (α-Fe2O3), magnetite (Fe3O4), and maghemite (γ-Fe2O3) NPs could be applied as an efficient crop fertilizer for the alleviation of Fe-deficiency, and thus increasing Fe availablilty to plants (Ren et al. Citation2011; Rui et al. Citation2016; Li et al. Citation2018). Hematite is the most thermodynamically stable iron oxide, and is widely used in different products including pigments, catalysts, and photo-electrochemical cells (Esmaeili et al. Citation2011; Ahmmad et al. Citation2013).
Oxidative stress is one of the main mechanisms through which NPs could mediate their effects in plants (Yang et al. Citation2017). Through the enhanced generation of reactive oxygen species (ROS), oxidative stress consequently causes damage to cellular macromolecules such as proteins, lipids, and DNA depending on the stress intensity. In order to overcome the oxidative damage, series of detoxification reactions are catalyzed by antioxidant enzymes such as superoxide dismutase (SOD), catalase (CAT), ascorbate peroxidase (APX), and glutathione reductase (GR) (Choudhury et al. Citation2013). Meanwhile, the impact of iron oxide NPs on the antioxidant enzyme activities has been reported in different plant species (Wang et al. Citation2016; Hu et al. Citation2017). Moreover, gene expression analyses of different genes such as genes related to oxidative stress response could potentially constitute promising molecular biomarker for the detection of NPs-induced toxicity in plants (Nair and Chung Citation2015; Zhang et al. Citation2018).
Cadmium is considered to be one of the extremely toxic heavy metal pollutants that has been spreading with an increasing frequency in soil due to various anthropogenic activities (Zhang et al. Citation2005). It is known to stimulate the production of free radicals in plants, and hence disrupts the defense system against oxidative damage (Li et al. Citation2016b). The application of NPs in remediating polluted soils has gained great attention since small size and large specific surface area of NPs make them important binding phases for both organic and inorganic contaminants (Wang et al. Citation2012). Among the most widely used NPs, iron oxide NPs have been reported as important scavengers of the most toxic forms of cadmium (II), chromium (VI), arsenic (III), and lead (II) from contaminated soils and water (Zuverza-Mena et al. Citation2017). Iron oxide NPs are cheap, abundant, environment-friendly, and have been proved to own an extensive application prospect in environmental remediation (Wang et al. Citation2012). Moreover, recent studies have been established concerning the effective role of iron oxide NPs in reducing heavy metal-induced toxicity in plants (Konate et al. Citation2017; Sebastian et al. Citation2018).
Zea mays L. is one of the most widely cultivated crops in the world and considered to be a critical source of several macro- and micronutrients, and thus serves as a nutrition source for people and livestock worldwide (Kean et al. Citation2008). In the present study, using maize as the model plant, we conducted a soil culture experiment to investigate the separate and combined effects of hematite NPs and Cd2+ on maize growth. Transmission electron microscope (TEM) was used to (i) examine hematite NPs and Cd2+ localization within maize root cells, and (ii) study ultra-structural alterations in different cell organelles in response to different treatments. Later, biochemical assays were carried out to determine the oxidative stress responses in maize including membrane damage and changes in antioxidant enzyme activities. Finally, gene expression patterns of oxidative stress related genes were investigated using qRT-PCR. To the best of our knowledge, several studies indicated that Fe could reduce Cd2+ toxicity in plants, however, the effect of nano-formulations on Cd2+ uptake by plants is rarely studied. Therefore, in our study, the addition of hematite NPs to metal-contaminated soil could possibly stimulate the growth and development of maize through the reduction of heavy metal phyto-availability.
2. Materials and methods
2.1. Test plant and growth substrate
Maize grains (Zea mays L., sakha 10) were kindly provided by the Agricultural Research Center, Ministry of Agriculture (El-Giza, Egypt). The grains were tested for the germination viability and then stored at 4°C until further use. Quartz sand was used as an inert growth substrate, it was sieved through nylon mesh, soaked in 2% hydrochloric acid (HCl) overnight, washed with running tap water, and then rinsed with distilled water several times until a neutral pH was achieved. The sand was first air dried for two days, and then oven dried at 100°C for another two days to ensure complete dryness and sterilization from any contaminants.
2.2. Synthesis and characterization of hematite NPs
Hematite NPs were synthesized according to the co-precipitation method of Kang et al. (Citation1996) and Peternele et al. (Citation2014). Briefly, 12 ml of [1 M ferric chloride hexahydrate (FeCl3.6H2O)/2 M HCl] were mixed with 30 ml of [0.2 M ferrous sulfate heptahydrate (FeSO4.7H2O)/0.2 M HCl] and slowly added to 123 ml of 1.22 M sodium hydroxide (NaOH) as precipitating agent, with continuous stirring. After complete addition, stirring was continued for another 30 minutes. The obtained black product was washed several times with distilled water and decanted to remove unwanted cations and reduce pH to ∼ 7. The resultant product was oven-dried at 50°C, grinded to a fine powder and then burned in muffle furnace at 430°C for 3 hours to obtain hematite NPs (calcination step). The structural characterization and the average size of hematite NPs were determined using a high-resolution transmission electron microscope (TEM; JEOL JEM-2100, Japan). Hematite powder was first dispersed in distilled water, ultra-sonicated to ensure a proper separation of particles, then a droplet of solution was added on TEM-copper grid, and left to air dry before imaging on the microscope. The mineralogy of hematite NPs was determined via X-ray diffraction (XRD) analysis. The NPs powder was loaded on a glass plate in a thin layer and scanned by using Cu Kα radiation using wavelength (λ) =1.5406 Å, with a powder diffractometer (Shimadzu Xlab 6100, Japan). The sample was scanned from 5 to 80° 2 theta (2θ), with a scan speed rate of 12˚ min−1. The crystallographic structure of the NPs and the average spacing between the atomic planes inside the NPs were determined. The XRD data were graphed out using OriginPro8 graphing and analysis software, in order to analyze the characteristic peaks for hematite. Later, screening of different concentrations of hematite NPs (100–10,000 mg NPs Kg−1 soil) was performed by mixing NPs with the soil medium, in order to evaluate the changes in maize growth traits, and then five sublethal concentrations were selected (500, 1000, 2000, 4000 and 8000 mg NPs kg−1 soil) representing the most significant changes in maize growth.
2.3. Preparation of cadmium stock solutions
Cadmium (Cd2+) used in this study was in the form of cadmium nitrate [Cd (NO3)2·4H2O]. The stock solutions were prepared by dissolving a series of different weights of Cd2+ salt in distilled water, and then applied to the soil to test the changes in growth traits of maize under different concentrations (10–160 mg Cd2+ kg−1 soil). Following the screening phase, only two sublethal concentrations including 110 and 130 mg Cd2+ kg−1 soil were selected and used in this study, on the basis of demonstrating the most significant morphological toxicity symptoms in maize seedlings.
2.4. Experimental setup and design
All experiments were conducted in culturing pots (10 cm diameter and 8 cm height) filled with 500 g of homogenous oven-dried soil. Each pot was furnished with a hole at the bottom and this was covered with filter paper before filling with the soil. Three independent experiments were performed in a completely randomized block design with five replicates of each experimental set. In the first set of experiment, a fine powder of hematite NPs was gently mixed with the soil to obtain homogenous NPs mixture of different concentrations (500, 1000, 2000, 4000 and 8000 mg kg−1). The pots were watered with 65 ml distilled water at the starting point, and then occasionally with a certain amount of water in order to keep constant water holding capacity. The second set of experiment was conducted using two Cd2+ levels (110 or 130 mg kg−1). In the third set of experiment, the soil that had been amended with 500 mg kg−1 hematite NPs was mixed with 110 or 130 mg kg−1 Cd2+ levels in order to study the combined effect of NPs and Cd2+. For the second and third experiments, pots were irrigated with 65 ml of 110 or 130 mg kg−1 Cd2+ solution at the starting point, and then with distilled water occasionally to maintain the constant water holding capacity. Soil without NPs nor Cd2+ addition was used as the control for the three experimental sets in which the pots were watered with 65 ml distilled water at the starting point, and then occasionally with a certain amount of water in order to keep constant water holding capacity. Furthermore, uniform and viable maize grains were sterilized with 5% (v/v) sodium hypochlorite for 5 minutes to avoid fungal contaminations and then rinsed three times with distilled water. Sterilized grains were then soaked in distilled water and incubated in the dark for 24 hours at room temperature. Following soil homogenization, three of the pre-soaked grains were placed in each pot beneath the soil surface (1 cm depth). Pots were incubated in the dark at room temperature for 24 hours and then were transferred to the botanical garden at Faculty of Science, Alexandria University, Egypt, under natural conditions where the temperature ranged from 25°C to 30°C during the day and 20°C to 24°C during the night. The geographic coordinates of the botanical garden were 31°12′6.3″ N, 29°54′56.9″ E, and elevation 36 meters. The pots were kept under normal light intensity 14/10 hours of light/dark and the relative air humidity was about 85%. After seven days, maize seedlings were carefully harvested, washed with running tap water then with distilled water to remove adhered sand, blotted dry gently between filter papers, and finally, carefully chosen homologous seedlings were used for further investigations.
2.5. Growth parameters determination
The control and treated maize seedlings were laid flat on a moist black cloth where a centimeter scale was fixed alongside the plants, and the exact length of the roots and shoots were determined in centimeter (cm) by reference to the scale. Then, the roots and shoots of homologous seedlings were oven-dried at 60°C till constant weight for dry weight determination.
2.6. Analysis of metals uptake in plant roots
Sample preparation, metal analysis, and quality control were carried out according to the standard method of Kimbrough and Wakakuwa (Citation1989). An aliquot (200 mg) of well-mixed, homogeneous, oven-dried roots of control, and treated maize seedlings were mixed with nitric acid (HNO3) and refluxed in a 250 ml Griffin beaker. At first, 3 ml of concentrated HNO3 were added to the sample in the beaker and covered with a ribbed watch glass. Then, the mixture was heated on a hot plate at 90–95°C and left to evaporate to a low volume. After cooling, the previous step was repeated with additional portions (3 ml) of HNO3 until the digested solution either turned into a lighter color or reached a stable color, and the digestate was then refluxed with a small portion of HCl (3 ml) for complete digestion. Finally, the sample was filtered through filter paper (Whatman 42, diameter 110 mm) to remove silicates and other insoluble materials that could clog the nebulizer of the instrument. Then, the beaker walls and watch glass were washed with deionized water and the filter paper was rinsed with diluted HNO3 (10%). The final volume was adjusted to 25 ml with deionized water. To determine different metal content, the solutions were subjected to Inductively Coupled Plasma-Optical Emission Spectroscopy (ICP-OES; Agilent 5100 VDV, USA). The content of iron (Fe), cadmium (Cd2+), and calcium (Ca2+) in maize roots was computed as parts per million (ppm). Flow rates of plasma, auxiliary, and nebulizer of ICP-OES were kept at 12, 1, 0.7 ml min−1, respectively. The sample uptake and stabilization time were 10 seconds for each sample. The wavelengths for Fe, Cd2+, and Ca2+ were 238.204, 214.439 and 317.933 nm, respectively.
2.7. Preparation of plant specimens for TEM analysis
Root segments (were cut just above the apical part of the root tip) of control, and treated maize seedlings were immediately fixed after harvesting by immersion in 4% formaldehyde and 1% glutaraldehyde fixative mixture in 0.1 M sodium phosphate buffer (pH 7.4) for 24 hours at 4°C (pre-fixation). Roots were then post fixed in 2% (w/v) osmium tetraoxide in the same phosphate buffer for 2 hours at 4°C. The fixed tissues were then rinsed with the same buffer and dehydrated in a graded series of ethanol (50%, 70%, 80%, 90% and 99.9%). Dehydrated tissues were embedded in the Epon-araldite resin mixture where infiltration and embedding were conducted in a graded resin of 30%, 50%, 70% for 2 hours, and of 100% overnight. The specimens were embedded in capsules and polymerized for 72 hours at 60°C in oven. Sections of 1 µm thick were cut, stained with toluidine blue, and examined by a light microscope to identify a suitable area for ultra-structural evaluation (Reynolds Citation1963). Ultrathin sections (60 nm thick) were cut using ultra-microtome (RMC PT-XL PowerTome, USA) with a diamond knife and then were double stained with uranyl acetate for 30 minutes and lead citrate for 20–30 minutes (Reynolds Citation1963). The ultrathin sections were then mounted on copper grids with 300 square mesh and the ultra-structure visualization and photographing were carried out using high-resolution TEM (JEOL JEM-2100, Japan).
2.8. Determination of membrane damage
Lipid peroxidation in plant tissues was determined by measuring the malondialdehyde (MDA) content which is the end product of peroxidation of polyunsaturated fatty acids of membrane lipids. As described by Heath and Packer (Citation1968), fresh plant tissues (200 mg) of roots/shoots of control, and treated maize seedlings were homogenized in 5 ml of 0.1% (w/v) trichloroacetic acid (TCA) on an ice bath. The homogenates were then centrifuged at 12,000 × g for 15 minutes at 4°C and the supernatants were collected in clean falcon tubes. The reaction mixtures contained 0.5 ml aliquot of each plant extract and 1 ml of 20% (w/v) TCA containing 0.5% (w/v) thiobarbituric acid (TBA). The mixture was kept in boiling water bath for 30 minutes, then cooled immediately on an ice bath, and followed by centrifugation at 10,000×g for 10 minutes. The non-specific absorbance of the colored supernatant at 600 nm was subtracted from the maximum absorbance at 532 nm for MDA measurement. Finally, the concentration of MDA was calculated using an extinction coefficient (Δε) = 155 mM−1 cm−1.
2.9. Antioxidant enzyme activities
2.9.1. Estimation of superoxide dismutase (SOD, EC 1.15.1.1) activity
Fresh plant tissues (2 g) of roots/shoots of control and treated maize seedlings were homogenized in 5 ml of 50 mM potassium phosphate buffer (pH 7.8) containing 0.1 mM EDTA and 1% insoluble polyvinyl pyrrolidone (PVP) using a chilled mortar and pestle in liquid nitrogen, then followed by centrifugation of the homogenates at 10,000×g for 20 minutes. The supernatants were collected and used for the assay of SOD enzyme activity according to the method described by Beyer and Fridovich (Citation1987) using a spectrophotometer at 25°C. The reaction mixture was prepared by mixing 27 ml of 50 mM potassium phosphate (pH 7.8), 1.5 ml of L-methionine (12 mM), 1 ml of nitrobluetetrazolium (NBT) salt (50 µM), and 0.75 ml of Triton X-100. An aliquot (1 ml) of this mixture was added to glass tubes, followed by 20 μl of enzyme extract and 10 μl of riboflavin (10 µM). The test tubes containing the reaction mixtures were gently shacked and exposed to light for 7 minutes by immersing the glass tubes in a cylindrical glass container three-fourth filled with clean water in which the whole set was placed in an aluminum foil-lined box containing two of 20 watt fluorescent lamps. A control glass tube containing 20 μl of the buffer instead of the enzyme extract was run in parallel and the increase in absorbance due to formazan formation was read at wavelength 560 nm. Under the described conditions, the increase in absorbance without the enzyme extract was taken as 100% and the enzyme activity was calculated by determining the percentage inhibition per minute, and 50% of inhibition was taken as equivalent to one unit (U) of SOD activity.
2.9.2. Preparation of enzyme extracts for estimation of CAT, APX and GR activities
The enzyme extracts were prepared following the method of Gossett et al. (Citation1994). Two grams of fresh roots and shoots of control and treated maize seedlings were ground to fine powder in liquid nitrogen. The samples were homogenized in 5 ml of 50 mM potassium phosphate buffer (pH 7.0) containing 1 mM EDTA, 1 mM D-isoascorbic acid, 2% (w/v) PVP, and 0.05% (w/v) Triton X-100 using a chilled mortar and pestle. The homogenates were centrifuged at 10,000 × g for 15 minutes at 4°C and the supernatants were collected and used for the assays of catalase, ascorbate peroxidase, and glutathione reductase.
2.9.2.1. Estimation of catalase (CAT, EC 1.11.1.6) activity
Catalase activity was assayed by measuring the rate of H2O2 disappearance using a spectrophotometer at 25°C. The reaction mixture was prepared using 950 μl of 50 mM potassium phosphate buffer (pH 7.0) containing 10.5 mM H2O2 and then 50 μl of the enzyme extract were added to start the reaction (Miyagawa et al. Citation2000). The initial linear rate of decrease in absorbance at 240 nm was used to calculate the enzyme activity after 2 minutes, taking the extinction coefficient (Δε) = 43.6 mM−1.cm−1.
2.9.2.2. Estimation of ascorbate peroxidase (APX, EC 1.11.1.11) activity
Ascorbate peroxidase activity was assessed as described by Nakano and Asada (Citation1981) using a spectrophotometer at 25°C. The reaction mixture was 950 μl of 50 mM potassium phosphate buffer (pH 7.0) containing 0.2 mM EDTA, 0.5 mM ascorbic acid and 0.25 mM H2O2. The reaction was started after adding 50 μl of the enzyme extract. The decrease in absorbance at wavelength 290 nm after 1 minute was recorded and the amount of ascorbate oxidized was calculated from the extinction coefficient (Δε) = 2.8 mM−1 cm−1.
2.9.2.3. Estimation of glutathione reductase (GR, EC 1.6.4.2) activity
Glutathione reductase activity was determined according to the method described by Smith et al. (Citation1988) using a spectrophotometer at 25°C. The reaction mixture consisted of 1000 μl of 0.2 M potassium phosphate (pH 7.5) containing 1 mM EDTA; 500 μl of 3 mM 5,5-dithio-bis-(2-nitrobenzoic acid) ‘DTNB’ in 0.01 M potassium phosphate buffer (pH 7.5); 200 μl of H2O; 100 μl of enzyme extract; 100 μl of 2 mM NADPH; and 100 μl of 20 mM oxidized glutathione (GSSG). The reaction was initiated by the addition of GSSG. The increase in the absorbance was monitored after 5 minutes at wavelength 412 nm.
2.9.3. Measurement of soluble protein content
Soluble protein content was determined by the protein-dye binding method described by Bradford (Citation1976), using bovine serum albumin (BSA) as a protein standard. Bradford reagent was prepared by weighing 100 mg of Coomassie Brilliant Blue G-250, adding 50 ml of 95% ethanol and 100 ml of 85% (w/v) phosphoric acid, and the resulting solution was diluted to a final volume of 1000 ml at room temperature. Final concentrations in the reagent were 0.01% (w/v) Coomassie Brilliant Blue G-250, 4.7% (w/v) ethanol, and 8.5% (w/v) phosphoric acid. 0.5 ml of the enzymes extract was added to 2.5 ml of Bradford reagent, and after standing for 2 minutes, the absorbance was measured at 595 nm.
2.10. Gene expression analysis
2.10.1. Total RNA isolation
Root and shoot tissues of control and treated maize seedlings were stored at −80˚C. The frozen tissues were thawed on ice and 100 mg of each frozen sample was used for RNA extraction. Tissue samples were cut into smaller pieces, grinded and lysed in 750 µl of Trizol reagent (Ambion, life technologies, USA). The phenol lysates were transferred into RNase-free tubes and 250 µl of molecular biology grade chloroform-isoamyl (Sigma-Aldrich) were added, mixed vigorously by vortexing and then left to settle down for 5 minutes at room temperature. Organic and aqueous phases were separated by centrifugation at 12,000 × g for 15 minutes at 4°C. The last step was repeated again to ensure a high purity of the RNA and to eliminate any protein or polysaccharides contamination. The aqueous phase of each sample was transferred to a new tube and an equal volume of ice-cold absolute ethanol was added alongside with 1 µl of GlycoBlue™ (Thermo Fisher Scientific, USA) and mixed well by gentle rotation. The RNA was left to precipitate for 1 hour at −80°C followed by centrifugation at 12,000 × g for 15 minutes at 4°C. The RNA pellet was further washed by 1 ml of 70% ice-cold ethanol and precipitated by centrifugation at 12,000 × g for 10 minutes at 4°C. The last step was repeated again to remove salt contaminants. The RNA pellet was left to dry at room temperature for 5 minutes and was re-suspended in 50 µl of RNase-free water. The samples were further precipitated by adding 25 µl of isopropanol and passed through spin columns (ReliaPrep™ RNA Miniprep System, Promega, USA). Following the manufacturer's instructions, the DNase treatment was performed on columns and RNA was finally eluted in 50 µl of nuclease-free water. The concentration of each RNA sample was determined using a NanoDrop spectrophotometer (ThermoFisher Scientific, USA), and then the purified RNA samples were kept at −80˚C until use.
2.10.2. Reverse transcription and cDNA synthesis
In order to synthesize the first complementary strand DNA (cDNA), 500 ng of each RNA sample was reverse transcribed using AMV reverse transcriptase system (Promega, USA). For each sample, 500 ng of total RNA was adjusted to 10 µl volume using nuclease-free water. For each reaction, 1µl of Oligo (dT)15 primer (0.5µg/µl) was added and incubated for 5 minutes at 70°C, then another 5 minutes at 4˚C. The reverse transcription mixture was prepared according to the manufacturer's instructions using 2 µl of 10 mM dNTPs mix, 2 µl of 10×reverse transcription buffer, 4 µl of 60 mM magnesium chloride (MgCl2), 0.5 µl of 1 U/µl Recombinant RNasin® Ribonuclease Inhibitor and 0.5 µl of 15 U/µl AMV Reverse Transcriptase. The final volume of each sample was adjusted to 20 µl. The reaction mixtures were placed in a thermal cycler (Eppendorf Mastercycler®, Germany) at pre-wared for 5 minutes at 25°C, and then the reverse transcriptase was activated for 1 hour at 42°C. The reactions were heat inactivated for 15 minutes at 70°C. The cDNA mixtures were diluted with nuclease-free water to a final volume of 200 µl with a concentration of 2.5 ng/µl.
2.10.3. Quantitative-Real time PCR (qRT-PCR)
For each gene of interest, the qRT-PCR reaction was done in triplicates. Each reaction mixture was prepared with 3 µl of cDNA (7.5 ng), 3.5 µl of nuclease-free water, 7.5 µl of SYBR™ Green PCR Master mix (Applied Biosystems®, ThermoFisher Scientific, USA) and 1 µl (10 µM) of primer (). The thermal cycling was performed as initial pre-heating step at 95°C for 10 minutes; 40 cycles of denaturing at 95°C for 5 seconds, annealing at 60°C for 30 seconds and extension at 72°C for 30 seconds using qRT-PCR (ViiA™ 7, Applied Biosystems®, ThermoFisher Scientific, USA).
Table 1. Name and sequence of primers used in Quantitative-Real Time PCR (qRT-PCR) analysis.
2.10.4. Calculation of relative gene expression
The Comparative Threshold Cycle (CT) values were exported into a spreadsheet and the relative expression of each sample was calculated according to the method of Livak and Schmittgen (Citation2001). At first, the CT values of the target gene were normalized to that of the reference gene (Actin) (), for both control and test sample, where Δ CT(test) = CT(target, test) – CT(reference, test), Δ CT(control) = CT(target, control) – CT(reference, control). Second, Δ CT of the test sample was normalized to the Δ CT of the control sample, where ΔΔ CT(test) = Δ CT(test) − Δ CT(control). Finally, the relative gene expression was calculated as follows: 2−ΔΔCT = Normalized gene expression. The result obtained is the fold increase (or decrease) of the target gene in the test sample relative to the control and is normalized to the expression of the reference gene. Normalizing the expression of the target gene to that of the reference gene compensates for any difference in the amount of the sample tissue. The statistical correlation between relative gene expressions and enzyme activities of superoxide dismutase and catalase were carried using Bivariate Pearson Correlation with SPSS 20 Statistical Analysis Software.
2.11. Statistical analysis
Each concentration was set in at least triplicate including the control in a completely random design. Separate sets of plants were used for growth parameters determination, elemental analysis, physiological measurements, and molecular studies. The results obtained from different experiments were expressed as mean values ± standard deviation (SD). The significance of the difference between mean values was determined at P < .05 using Duncan's multiple range test. One way analysis of variance (ANOVA) for all experimental data were performed using SPSS 20 Statistical Analysis Software following the methods of Sokal and Rohlf (Citation2013). The statistical correlation between relative gene expressions and enzyme activities of superoxide dismutase and catalase were carried using Bivariate Pearson Correlation with SPSS 20 Statistical Analysis Software, and a probability level of P < .05 and P < .01 was considered significant.
3. Results
3.1. Hematite NPs characterization
The TEM images of hematite NPs showed that their average diameter ranged from 32.0 to 35.78 nm and their shapes varied between spherical and hexagonal ((A)). The identity and purity of hematite NPs were verified by XRD ((B)). The diffraction peaks observed were at 2 theta (2θ) = 24.0, 32.9, 35.4, 40.6, 49.2, 53.9, 62.3 and 63.7 corresponding to the standard diffraction patterns of hematite (Joint Committee on Powder Diffraction Standards ‘JCPDS’ no. 079-0007). Thus, the characteristic pattern of peaks for hematite was illustrated with no detectable peaks corresponding to other minerals.
3.2. Root and shoot growth
Exposure to the lowest hematite NPs concentration (500 mg kg−1) led to a significant increase in root and shoot lengths of maize by about 30% and 15%, respectively, compared to their controls ((A)). However, lengths were obviously diminished starting from 4000 mg kg−1 NPs ((A)). Consequently, 8000 mg kg−1 hematite NPs significantly reduced root and shoot lengths by about 85% and 34% respectively, relative to their corresponding controls ((A)). Dry weights of maize roots and shoots behave in a similar way to their corresponding lengths. For instance, the highest root and shoot mass was found at the lowest hematite NPs level treatment, which increased by about 46% and 26% respectively, compared to their respective controls ((B)). On the other hand, 8000 mg kg−1 hematite NPs significantly decreased root and shoot dry weights by 56% and 52% respectively, relative to their corresponding controls ((B)).
Figure 2. Changes in length and dry weight of maize roots and shoots. A,B: seedlings treated with different hematite NPs concentrations. C,D: seedlings treated with Cd2+ concentrations only or combined with 500 mg kg−1 hematite NPs. The values reported in the figure are means ± standard deviation of three replicates. Different letters on the bars (lower case for roots and uppercase for shoots) indicate significant differences among treatments as evaluated by Duncan's multiple comparison test (p < .05).
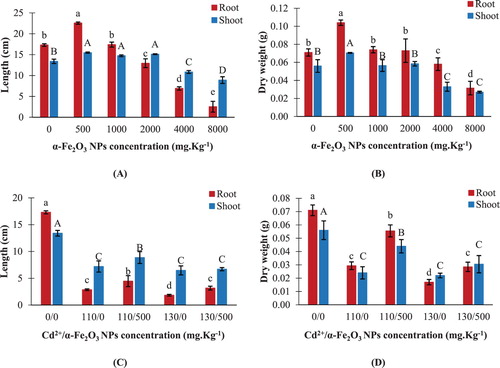
Cadmium at concentrations either 110 or 130 mg kg−1 displayed a significant reduction in root lengths by about 83% and 89%, and shoot lengths by about 46% and 52%, respectively, in comparison to their corresponding controls ((C)). On the contrary, combined treatment of 500 mg kg−1 hematite NPs and Cd2+ led to a noticeable increase in root and shoot lengths of maize, in comparison to Cd2+-treated seedlings. For instance, compared to 130 mg kg−1 Cd2+-treated seedlings, the amendment with a mixture of 500 mg kg−1 NPs and 130 mg kg−1 Cd2+ significantly increased root length by about 73%, but an insignificant change in shoot length was recorded under the same conditions ((C)). It was evident that a respective reduction in root and shoot dry weights was observed by about 59% and 57%, respectively, upon treating maize seedlings with 110 mg kg−1 Cd2+ ((D)). On the other hand, root and shoot dry weights were highly stimulated by about 89% and 83% respectively, upon treatment with a mixture of 500 mg kg−1 NPs and 110 mg kg−1 Cd2+, compared to the corresponding Cd2+-treated seedlings ((D)).
3.3. Elements content in roots
The internalization of hematite NPs in maize roots was expressed as total Fe which significantly increased by about 25%, 37%, 75%, 101% and 202% following an elevation of NPs concentrations from 500 to 8000 mg kg−1 respectively, in comparison to the control ((A)). Moreover, root calcium content reduced significantly at hematite NPs level treatments exceeding 2000 mg kg−1 ((B)).
Figure 3. Changes in elements uptake in maize roots. A,B: seedlings treated with different hematite NPs concentrations. C–E: seedlings treated with Cd2+ concentrations only or combined with 500 mg kg−1 hematite NPs. The values reported in the figure are means ± standard deviation of three replicates. Different letters on the bars indicate significant differences among treatments as evaluated by Duncan's multiple comparison test (p < .05).
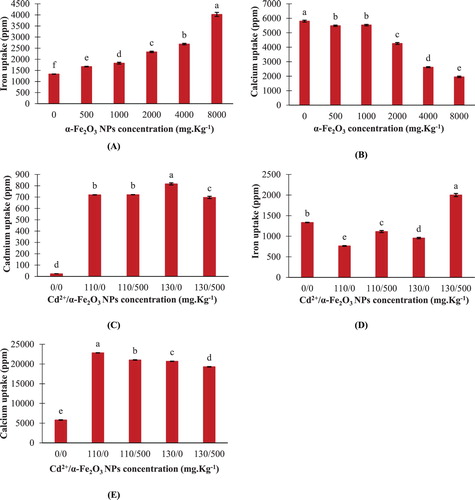
The ICP-OES analysis indicated an increase in the intracellular Cd2+ levels in maize roots confirming the uptake of Cd2+ from the soil ((C)). Interestingly, the addition of 500 mg kg−1 hematite NPs to the soil amended with 130 mg kg−1 Cd2+ resulted in a significant diminution of Cd2+ uptake by about 15%, compared to the corresponding Cd2+ treatment ((C)).
Cadmium toxicity either 110 or 130 mg kg−1 significantly reduced Fe content in maize roots by about 43% and 28% respectively, in comparison to the control ((D)). While significant stimulation of Fe uptake by about 46% and 109% was observed in seedlings grown in a mixture of 500 mg kg−1 hematite NPs with either 110 or 130 mg kg−1 Cd2+ respectively, compared to the corresponding Cd2+ treatments ((D)). Exposing maize seedlings to either 110 or 130 mg kg−1 Cd2+ resulted in a significant elevation in calcium uptake by about ∼2.9 and ∼2.6 fold over the control, respectively ((E)). Though, a significant reduction in calcium content by about 7.9% and 6.7% was observed in maize roots upon combined treatments of 500 mg kg−1 hematite NPs with either 110 or 130 mg kg−1 Cd2+ respectively, compared to their corresponding Cd2+ levels ((E)).
3.4. Ultrastructure observations
Transmission electron microscopy demonstrated unperturbed typical ultrastructure morphology of the control root tissues of maize seedlings in which cells exhibited thinly distributed small vacuoles, centrally located well-shaped nuclei containing nucleolus with normal chromatin distribution and distinct nuclear membrane ((A, B)). Moreover, root tip cells had round-shaped mitochondria and few number of peroxisomes ((C, D)). In addition, cell walls in the control root tip cells showed a thickness of 0.22–0.30 µm ((D)). For maize seedlings treated with the lowest hematite NPs concentration (500 mg kg−1), root tip cells appeared almost the same as the control with no detectable changes in organelles shape and size ((A–D)).
Figure 4. Transmission electron micrographs of control root tip cells of maize (A–D) showing N: nucleus, Nue: nucleolus, M: mitochondria, V: vacuole, P: peroxisome and CW: cell wall. Bars A – 10 µm; B – 10 µm; C – 5 µm; D – 2 µm. Magnification A – 500×; B – 800×; C – 1000×; D – 2000×.
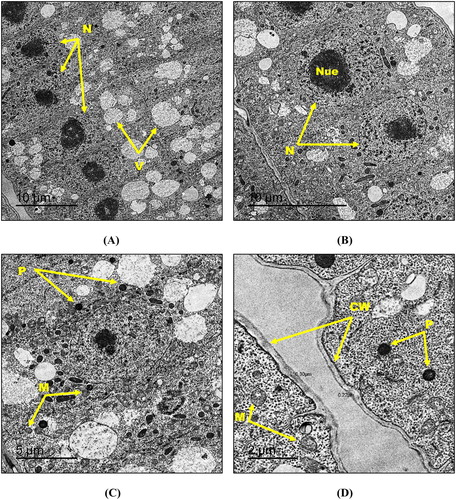
Figure 5. Transmission electron micrographs of root tip cells of maize treated with 500 mg kg−1 hematite NPs (A-D) showing N: nucleus, Nue: nucleolus, M: mitochondria, V: vacuole, P: peroxisome and CW: cell wall. Bars A – 20 µm; B – 10 µm; C – 5 µm; D – 2 µm. Magnification A – 400×; B – 800×; C – 1500×; D – 2000×.
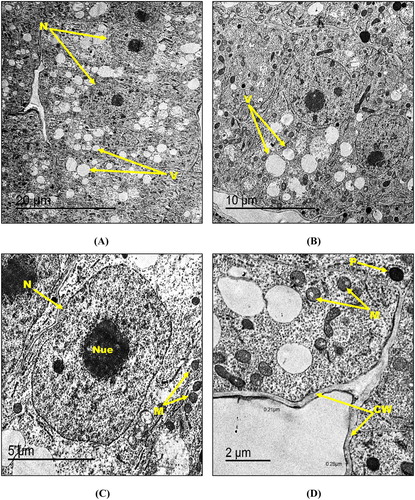
The exposure to the highest hematite NPs concentration (8000 mg kg−1) resulted in the appearance of aggregated NPs (black rings) within the vacuoles of the root tip cells. The shape of the NPs aggregates were shown in the insets of (A). Foremost and visible alterations like peripherally located irregularly-shaped nuclei with a fragmented and unequally distributed chromatin along with either an increase or a dimension of the nucleoli were observed in the root tip cells ((B)–(D)). Moreover, an increase in the number of vacuoles in the form of aggregates ((A, C)) and also numerous amyloplasts ((D)) were observed in the 8000 mg kg−1 NPs-treated root cells. In addition, these cells showed an increase in cell wall thickness (0.34–0.63 µm) relative to the control and to 500 mg kg−1-NPs-treated root cells ((E)).
Figure 6. Transmission electron micrographs of root tip cells of maize treated with 8000 mg kg−1 hematite NPs (A–E) showing N: nucleus, Nue: nucleolus, V: vacuole, Amy: amyloplast and CW: cell wall. Magnified TEM image (a) of NPs agglomerates are included as insets in (A). Bars A – 10 µm, a – 200 nm; B – 20 µm; C – 5 µm; D – 5 µm; E – 2 µm. Magnification A – 800×, a – 25,000×; B – 400×; C – 1000×; D – 1000×; E – 2000×.
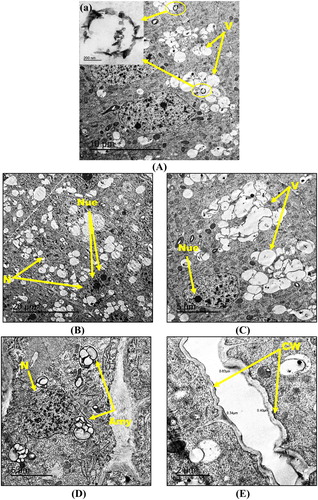
The TEM micrographs revealed that the treatment with 130 mg kg−1 Cd2+ induced the most severe ultra-morphological damage in maize root tip cells among all studied treatments. The most detectable alterations included high vacuolization events that led to a displacement of the cytoplasm by those enlarged vacuoles ((A)–(C)). Also, Cd2+ deposition was observed within the vacuoles in the form of dense granules ((B,C)). Moreover, nuclei were present in the form of highly damaged, irregularly-shaped, relatively small size structures and not localized in the center of the cells ((A,B)). Furthermore, less number of mitochondria was observed in Cd2+-treated root tip cells respective to the control, and the cell wall thickness increased to reach 0.25–0.68 µm ((D)).
Figure 7. Transmission electron micrographs of root tip cells of maize treated with 130 mg kg−1 Cd2+ (A–D) showing N: nucleus, Nue: nucleolus, M: mitochondria, V: vacuole, CW: cell wall and Cd: cadmium deposition. Bars A – 10 µm; B – 10 µm; C – 5 µm; D – 2 µm. Magnification A – 500×; B – 500×; C – 800×; D – 2000×.
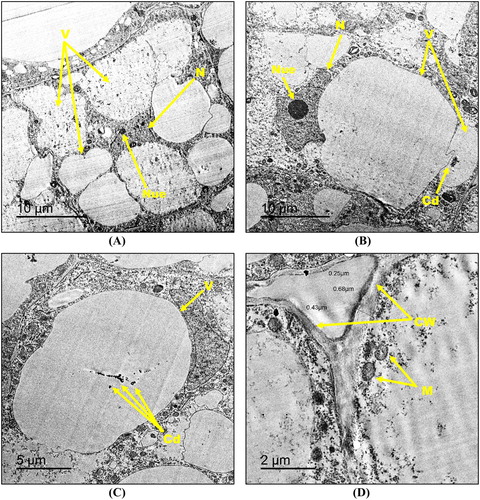
On the contrary, root cell structure relatively retained its integrity upon the exposure to combined treatment of 500 mg kg−1 hematite NPs and 130 mg kg−1 Cd2+, compared to the corresponding Cd2+ treated ones. Briefly, TEM micrographs showed root cells having centrally-located, regularly-shaped nuclei associated with an equal chromatin distribution along with occasional absence of the nucleoli in some cells ((A)–(C)). Moreover, mitochondria almost exhibited normal size, shape, and distribution ((D)). The root cells showed a slight increase in the cell wall thickness (0.33–0.36 µm) compared to the untreated control, nevertheless, it was still lower than the Cd2+-treated root cells ((D)). Though the only detectable damage sign was the slight increase in vacuoles size and number compared to the control which was still lower than the Cd2+-treated roots ((A)–(C)).
Figure 8. Transmission electron micrographs of root tip cells of maize treated with 130 mg kg−1 Cd2+ amended with 500 mg kg−1 hematite NPs (A-D) showing N: nucleus, Nue: nucleolus, M: mitochondria, V: vacuoles and CW: cell wall. Bars A – 10 µm; B – 10 µm; C – 5 µm; D – 2 µm. Magnification A – 600×; B – 600×; C – 800×; D – 2000×.
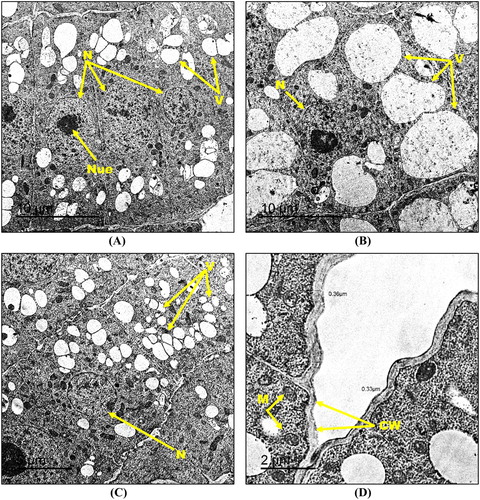
3.5. Lipid peroxidation levels
The MDA content significantly increased in maize roots and shoots by about 61% and 64% respectively, upon exposure to 8000 mg kg−1 hematite NPs, compared to their controls ((A)). However, insignificant changes in MDA content were detected in both roots and shoots upon exposure to 500 mg kg−1 NPs ((A)). Cadmium toxicity (130 mg kg−1) led to a significant increase in MDA content by 123% and 84% in maize roots and shoots respectively, relative to their corresponding controls ((B)). On the other hand, combined treatment of 500 mg kg−1 hematite NPs and 130 mg kg−1 Cd2+ significantly decreased MDA content in shoots by about 16%, compared to the corresponding Cd2+ treatment ((B)).
Figure 9. Changes in malondialdehyde ‘MDA’ content in maize roots and shoots. A: seedlings treated with different hematite NPs concentrations. B: seedlings treated with Cd2+ concentrations only or combined with 500 mg kg−1 hematite NPs. The values reported in the figure are means ± standard deviation of three replicates. Different letters on the bars (lower case for roots and uppercase for shoots) indicate significant differences among treatments as evaluated by Duncan's multiple comparison test (p < .05).
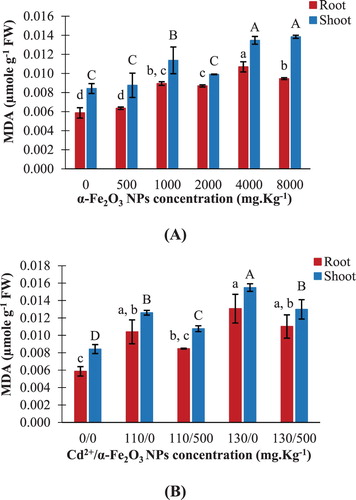
3.6. Antioxidant enzymes
In maize seedlings, it was noticed that exposure to 500 mg kg−1 hematite NPs led to insignificant changes in SOD activities in roots and shoots relevant to their control ((A)). On the contrary, seedlings grown in 4000 and 8000 mg kg−1 hematite NPs showed a significant increase in SOD activity in roots by about 114% and 50% respectively, compared to the control ((A)). Furthermore, the addition of 130 mg kg−1 Cd2+ to the soil led to increase in SOD activity by about 45% and 99% in maize roots and shoots respectively, compared to their corresponding controls ((E)). However, combined treatment of 500 mg kg−1 hematite NPs and 130 mg kg−1 Cd2+ significantly reduced SOD activity by about 46% and 31% in roots and shoots respectively, relevant to their corresponding Cd2+-treatment ((E)).
Figure 10. Changes in SOD, CAT, APX and GR activities in maize roots and shoots. A–D: seedlings treated with different hematite NPs concentrations. E–H: seedlings treated with Cd2+ concentrations only or combined with 500 mg kg−1 hematite NPs. The values reported in the figure are means ± standard deviation of three replicates. Different letters on the bars (lower case for roots and uppercase for shoots) indicate significant differences among treatments as evaluated by Duncan's multiple comparison test (p < .05).
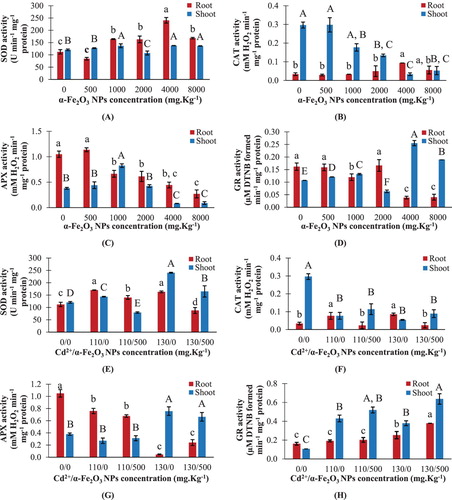
In response to hematite NPs addition, maize seedlings grown in 500 and 1000 mg kg−1 showed insignificant changes in CAT activities in both roots and shoots, compared to their corresponding controls ((B)). At 4000 mg kg−1 hematite NPs, CAT activity in roots was significantly increased by about ∼2 fold with respect to the control ((B)). However, exposing maize seedlings to 2000, 4000 and 8000 mg kg−1 NPs significantly reduced CAT activity in shoots by 55%, 89%, and 82%, respectively ((B)). It was noticed that CAT activity was elevated by about 154% in roots upon exposure to 130 mg kg−1 Cd2+, while a significant decrease in CAT activity by about 82% was detected in shoots upon the same level treatment, respective to their corresponding controls ((F)). Meanwhile, combined treatment of 500 mg kg−1 hematite NPs and 130 mg kg−1 Cd2+ significantly reduced CAT activity in roots by 71%, compared to the corresponding Cd2+-treatment ((F)). While shoots exposed to a mixture of 500 mg kg−1 hematite NPs with either with 110 or 130 mg kg−1 Cd2+ displayed insignificant changes in CAT activity relevant to their corresponding Cd2+ treatments ((F)).
The APX activities in both roots and shoots of maize showed a significant reduction upon 4000 and 8000 mg kg−1 hematite NPs treatments ((C)). However, the only significant elevation in APX activity among all hematite NPs treatments was noticed by about 117% in shoots grown in 1000 mg kg−1 NPs ((C)). Hematite NPs at 500 mg kg−1 led to insignificant changes in APX activities in both roots and shoots, respective to the control ((C)). On the other hand, maize seedlings grown in 130 mg kg−1 Cd2+ showed a significant reduction in APX activity in roots by about 96%, and a significant elevation in shoots by about ∼1 fold, compared to the control ((G)). Concerning the combined treatments of 500 mg kg−1 hematite NPs with either 110 or 130 mg kg−1 Cd2+, insignificant changes were observed in APX activities in roots and shoots of maize, compared to the corresponding Cd2+ treatments ((G)).
The exposure to hematite NPs at concentrations 1000, 4000 and 8000 mg kg−1 significantly reduced GR activity in roots, relative to the control, while insignificant changes in activity were detected in roots at 500 and 2000 mg kg−1 NPs ((D)). Regarding the activity in maize shoots, treatment with 500, 1000, 4000 and 8000 mg kg−1 hematite NPs led to a significant increase in GR activity by about 13%, 23%, 139%, and 78% respectively, in comparison to the control ((D)). Furthermore, exposure to 130 mg kg−1 Cd2+ led to significant elevation in GR activity in roots and shoots by about 57% and 254% over the control, respectively ((H)). Meanwhile, combined treatment of 500 mg kg−1 hematite NPs and 130 mg kg−1 Cd2+ significantly increased GR activity in roots and shoots by about 50% and 68% respectively, in comparison to the corresponding Cd2+-treated ones ((H)).
3.7. Gene expression studies
The expression levels of the SOD gene in roots and shoots showed insignificant changes in response to low doses of hematite NPs (500, 1000 and 2000 mg kg−1) ((A)). However, exposure to the highest NPs dose (8000 mg kg−1) resulted in significant up-regulation of SOD gene expression in roots by ∼5 fold over the control ((A)). The SOD gene expression levels were significantly positively correlated with the SOD enzyme activities in shoots, upon exposure to hematite NPs treatments (r = +0.472, p < .05), while the insignificant correlation was detected in roots (). It's remarkable that the expression levels of SOD significantly increased by about ∼12.5 and ∼4.1 fold over the control in roots and shoots respectively, upon exposure to 130 mg kg−1 Cd2+ ((C)). However, combined treatment of 500 mg kg−1 hematite NPs with either 110 or 130 mg kg−1 Cd2+ significantly declined the SOD expression levels in roots by about 83% and 75% respectively, compared to their corresponding Cd2+-treated groups ((C)). The SOD gene expression levels were significantly positively correlated with the SOD enzyme activities in roots under Cd2+ and combined treatments (r = +0.696, p < .01), while the insignificant correlation was noticed in shoots ().
Figure 11. Changes in relative mRNA expression of SOD and CAT genes in maize roots and shoots. A,B: seedlings treated with different hematite NPs concentrations. C,D: seedlings treated with Cd2+ concentrations only or combined with 500 mg kg−1 hematite NPs. The values reported in the figure are means ± standard deviation of three replicates. Different letters on the bars (lower case for roots and uppercase for shoots) indicate significant differences among treatments as evaluated by Duncan's multiple comparison test (p < .05).
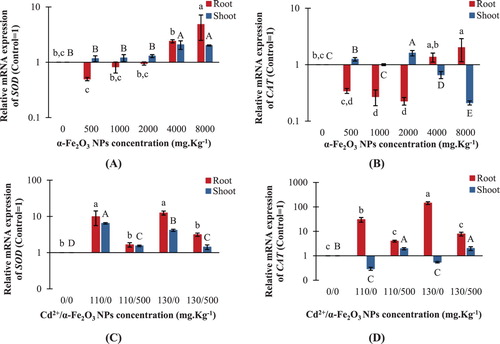
Table 2. Pearson correlation between relative mRNA expression levels and enzyme activities of superoxide dismutase (SOD) and catalase (CAT) in maize roots and shoots treated with different concentrations of hematite NPs (0, 500, 1000, 2000, 4000 and 8000 mg Kg−1).
Table 3. Pearson correlation between relative mRNA expression levels and enzyme activities of superoxide dismutase (SOD) and catalase (CAT) in maize roots and shoots exposed to 110 or 130 mg kg−1 Cd2+ and combined treatments of hematite NPs (500 mg kg−1) with the corresponding Cd2+ concentration.
It was noticed that CAT expression was significantly up-regulated in maize roots by ∼2 fold over the control upon exposure to the highest hematite NPs concentration, while CAT expression was insignificantly different from the control in roots treated with 500 and 4000 mg kg−1 NPs ((B)). For maize shoots, high doses of hematite NPs (4000 and 8000 mg kg−1) significantly reduced the CAT expression by about 34% and 79% respectively, compared to the control ((B)). The CAT expression levels were insignificantly correlated with the CAT activities in both roots and shoots upon exposure to hematite NPs treatments (). Interestingly, maize roots treated with 130 mg kg−1 Cd2+ showed the highest up regulation in CAT gene expression among all treatments by about ∼143.6 fold over the control ((D)). However, in roots, CAT expression significantly decreased by about 87% and 95% upon combined treatment of 500 mg kg−1 hematite NPs either with 110 or 130 mg kg−1 Cd2+ respectively, compared to their corresponding Cd2+ treatments ((D)). The CAT expression levels were significantly positively correlated with the enzyme activities in roots, upon exposure to Cd2+ and combined treatments (r = +0.694, p < .01), while the insignificant correlation was detected in shoots ().
4. Discussion
In the present study, TEM and XRD analyses confirmed that chemically pure hematite (α-Fe2O3) NPs could be synthesized. Hematite is a mineral occurring in the environment either naturally or via anthropogenic processes (Esmaeili et al. Citation2011), therefore the successfully synthesized NPs presented herein could be a reasonable representation of iron oxide NPs that may occur in the environment. The effect of hematite NPs (500–8000 mg kg−1), Cd2+ (110 or 130 mg kg−1) and combined treatments of hematite NPs (500 mg kg−1) with the corresponding Cd2+ level was carried out using Zea mays L. as a model plant. This study may help to better understand the effects of hematite NPs, suggesting a complex mode of action that was dependent on hematite NPs composition, concentration, and dissolved metallic ions.
It was observed in our study that lengths and dry weights of maize roots/shoots were significantly stimulated at the lowest concentration of hematite NPs (500 mg kg−1). Li et al. (Citation2018) assumed that iron oxide NPs could act as iron suppliers through releasing Fe in a form that could be taken up by plant roots. Moreover, iron is an essential microelement which serves as a potential nutrition source for plants; accordingly, controlled addition of hematite NPs possibly stimulated the growth of maize seedlings as well as biomass production. It was proposed by Ren et al. (Citation2011) that NPs might facilitate the opening of water channels in roots, and therefore stimulates better cell absorption to water, inorganic ions, and nutrients, and thus stimulates plant growth. On the other hand, high concentrations of hematite NPs were considered a major factor influencing their toxicity in maize seedlings. Herein, hematite NPs at concentrations 4000 and 8000 mg kg−1 demonstrated inhibitory effects on lengths and dry weights of maize roots/shoots. Metal oxide NPs at high concentrations were assumed to adsorb on seed surfaces due to electrostatic attraction and hydrophobic interactions between seeds and NPs agglomerates, and hence release metal ions which eventually enhanced NPs phytotoxicity (Wu et al. Citation2012). It was reported by Palchoudhury et al. (Citation2018) that low concentration (5.54 × 10−3 mg L−1) of iron oxide NPs significantly increased the root growth of Pisum sativum, Cicer arientinum, and Vigna radiata, while high concentration (27.7 mg L−1) reduced root growth of the treated plants. Pariona et al. (Citation2017) recorded that no signs of toxicity were observed upon treating maize seedlings with hematite NPs at concentrations 1, 2, 4 and 6 g.L−1. Therefore, the stimulatory or inhibitory effects of NPs on plant growth mainly depend on the NPs concentration as well as the plant species.
Growth parameters could be effectively used as potential criteria of Cd2+ toxicity in plants. Herein, Cd2+ (110 or 130 mg kg−1) resulted in a significant reduction in lengths and dry weights of maize seedlings, which could be attributed to the inhibition of cell division and elongation (Ahmad et al. Citation2011). However, in our study, the growth measurements of maize including lengths and dry weights were highly stimulated upon combined treatments of 500 mg kg−1 hematite NPs with either 110 or 130 mg kg−1 Cd2+, compared to the corresponding Cd2+ treatments. The markedly diminished Cd2+ toxicity in maize seedlings may be attributed to the loss of heavy metal phytoavailability through adsorption onto hematite NPs surface; the high reactivity and large specific surface area of NPs may help in decreasing the mobility and bioavailability of Cd2+, and hence resulting in their stabilization in the soil (Michálková et al. Citation2014). Moreover, in this study, reduced Cd2+ accumulation along with enhanced Fe supply was observed in maize roots upon combined treatment of 500 mg kg−1 hematite NPs and 130 mg kg−1 Cd2+, respective to the corresponding Cd2+ treatment, which probably reflected the elevation in maize growth. Rizwan et al. (Citation2019) and Wang et al. (Citation2012) suggested that iron oxide NPs had a great impact in reducing cadmium-induced phytotoxicity without showing a negative impact on the growth of different plant species such as wheat, tomato, cucumber, carrot, and lettuce.
The organic substances in the soil may influence the mobility of NPs, and hence favored their accumulation in plant root tissues (Chichiriccò and Poma Citation2015). In the current study, the ICP-OES analysis of maize roots revealed the internalization and accumulation of hematite NPs in the root cells. It is known that the pore diameter (5–20 nm) of the plant cell wall serves as a barrier against the easy entry of any external agents (Fleischer et al. Citation1999). Therefore, NPs or their aggregates could readily pass through the cell wall and reach the plasma membrane if they possess a diameter less than 20 nm (Navarro et al. Citation2008). However, in our study, hematite NPs possess a larger diameter (∼34 nm). Accordingly, NPs might have been taken up by cells through enlargement of existing pores, induction of new cell wall pores, internalization during endocytosis in the plasma membrane, or crossing the cell membrane with the help of transport carrier proteins or through ion channels (Nair et al. Citation2010). It was also suggested by Alidoust and Isoda (Citation2014) that the large surface area to mass ratio of iron oxide NPs might be an important phenomenon that affects the solubility and availability of iron in the soil, as NPs have a great potential to be adsorbed onto soil colloids, and thus enhance their uptake. The current findings were consistent with previous studies regarding a dose dependent increase in the amount of Fe in Solanum lycopersicum (Shankramma et al. Citation2016) and Arachis hypogaea (Rui et al. Citation2016) upon exposure to increasing concentrations of iron oxide NPs with average sizes 10 and 20 nm, respectively. However, Marusenko et al. (Citation2013) pointed out that Arabidopsis thaliana plants didn't show uptake of hematite NPs (∼40.9 nm) when grown in agar media, suggesting that the failure to obtain NPs may be a possible mechanism for plant survival against NPs toxicity. Furthermore, the current findings showed that the accumulation of hematite NPs in maize roots resulted in a significant reduction in calcium uptake at NPs treatments ranged from 2000 to 8000 mg kg−1. It was proposed that NPs aggregation on root surface might led to damage in the root cellular membranes and water transport system (Martínez-Fernández et al. Citation2016), and hence resulting in membrane leakage to ions and membrane ion-channels disturbance which eventually influenced nutrients uptake The disturbance in membrane function was strongly consistent with the development of lipid peroxidation in maize roots herein, upon exposure to 2000–8000 mg kg−1 of hematite NPs.
On other hand, an increase in the intracellular Cd2+ level was demonstrated in maize roots herein, upon exposure to 110 or 130 mg kg−1 Cd2+, confirming the uptake of Cd2+ from the amended soil. The exposure to both Cd2+ concentrations significantly declined Fe content in maize roots, possibly through interfering of Cd2+ with the metal transport system (Andresen and Küpper Citation2013) or due to increased membrane damage of root cells, and thus leading to inhibition of water and elements absorption or even leakage of elements out of the cells. In contrast, a significant elevation in Ca2+ content was detected herein in Cd2+-treated roots, respective to the control, which might be attributed to the importance of Ca2+ in maintaining cell wall and membrane stabilization under Cd2+ stress through providing intermolecular linkages, and subsequently reducing the toxic effects of Cd2+ (Jiang et al. Citation2004). Interestingly, combined treatment of 500 mg kg−1 hematite NPs and 130 mg kg−1 Cd2+ herein resulted in a significant diminution of Cd2+ content in maize roots, compared to the corresponding Cd2+-treated ones. This could be attributed to the significant increase in Fe accumulation in roots upon NPs addition, in which Fe possibly competed with Cd2+ on the root surface resulting in the reduction of Cd2+ uptake by roots. Moreover, hematite NPs amendment might reduce the Cd2+ amount per unit mass ‘dilution effect,’ through promoting length and weight of maize seedlings. The current results were in agreement with Rizwan et al. (Citation2019) who showed that treating wheat seedlings with iron oxide NPs reduced Cd2+ concentrations in plant roots, shoots and grains, respective to the control. However, these findings were contradicted with Gong et al. (Citation2017) who reported increased Cd2+ concentrations in Boehmeria nivea upon amendment with nanoscale zerovalent iron (nZVI). Moreover, our findings showed that exposure to combined treatments of hematite NPs and Cd2+ led to an improvement in the membrane structure and function of maize roots as supported by the TEM micrographs, which consequently increased the uptake of Fe and reduced Ca2+ content in roots, respective to Cd2+ treatments.
The ultra-structure micrographs in the current study revealed that black aggregates of NPs were accumulated inside the vacuoles of maize roots treated with the highest hematite NPs concentration (8000 mg kg−1). This was possibly due to excessive hematite uptake by maize roots which probably resulted in the development of NPs aggregates, suggesting developed Van der Waals forces between the particles due to their magnetic properties (Maity and Agrawal Citation2007). Moreover, high vacuolization was shown herein in 8000 mg kg−1 hematite NPs-treated roots, which indicated that vacuoles might have been the primary storage for accumulated NPs in maize roots, and hence decreasing their levels in the cytoplasm and nucleus. Since hydrolytic enzymes and a variety of defense proteins are considered main constituents of plant vacuoles (Hara-Nishimura and Hatsugai Citation2011), therefore the accumulation of hematite NPs within the vacuoles might function as a detoxifying mechanism against NPs stress. The current findings were consistent with Li et al. (Citation2016a) who showed the localization of iron oxide NPs within the vacuoles of Zea mays root cells. Furthermore, in the current study, the penetration of hematite NPs (8000 mg kg−1 of NPs) inside maize roots led to severe ultra-morphological perturbations including irregularly-shaped nuclei with fragmented and unequally distributed chromatin. It was suggested that chromatin condensation or fragmentation might be a part of the programed cell death (PCD) in plants which possibly plays a key role in mediating plant adaptation to the environmental stresses (Gladish et al. Citation2006). Accordingly, PCD might be an important step in activating DNA damage-repair signaling in response to NPs stress. However, it was still unclear whether the observed genotoxic effects in the studied maize roots were direct or indirect consequences of the treatments, since hematite NPs were not localized in the nucleus. Furthermore, an increase in cell wall thickness was detected in 8000 mg kg−1 hematite NPs-treated roots, which might be attributed to enhanced lignification of plant cell walls in response to NPs stress. Thus, enhanced cell wall rigidity, reduction in cell expansion and elongation and reduced minerals uptake by roots might have been developed in maize seedlings herein, leading to a significant reduction in root growth. Moreover, the TEM micrographs of 8000 mg kg−1 hematite NPs-treated roots showed an increase in the number of amyloplasts, suggesting a disruption of the photosynthesis in maize which probably reflected the accumulation of more amyloplasts in roots in response to high concentrations of hematite NPs.
According to the TEM micrographs in the current study, 130 mg kg−1 Cd2+ treatment induced the most severe ultra-morphological damage in maize root cells among all studied treatments, indicating Cd2+ toxicity in maize roots. The most detected variation included high vacuolization events accompanied with Cd2+ deposition within the vacuoles, which might be a detoxification mechanism preventing the circulation of free Cd2+ in the cytosol by forcing them into a limited area. Consequently, accumulation of Cd2+ within the vacuoles could be responsible for high Cd2+ content in maize roots herein upon 130 mg kg−1 Cd2+ level treatment, as supported by the ICP-OES analysis in the current study. These results were in agreement with Daud et al. (Citation2015) who figured out the accumulation of Cd2+ in the form of electron dense granules in the vacuoles of two transgenic cotton cultivars exposed to different Cd2+ doses. In addition, an increase in cell wall thickness was detected in 130 mg kg−1 Cd2+-treated roots herein, which might be considered as a defense mechanism, and thus diminishing the Cd2+-induced damage. Moreover, nuclei were present in the form of highly damaged and irregularly-shaped structures upon 130 mg kg−1 Cd2+, which possibly indicated high ROS production in maize seedlings resulting in DNA damage.
Interestingly, ultra-structure micrographs revealed that the root cell structure relatively retained its integrity upon combined treatment of 500 mg kg−1 hematite NPs and 130 mg kg−1 Cd2+, compared to the corresponding Cd2+ treatment. The TEM images of root cells showed normal size and distribution of mitochondria in roots exposed to combined treatment, compared to Cd2+-treated ones, which might be a mechanism to combat with Cd2+ toxicity through the production of a large amount of energy inside the cells. Though the only detectable damage signs in roots exposed to combined treatment were the slight increase in vacuoles size and number as well as an increase in cell wall thickness, compared to the control, which was still lower than the Cd2+-treated ones. Accordingly, it was concluded that amendment with hematite NPs (500 mg kg−1) probably exerted a positive effect on ameliorating Cd2+ toxicity in maize seedlings to a certain degree.
Nanoparticles were supposed to induce toxicity through the excessive generation of ROS, and therefore leading to oxidative stress in plants (Khan et al. Citation2017). In the current study, a significant elevation in MDA content was detected in maize roots and shoots upon exposure to the highest hematite NPs treatment (8000 mg kg−1). It was proposed that NPs-induced toxicity might be attributed to either the direct interaction of NPs with plants or as a result of NPs dissolution into toxic ions (Dietz and Herth Citation2011). Accordingly, free metal ions might participate in Fenton reaction leading to the generation of hydroxyl radicals, and hence developing membrane lipid peroxidation in plants (Li et al. Citation2018). Moreover, Wang et al. (Citation2011) suggested that an increase in lipid peroxidation in plant might be attributed to blockage of the aquaporins and disturbance of the respiration rate in the roots in response to NPs accumulation. On the other hand, insignificant changes in MDA content were detected in maize roots and shoots herein, upon exposure to the lowest hematite NPs concentration (500 mg kg−1). This might be attributed to the lack of membrane damage possibly due to either lower ROS production in cells or that the antioxidant defense system of maize seedlings was sufficient to eliminate the induced damage.
According to our findings, the exposure to Cd2+ (110 or 130 mg kg−1) resulted in severe oxidative stress in maize seedlings, as indicated by increased MDA content in maize roots and shoots, compared to control, which was probably due to enhanced ROS generation in cells. On the other hand, combined treatments of 500 mg kg−1 hematite NPs with either 110 or 130 mg kg−1 Cd2+ significantly reduced MDA content in maize shoots, respective to the corresponding Cd2+ treatments, suggesting that hematite NPs possibly protected the cell membranes from Cd2+-mediated oxidative stress. The positive effect of combined treatment was also confirmed by the significant enhancement in lengths and dry weights of maize shoots, respective to the corresponding Cd2+ treatments. These results were in agreement with Konate et al. (Citation2017) who pointed out a reduction in MDA content in Triticum aestivum seedlings upon amending iron oxide NPs with heavy metals.
Plants could develop different antioxidant defense systems in order to enhance their resistance to different contaminants, and thus counteract oxidative damage. SOD plays a vital role in the antioxidant defense system in plants by catalyzing highly toxic ROS to less toxic H2O2 and O2. Significant increase in SOD activity was reported in maize roots upon exposure to high hematite NPs concentrations (4000 and 8000 mg kg−1), suggesting that maize seedlings might have strengthened their antioxidant defense system in order to detoxify the elevated ROS levels. On the other hand, 500 mg kg−1 hematite NPs led to insignificant changes in SOD activity in maize roots and shoots, suggesting reduced ROS production and less oxidative stress, which was consistent with the insignificant changes observed in MDA content at the same level treatment. The current findings were consistent with Li et al. (Citation2016a) who found that no changes in SOD activities were detected in Zea mays roots upon exposure to 20–100 mg L−1 iron oxide NPs. In contrast, Li et al. (Citation2017) reported that SOD activities in Citrus reticulata leaves were decreased at lower iron oxide NPs concentration (20 mg L−1), while treatment with 50 and 100 mg L−1 NPs increased SOD activities compared to control.
Catalase is an essential antioxidant enzyme that plays a key role in the detoxification of ROS by converting H2O2 to H2O and O2. CAT activity in maize roots significantly increased at high hematite NPs concentration (4000 mg kg−1), indicating the detoxification of excessive H2O2 which possibly resulted from an increased accumulation of hematite NPs in cells. This was consistent with Li et al. (Citation2016a) who suggested the accumulation of H2O2 in Zea mays roots after treatment with 100 mg L−1 of iron oxide NPs, which eventually led to enhanced CAT activity. In contrast, Saquib et al. (Citation2016) pointed out that exposing Raphanus sativus to lower concentrations of iron oxide NPs enhanced CAT activity in roots, while a declining pattern of CAT activity was detected at higher concentrations. Furthermore, in the present study, exposure to 2000, 4000 and 8000 mg kg−1 hematite NPs significantly reduced CAT activity in maize shoots. This might be attributed to the increased supply of hematite NPs which possibly led to excessive ROS production, and subsequently exceeded the defense mechanism in maize shoots. Accordingly, inactivation of the enzyme protein, decrease in enzyme synthesis, or change in assembly of the enzyme subunits might have been developed in response to oxidative damage (Ghosh et al. Citation2016). This was contradicted with Rui et al. (Citation2016) who found that treating Arachis hypogaea with different concentrations of iron oxide NPs enhanced CAT activity in shoots.
Ascorbate peroxidase catalyzes the detoxification of H2O2 using ascorbic acid as a reducing agent with generation of H2O. The obtained results revealed that APX activity in maize roots showed a significant reduction upon treatment with 4000 mg kg−1 hematite NPs, respective to the control. It was suggested that APX and CAT enzymes could function simultaneously in different parts of the plants in order to break down H2O2 (Słomka et al. Citation2008). Therefore, the reduction in APX activity in maize roots herein was parallel with the increase in CAT activity upon exposure to 4000 mg Kg−1 hematite NPs, and accordingly, enhanced CAT activity might be considered as a key point for the decomposition of H2O2 in maize roots; especially under APX inactivation. These results were contradicted with Trujillo-Reyes et al. (Citation2014) who pointed out an increase in APX activity in roots of Lactuca sativa following the treatment with iron oxide NPs. Furthermore, in the current study, maize shoots grown in 1000 mg kg−1 hematite NPs exhibited a significant elevation in APX activity, while a significant diminution in activity was noticed in shoots upon treatment with the highest NPs concentration (8000 mg Kg−1). It was suggested that this reversal may be due to that maize seedlings initially strengthened their antioxidant defense system in shoots to deal with the oxidative damage, but then lost their capability to control APX activity when the ROS burst and the enzyme structure became disrupted.
Glutathione reductase is a NADPH-dependent enzyme which catalyzes the reduction of oxidized glutathione (GSSG) to reduced glutathione (GSH), and thus sustaining the reduced status of GSH. In the current study, significant increase in GR activity was observed in shoots upon treatment with 500, 1000, 4000 and 8000 mg kg−1 hematite NPs, relative to the control. The increase in GR activity under hematite NPs application was not observed in linear or even regular trend. It is well known that the role of GR in H2O2 scavenging in plants has been well established in ascorbate glutathione (AsA-GSH) cycle (Gill and Tuteja Citation2010). Accordingly, the observed increase in GR activity in shoots could result in the availability of NADP+, which could accept electrons from ferredoxin, thereby minimizing chances of superoxide formation in addition to scavenging of H2O2 and maintained photosystem II efficiency (Arora et al. Citation2002). Thus, GR might be important in fighting oxidative stress in maize shoots under hematite NPs treatments. In contrast, concerning the GR activity in maize roots herein, a significant reduction was observed upon exposure to 1000, 4000 and 8000 mg kg−1 hematite NPs, which could be attributed to direct binding of NPs to sulfhydryl group of the enzyme in roots, and hence influencing the glutathione cycle.
In the present study, Cd2+ (130 mg kg−1) toxicity significantly increased SOD activity in roots and shoots, compared to the control. It was suggested that maize seedlings might have strengthened the antioxidant defense system in order to sequester Cd2+-induced damage. However, when the stress of Cd2+ exceeded the self-protection capacity of plant, the excessive free radicals could not be removed. This was the reason behind the increase in membrane lipid peroxidation herein, even at enhanced SOD activity in Cd2+-treated seedlings. On the other hand, combined treatment of 500 mg Kg−1 hematite NPs and 130 mg Kg−1 Cd2+ significantly reduced SOD activities in maize roots and shoots, relevant to the corresponding Cd2+ treatment. In spite that the decline in SOD activity might reveal that the antioxidant defense system was compromised. Though, the decrease in SOD activity was not correlated with growth inhibition under the interaction between hematite NPs and Cd2+. Moreover, the membrane lipid peroxidation in the present study was decreased in combined treatment of 500 mg Kg−1 hematite NPs and 130 mg Kg−1 Cd2+ in maize shoots, respective to Cd2+ treatment. Accordingly, the presence of hematite NPs might reduce Cd2+ toxicity through the mitigation of membrane damage. Overall, the existence of hematite NPs might not change the mechanism of Cd2+ toxicity, while it decreased Cd2+ accumulation in maize and further decreased the toxicity of Cd2+.
According to the current findings, 130 mg kg−1 Cd2+ significantly elevated CAT activity in roots, while in shoots a significant reduction was observed. Meanwhile, combined treatment of hematite NPs and 130 mg kg−1 Cd2+ significantly reduced CAT activity in roots compared to the corresponding Cd2+-treated ones, suggesting that other pathways for detoxification and scavenging of H2O2 might have been involved. On the other hand, APX activity was significantly reduced in maize roots and increased in shoots upon exposure to 130 mg kg−1 Cd2+. The former effect was probably due to the higher levels of H2O2 formed in roots under Cd2+ stress which became inhibitory to APX. Moreover, the sequestering of Cd2+ in roots (as supported by the ICP-OES and TEM analysis) possibly led to a fewer passage of Cd2+ to shoots which acted as a stimulant to APX activity in order to counteract ROS-mediated damage in shoots. Consistently, both APX and CAT enzymes could work in a parallel mechanism to scavenge H2O2, and thus the decrease in the activity of one of them might be compensated by the activation of the other.
Exposure to 130 mg kg−1 cadmium and combined treatment of 500 mg kg−1 hematite NPs with the same Cd2+ level, significantly increased GR activity in maize roots and shoots. This might reflect the maintenance of GSH level involved in the antioxidant defense mechanism, probably through either the efficient functioning of GR by regulating its biosynthesis or through protecting the reactive cysteine residue of GSH (Foyer and Noctor Citation2005).
In conclusion, exposing maize seedlings to combined treatments of hematite NPs and Cd2+ significantly stimulated activities of enzymes such as GR in some cases, or significantly reduced activities of enzymes such as SOD and CAT. Therefore, it has to be concluded that stimulated antioxidant enzymes activity might not be the main reason for hematite NPs-induced alleviation of Cd2+ toxicity in all treatments. Exploitation or activation of any of these enzymes or biomolecules may depend on plant species, the level of their metal tolerance, plant growth stage and metal type.
Under NPs stress, the increase in intracellular levels of ROS and peroxidation products were supposed to trigger various defense systems through inducing/suppressing the expression of many genes such as oxidative stress related genes (Singh et al. Citation2017). According to the current study, exposure to the highest NPs dose (8000 mg kg−1) significantly up-regulated SOD and CAT gene expression in roots. This could be attributed to the development of oxidative stress probably through enhanced ROS generation, and therefore a protective scavenging system of ROS was possibly induced in order to counteract NPs-induced oxidative damage. These findings were consistent with Li et al. (Citation2018) who reported that the SOD gene was up-regulated in roots of Citrus maxima seedlings in response iron oxide NPs. On the other hand, maize shoots herein showed significant down regulation of CAT expression, following the exposure to high doses of hematite NPs (4000 and 8000 mg kg−1), which consequently might have been resulted in inadequate scavenging of excess ROS leading to lipid peroxidation in maize shoots.
Cadmium toxicity (130 mg kg−1) led to significant up-regulation of SOD and CAT gene expression in maize roots. This possibly indicated an excess ROS production in maize cells, which eventually led to an activated ROS defense system. On the other hand, the addition of hematite NPs (500 mg kg−1) to soil amended with 110 or 130 mg kg−1 Cd2+ resulted in a significant reduction in SOD and CAT gene expression in maize roots, compared to the corresponding Cd2+-treated groups. This might suggest the positive effect of hematite NPs in ameliorating Cd2+-induced stress in maize seedlings, which possibly led to less oxidative stress in combined treatments, respective to Cd2+ treatments. The positive effect of hematite NPs was consistent with improved maize growth and lower lipid peroxidation levels upon combined treatments, respective to Cd2+-treatments.
Taken together, in shoots, SOD gene expression levels were significantly positively correlated with their corresponding enzyme activities upon hematite NPs treatments, while in roots both SOD and CAT gene expression levels were significantly positively correlated with their corresponding enzyme activities upon Cd2+, or combined treatments. Several genes coding for enzymatic defense mechanisms are activated in different cell compartments such as mitochondria, chloroplasts, peroxisomes, apoplasts, and the cytosol in order to protect cells from oxidative stress (Fridovich Citation1986). Since, RT–PCR provided data about specific gene expression, therefore, it is possible to understand that the up/down-regulation of a certain gene might not reflect the whole antioxidant state of the plant. Moreover, the changes in enzyme activity might not possibly be influenced by gene expression (mRNA levels), suggesting that the post-transcriptional regulation might play a role in the activation of enzyme activity under NPs exposure (Gupta et al. Citation2018). The regulation of gene expression in plants under NPs is a complex mechanism that is mainly influenced by NPs type, concentration as well as the plant species.
5. Conclusions
In summary, it was hypothesized from our findings that hematite NPs (500 mg kg−1), as an iron source, could be applied as nanofertilizer in maize from the aspect of nutrient supplement, though high concentrations of hematite NPs (4000–8000 mg kg−1) induced inhibitory effects on maize growth. Furthermore, it was found that in certain conditions amending hematite NPs (500 mg kg−1) with Cd2+ (110 or 130 mg kg−1) modulated the cadmium-induced toxicity to a certain degree. Hence, this study could further fill up the gaps of hematite NPs use in alleviating Cd2+ toxicity in maize seedlings through enhancing the plant growth and Fe concentrations while reducing Cd2+ accumulation in plant tissues. Hematite NPs, owing to their nanoscale size and large surface area, could easily penetrate into the contamination zone, and thus possess a strong affinity for metal adsorption. Accordingly, this study highlights the importance of hematite NPs on maize growth and could provide new insights in predicting maize performance under Cd2+ stress which is a commonly occurring phenomenon in agricultural systems.
6. Future perspectives
Recent studies have shown that NPs could be implicated in different fields such as agriculture, environmental remediation, catalysis, medicine and the protection of plants against heavy metals-induced toxicity, however, publications concerning the role of hematite NPs are still limited. Therefore, this study may help to better understand the hematite NPs-plant interactions. Though, further research is essential to evaluate the effect of different characteristics of hematite NPs on plant growth; this includes NPs charge, hydrophobicity, coating agents, etc. Also, widespread field studies and long-term exposure scenarios could be useful to obtain full investigation about the whole life cycle of plant as a consequence of hematite NPs and heavy metal exposure, which might be useful for agricultural crop yield and food safety. Furthermore, detailed insight on the interaction of hematite NPs with soil rhizosphere is required in order to detect their possible dissolution into metal ions and their subsequent transportation through the shoot system with a consequent impact on human health.
Acknowledgements
Ola Youssef carried out the experiments, processed the samples and performed the biochemical and the statistical analysis, interpreted the results, wrote and revised the manuscript. Amel Tammam designed the research, supervised biochemical determinations, interpreted the results, wrote and revised the manuscript. Ranya F. El-Bakatoushi revised the data of molecular biology techniques and interpreted the molecular biology results. Asmaa ِِAlframawy and Ola Youssef performed the molecular biology experiments. Mahmoud Emara suggested the type of NPs used in this work and provided critical guidance in characterization methods and preparation procedure of NPs. Laila El-Sadek carried out supervision guidance and gave critical comments.
Disclosure statement
No potential conflict of interest was reported by the author(s).
Correction Statement
This article has been corrected with minor changes. These changes do not impact the academic content of the article.
Additional information
Notes on contributors
Ola A. Youssef
Ola A. Youssef is an Assistant Lecturer, Section Cytology and Genetics, Botany and Microbiology Department, Faculty of Science, Alexandria University, Egypt. In 2013, she graduated in the Faculty of Science, Alexandria University with Bachelor’s Degree in Major Botany / Minor Chemistry, with a cumulative degree 3.9/4 (Excellent with Honor). She won the Ideal student award Class 2013 from Faculty of Science, Alexandria University. After that, in 2019, Ola accomplished her Master’s Degree in Plant Cytology and Genetics from Botany and Microbiology Department, Faculty of Science, Alexandria University. She had a good experience in teaching practical techniques for undergraduates in General Botany, Plant Cell Biology, Principles of Genetics, and Plant Biotechnology. Her research interests and innovative skills are mainly focused on plant cell biology, nanotechnology, and PCR based techniques.
Amel A. Tammam
Amel A. Tammam is a Professor of Molecular Plant Physiology in the Department of Botany and Microbiology, Faculty of Science, Alexandria University, Egypt. She obtained her Bachelor’sDegree in Botany (1989) and her Ph.D. (2001) from Faculty of Science, Alexandria University. She was promoted to Full Professor in 2012. Current research in the lab emphasizes on the use of physiological, ultrastructural and molecular techniques to: 1) study the mechanism of plant hormone action (e.g. gibberellin, auxin, abscissic acid, salicylic acid and indole butyric acid) in stress tolerance; 2) determine the action of allelochemicals in defense system for biological control as a natural herbicide; 3) evaluate salt tolerance in aquatic fern Azolla caroliniana, as bio fertilizer; 4) assess the influence of AMF inoculum on P transport, the evolution of H+-ATPases and its implications for tolerance in calcareous soil; 5) evaluate the chemical and biological amendments in improving phytoremediation of heavy metal contaminated soil; 6) study role of nanoparticles in mitigation of abiotic stress; and 7) study role of vermicompos for waste management in conferring salt stresstolerance.
Ranya F. El-Bakatoushi
Dr. Ranya F. El-Bakatoushi holds a PhD in Genetics from the University of Newcastle upon Tyne, UK. She is a Professor in Genetics and the head of biological and Geological Sciences department of Alexandria University, Egypt. Her research goal is to increase understanding of biological patterns and processes that shaped plant biodiversity. She has broad interests in different taxa to understand how speciation and phenotypic variation have occurred over different evolutionary time scale. Her research focuses on population genetics, functional genomics in plants. She also has interest in plant pollution interaction.
Asmaa M. Alframawy
Asmaa M. Alframawy is an Associate Professor of molecular genetics in Department of Nucleic acids Research, member of Genetic Engineering and Biotechnology Research Institute, City of Scientific Research and Technology Applications. Her PhD was in 2007 from Faculty of Agricultural, Department of Genetics, Alexandria University. Her research interests include biotic and a biotic stress in plants and related genes. Dr Asmaa focused her research on gene expression in plants and phylogenetic relationship in plants. Her research in these areas has been published in numerous journals and featured in GENETICA, Flora, International journal of phytoremediation, Life Science, Journal of Pure and Applied Microbiology, and many other national and international periodicals.
Mahmoud M. Emara
Mahmoud M. Emara is an assistant professor of physical chemistry in the Chemistry Department, Faculty of Science, Alexandria University, Egypt. In 1995, he graduated in the Faculty of Science, Alexandria University with special bachelor’s degree in chemistry. In 1999, he graduated in the Faculty of pharmacy, Alexandria University with bachelor’s degree in pharmaceutical sciences. In 1998, he was hired as teaching assistant in the Chemistry Department, Faculty of Science, Alexandria University, then he moved in 2003 to the USA to earn Ph.D. degree in physical chemistry in Ohio University, USA in 2008. His Ph.D. was about directed self-assembly of semiconductor nanoparticles for the purpose of reducing the power threshold for their optical gain (lasing). In 2010, he was hired an affiliated advisor in the Center of Excellence of Nano-manufacturing Applications (CENA), a consortium between Intel Corporation and King Abdul Aziz City of Science and Technology (KACST). His research work has been focused on tailoring inorganic semiconductor nanocomposites and applying them in catalysis, photocatalysis, and energy storage.
Laila M. El-Sadek
Laila M. El-Sadek holds a PhD in genetics from the University of Saskatchewan - Canada and assistant professor and full professor of genetics and cytology in botany and microbiology department of Faculty of Science, Alexandria University, Egypt. Also held the post of Head of Botany and Microbiology Department for 3 years. I am now an Emeritus professor in the same Department. My interest is the effect of pollution on economic plants, genetic diversity, population genetics and resolving problems of taxonomic nature through molecular techniques.
References
- Ahmad P, Nabi G, Ashraf M. 2011. Cadmium-induced oxidative damage in mustard [Brassica juncea (L.) Czern. & Coss.] plants can be alleviated by salicylic acid. S Afr J Bot. 77:36–44. doi: 10.1016/j.sajb.2010.05.003
- Ahmmad B, Leonard K, Shariful Islam M, Kurawaki J, Muruganandham M, Ohkubo T, Kuroda Y. 2013. Green synthesis of mesoporous hematite (α-Fe2O3) nanoparticles and their photocatalytic activity. Adv Powder Technol. 24:160–167. doi: 10.1016/j.apt.2012.04.005
- Alidoust D, Isoda A. 2014. Phytotoxicity assessment of γ-Fe2O3 nanoparticles on root elongation and growth of rice plant. Environ Earth Sci. 71:5173–5182. doi: 10.1007/s12665-013-2920-z
- Andresen E, Küpper H. 2013. Cadmium toxicity in plants. In: Sigel A., Sigel H., Sigel R. K. O, editor. Cadmium: from toxicity to essentiality. Dordrecht: Springer; p. 395–413.
- Arora A, Sairam RK, Srivastava GC. 2002. Oxidative stress and antioxidative system in plants. Curr Sci. 82:1227–1238.
- Ashokkumar T, Prabhu D, Geetha R, Govindaraju K, Manikandan R, Arulvasu C, Singaravelu G. 2014. Apoptosis in liver cancer (HepG2) cells induced by functionalized gold nanoparticles. Colloids Surf B: Biointerfaces. 123:549–556. doi: 10.1016/j.colsurfb.2014.09.051
- Beyer WF, Fridovich I. 1987. Assaying for superoxide dismutase activity: some large consequences of minor changes in conditions. Anal Biochem. 161:559–566. doi: 10.1016/0003-2697(87)90489-1
- Bradford MM. 1976. A rapid and sensitive method for the quantitation of microgram quantities of protein utilizing the principle of protein-dye binding. Anal Biochem. 72:248–254. doi: 10.1016/0003-2697(76)90527-3
- Buzea C, Pacheco II, Robbie K. 2007. Nanomaterials and nanoparticles: Sources and toxicity. Biointerphases. 2:17–71. doi: 10.1116/1.2815690
- Chichiriccò G, Poma A. 2015. Penetration and toxicity of nanomaterials in higher plants. Nanomaterials. 5:851–873. doi: 10.3390/nano5020851
- Choudhury S, Panda P, Sahoo L, Panda SK. 2013. Reactive oxygen species signaling in plants under abiotic stress. Plant Signal Behavior. 8:1–6.
- Daud MK, Quiling H, Lei M, Ali B, Zhu SJ. 2015. Ultrastructural, metabolic and proteomic changes in leaves of upland cotton in response to cadmium stress. Chemosphere. 120:309–320. doi: 10.1016/j.chemosphere.2014.07.060
- Dietz K-J, Herth S. 2011. Plant nanotoxicology. Trends Plant Sci. 16:582–589. doi: 10.1016/j.tplants.2011.08.003
- Esmaeili E, Salavati-Niasari M, Mohandes F, Davar F, Seyghalkar H. 2011. Modified single-phase hematite nanoparticles via a facile approach for large-scale synthesis. Chem Eng J. 170:278–285. doi: 10.1016/j.cej.2011.03.010
- Fleischer A, O’neill MA, Ehwald R. 1999. The pore size of non-graminaceous plant cell walls is rapidly decreased by borate ester cross-linking of the pectic polysaccharide rhamnogalacturonan II. Plant Physiol. 121:829–838. doi: 10.1104/pp.121.3.829
- Foyer CH, Noctor G. 2005. Redox homeostasis and antioxidant signaling: a metabolic interface between stress perception and physiological responses. Plant Cell. 17:1866–1875. doi: 10.1105/tpc.105.033589
- Fridovich I. 1986. Superoxide dismutases. In: Meister A, editor. Advances in enzymology and related areas of molecular biology. New York: John Wiley & Sons; p. 61–97.
- Ghosh M, Jana A, Sinha S, Jothiramajayam M, Nag A, Chakraborty A, Mukherjee A, Mukherjee A. 2016. Effects of ZnO nanoparticles in plants: cytotoxicity, genotoxicity, deregulation of antioxidant defenses, and cell-cycle arrest. Mutation Res/Genetic Toxicol Environ Mutagenesis. 807:25–32. doi: 10.1016/j.mrgentox.2016.07.006
- Gill SS, Tuteja N. 2010. Reactive oxygen species and antioxidant machinery in abiotic stress tolerance in crop plants. Plant Physiol Biochem. 48:909–930. doi: 10.1016/j.plaphy.2010.08.016
- Gladish DK, Xu J, Niki T. 2006. Apoptosis-like programmed cell death occurs in procambium and ground meristem of pea (Pisum sativum) root tips exposed to sudden flooding. Ann Bot. 97:895–902. doi: 10.1093/aob/mcl040
- Gong X, Huang D, Liu Y, Zeng G, Wang R, Wan J, Zhang C, Cheng M, Qin X, Xue W. 2017. Stabilized nanoscale zerovalent iron mediated cadmium accumulation and oxidative damage of Boehmeria nivea (L.) Gaudich cultivated in cadmium contaminated sediments. Environ Sci Technol. 51:11308–11316. doi: 10.1021/acs.est.7b03164
- Gossett DR, Millhollon EP, Lucas M. 1994. Antioxidant response to NaCl stress in salt-tolerant and salt-sensitive cultivars of cotton. Crop Sci. 34:706–714. doi: 10.2135/cropsci1994.0011183X003400030020x
- Gupta SD, Agarwal A, Pradhan S. 2018. Phytostimulatory effect of silver nanoparticles (AgNPs) on rice seedling growth: An insight from antioxidative enzyme activities and gene expression patterns. Ecotoxicol Environ Saf. 161:624–633. doi: 10.1016/j.ecoenv.2018.06.023
- Hara-Nishimura I, Hatsugai N. 2011. The role of vacuole in plant cell death. Cell Death Differ. 18:1298–1304. doi: 10.1038/cdd.2011.70
- Heath RL, Packer L. 1968. Photoperoxidation in isolated chloroplasts: I. Kinetics and stoichiometry of fatty acid peroxidation. Arch Biochem Biophys. 125:189–198. doi: 10.1016/0003-9861(68)90654-1
- Hu J, Guo H, Li J, Wang Y, Xiao L, Xing B. 2017. Intracellular trafficking and cellular uptake mechanism of PHBV nanoparticles for targeted delivery in epithelial cell lines. J Nanobiotechnol. 15:1–12. doi: 10.1186/s12951-017-0286-1
- Jiang XJ, Luo YM, Liu Q, Liu SL, Zhao QG. 2004. Effects of cadmium on nutrient uptake and translocation by Indian Mustard. Environ Geochem Health. 26:319–324. doi: 10.1023/B:EGAH.0000039596.15586.b3
- Kailasam K, Epping JD, Thomas A, Losse S, Junge H. 2011. Mesoporous carbon nitride–silica composites by a combined sol–gel/thermal condensation approach and their application as photocatalysts. Energy Environ Sci. 4:4668–4674. doi: 10.1039/c1ee02165f
- Kang YS, Risbud S, Rabolt JF, Stroeve P. 1996. Synthesis and characterization of nanometer-size Fe3O4 and γ-Fe2O3 particles. Chem Mater. 8:2209–2211. doi: 10.1021/cm960157j
- Kean EG, Hamaker BR, Ferruzzi MG. 2008. Carotenoid bioaccessibility from whole grain and degermed maize meal products. J Agric Food Chem. 56:9918–9926. doi: 10.1021/jf8018613
- Khan MN, Mobin M, Abbas ZK, Almutairi KA, Siddiqui ZH. 2017. Role of nanomaterials in plants under challenging environments. Plant Physiol Biochem. 110:194–209. doi: 10.1016/j.plaphy.2016.05.038
- Kimbrough DE, Wakakuwa JR. 1989. Acid digestion for sediments, sludges, soils, and solid wastes: a proposed alternative to EPA SW 846 method 3050. Environ Sci Technol. 23:898–900. doi: 10.1021/es00065a021
- Konate A, He X, Zhang Z, Ma Y, Zhang P, Alugongo GM, Rui Y. 2017. Magnetic (Fe3O4) nanoparticles reduce heavy metals uptake and mitigate their toxicity in wheat seedling. Sustainability. 9:790. doi: 10.3390/su9050790
- Li S, Chen J, Islam E, Wang Y, Wu J, Ye Z, Yan W, Peng D, Liu D. 2016b. Cadmium-induced oxidative stress, response of antioxidants and detection of intracellular cadmium in organs of moso bamboo (Phyllostachys pubescens) seedlings. Chemosphere. 153:107–114. doi: 10.1016/j.chemosphere.2016.02.062
- Li J, Hu J, Ma C, Wang Y, Wu C, Huang J, Xing B. 2016a. Uptake, translocation and physiological effects of magnetic iron oxide (γ-Fe2O3) nanoparticles in corn (Zea mays L.). Chemosphere. 159:326–334. doi: 10.1016/j.chemosphere.2016.05.083
- Li J, Hu J, Xiao L, Gan Q, Wang Y. 2017. Physiological effects and fluorescence labeling of magnetic iron oxide nanoparticles on citrus (Citrus reticulata) seedlings. Water, Air, Soil Pollut. 228:1–9. doi: 10.1007/s11270-016-3178-3
- Li J, Hu J, Xiao L, Wang Y, Wang X. 2018. Interaction mechanisms between α-Fe2O3, γ-Fe2O3 and Fe3O4 nanoparticles and citrus maxima seedlings. Sci Total Environ. 625:677–685. doi: 10.1016/j.scitotenv.2017.12.276
- Livak KJ, Schmittgen TD. 2001. Analysis of relative gene expression data using real-time quantitative PCR and the 2−ΔΔCT method. Methods. 25:402–408. doi: 10.1006/meth.2001.1262
- Maity D, Agrawal D. 2007. Synthesis of iron oxide nanoparticles under oxidizing environment and their stabilization in aqueous and non-aqueous media. J Magn Magn Mater. 308:46–55. doi: 10.1016/j.jmmm.2006.05.001
- Martínez-Fernández D, Barroso D, Komárek M. 2016. Root water transport of Helianthus annuus L. under iron oxide nanoparticle exposure. Environ Sci Pollut Res. 23:1732–1741. doi: 10.1007/s11356-015-5423-5
- Marusenko Y, Shipp J, Hamilton GA, Morgan JLL, Keebaugh M, Hill H, Dutta A, Zhuo X, Upadhyay N, Hutchings J, et al. 2013. Bioavailability of nanoparticulate hematite to Arabidopsis thaliana. Environ Pollut. 174:150–156. doi: 10.1016/j.envpol.2012.11.020
- Michálková Z, Komárek M, Šillerová H, Della Puppa L, Joussein E, Bordas F, Vaněk A, Vaněk O, Ettler V. 2014. Evaluating the potential of three Fe-and Mn-(nano) oxides for the stabilization of Cd, Cu and Pb in contaminated soils. J Environ Manag. 146:226–234. doi: 10.1016/j.jenvman.2014.08.004
- Miyagawa Y, Tamoi M, Shigeoka S. 2000. Evaluation of the defense system in chloroplasts to photooxidative stress caused by paraquat using transgenic tobacco plants expressing catalase from Escherichia coli. Plant Cell Physiol. 41:311–320. doi: 10.1093/pcp/41.3.311
- Nair PMG, Chung IM. 2015. Changes in the growth, redox status and expression of oxidative stress related genes in chickpea (Cicer arietinum L.) in response to copper oxide nanoparticle exposure. J Plant Growth Regul. 34:350–361. doi: 10.1007/s00344-014-9468-3
- Nair PMG, Chung IM. 2014. Cell cycle and mismatch repair genes as potential biomarkers in Arabidopsis thaliana seedlings exposed to silver nanoparticles. B Environ Contam Tox. 92:719–725. doi: 10.1007/s00128-014-1254-1
- Nair R, Varghese SH, Nair BG, Maekawa T, Yoshida Y, Kumar DS. 2010. Nanoparticulate material delivery to plants. Plant Sci. 179:154–163. doi: 10.1016/j.plantsci.2010.04.012
- Nakano Y, Asada K. 1981. Hydrogen peroxide is scavenged by ascorbate-specific peroxidase in spinach chloroplasts. Plant Cell Physiol. 22:867–880.
- Navarro E, Baun A, Behra R, Hartmann NB, Filser J, Miao A-J, Quigg A, Santschi PH, Sigg L. 2008. Environmental behavior and ecotoxicity of engineered nanoparticles to algae, plants, and fungi. Ecotoxicology. 17:372–386. doi: 10.1007/s10646-008-0214-0
- Palchoudhury S, Jungjohann KL, Weerasena L, Arabshahi A, Gharge U, Albattah A, Miller J, Patel K, Holler RA. 2018. Enhanced legume root growth with pre-soaking in α-Fe2O3 nanoparticle fertilizer. Royal Soc Chem Adv. 8:24075–24083.
- Pariona N, Martinez AI, Hdz-García HM, Cruz LA, Hernandez-Valdes A. 2017. Effects of hematite and ferrihydrite nanoparticles on germination and growth of maize seedlings. Saudi J Biol Sci. 24:1547–1554. doi: 10.1016/j.sjbs.2016.06.004
- Peternele WS, Fuentes VM, Fascineli ML, Da Silva JR, Silva RC, Lucci CM, De Azevedo RB. 2014. Experimental investigation of the coprecipitation method: An approach to obtain magnetite and maghemite nanoparticles with improved properties. J Nanomater. 2014:1–10. doi: 10.1155/2014/682985
- Ren H-X, Liu L, Liu C, He S-Y, Huang J, Li J-L, Zhang Y, Huang X-J, Gu N. 2011. Physiological investigation of magnetic iron oxide nanoparticles towards Chinese mung bean. J Biomed Nanotechnol. 7:677–684. doi: 10.1166/jbn.2011.1338
- Reynolds ES. 1963. The use of lead citrate at high pH as an electron-opaque stain in electron microscopy. J Cell Biol. 17:208–212. doi: 10.1083/jcb.17.1.208
- Rizwan M, Ali S, Ali B, Adrees M, Arshad M, Hussain A, Zia-Ur-Rehman M, Waris AA. 2019. Zinc and iron oxide nanoparticles improved the plant growth and reduced the oxidative stress and cadmium concentration in wheat. Chemosphere. 214:269–277. doi: 10.1016/j.chemosphere.2018.09.120
- Rui M, Ma C, Hao Y, Guo J, Rui Y, Tang X, Zhao Q, Fan X, Zhang Z, Hou T, Zhu S. 2016. Iron oxide nanoparticles as a potential iron fertilizer for peanut (Arachis hypogaea). Front Plant Sci. 7:1–10. doi: 10.3389/fpls.2016.00815
- Saquib Q, Faisal M, Alatar AA, Al-Khedhairy AA, Ahmed M, Ansari SM, Alwathnani HA, Okla MK, Dwivedi S, Musarrat J, et al. 2016. Genotoxicity of ferric oxide nanoparticles in Raphanus sativus: deciphering the role of signaling factors, oxidative stress and cell death. J Environ Sci. 47:49–62. doi: 10.1016/j.jes.2015.12.037
- Sebastian A, Nangia A, Prasad MNV. 2018. A green synthetic route to phenolics fabricated magnetite nanoparticles from coconut husk extract: implications to treat metal contaminated water and heavy metal stress in Oryza sativa L. J Cleaner Prod. 174:355–366. doi: 10.1016/j.jclepro.2017.10.343
- Shankramma K, Yallappa S, Shivanna MB, Manjanna J. 2016. Fe2O3 magnetic nanoparticles to enhance S. lycopersicum (tomato) plant growth and their biomineralization. Appl Nanosci. 6:983–990. doi: 10.1007/s13204-015-0510-y
- Singh S, Vishwakarma K, Singh S, Sharma S, Dubey NK, Singh VK, Liu S, Tripathi DK, Chauhan DK. 2017. Understanding the plant and nanoparticle interface at transcriptomic and proteomic level: A concentric overview. Plant Gene. 11:265–272. doi: 10.1016/j.plgene.2017.03.006
- Słomka A, Libik-Konieczny M, Kuta E, Miszalski Z. 2008. Metalliferous and non-metalliferous populations of Viola tricolor represent similar mode of antioxidative response. J Plant Physiol. 165:1610–1619. doi: 10.1016/j.jplph.2007.11.004
- Smith IK, Vierheller TL, Thorne CA. 1988. Assay of glutathione reductase in crude tissue homogenates using 5,5′-dithiobis(2-nitrobenzoic acid). Anal Biochem. 175:408–413. doi: 10.1016/0003-2697(88)90564-7
- Sokal RR, Rohlf FJ. 2013. Biometry: the principles and practice of statistics in biological research. In: Correa J, editor. New York: W.H. Freeman and Company; p. 177–602.
- Trujillo-Reyes J, Majumdar S, Botez CE, Peralta-Videa JR, Gardea-Torresdey JL. 2014. Exposure studies of core–shell Fe/Fe3O4 and Cu/CuO NPs to lettuce (Lactuca sativa) plants: Are they a potential physiological and nutritional hazard? J Hazard Mater. 267:255–263. doi: 10.1016/j.jhazmat.2013.11.067
- Wang M, Chen L, Chen S, Ma Y. 2012. Alleviation of cadmium-induced root growth inhibition in crop seedlings by nanoparticles. Ecotoxicol Environ Saf. 79:48–54. doi: 10.1016/j.ecoenv.2011.11.044
- Wang Y, Hu J, Dai Z, Li J, Huang J. 2016. In vitro assessment of physiological changes of watermelon (Citrullus lanatus) upon iron oxide nanoparticles exposure. Plant Physiol Biochem. 108:353–360. doi: 10.1016/j.plaphy.2016.08.003
- Wang H, Kou X, Pei Z, Xiao JQ, Shan X, Xing B. 2011. Physiological effects of magnetite (Fe3O4) nanoparticles on perennial ryegrass (Lolium perenne L.) and pumpkin (Cucurbita mixta) plants. Nanotoxicology. 5:30–42. doi: 10.3109/17435390.2010.489206
- Wu T-M, Hsu Y-T, Lee T-M. 2009. Effects of cadmium on the regulation of antioxidant enzyme activity, gene expression, and antioxidant defenses in the marine macroalga Ulva fasciata. Bot Stud. 50:25–34.
- Wu SG, Huang L, Head J, Chen D-R, Kong I-C, Tang YJ. 2012. Phytotoxicity of metal oxide nanoparticles is related to both dissolved metals ions and adsorption of particles on seed surfaces. Petrol Environ Biotechnol. 3:1–5.
- Yang J, Cao W, Rui Y. 2017. Interactions between nanoparticles and plants: phytotoxicity and defense mechanisms. J Plant Interact. 12:158–169. doi: 10.1080/17429145.2017.1310944
- Zhang H, Jiang Y, He Z, Ma M. 2005. Cadmium accumulation and oxidative burst in garlic (Allium sativum). J Plant Physiol. 162:977–984. doi: 10.1016/j.jplph.2004.10.001
- Zhang Z, Ke M, Qu Q, Peijnenburg WJGM, Lu T, Zhang Q, Ye Y, Xu P, Du B, Sun L, Qian H. 2018. Impact of copper nanoparticles and ionic copper exposure on wheat (Triticum aestivum L.) root morphology and antioxidant response. Environ Pollut. 239:689–697. doi: 10.1016/j.envpol.2018.04.066
- Zuo Y, Zhang F. 2011. Soil and crop management strategies to prevent iron deficiency in crops. Plant Soil. 339:83–95. doi: 10.1007/s11104-010-0566-0
- Zuverza-Mena N, Martínez-Fernández D, Du W, Hernandez-Viezcas JA, Bonilla-Bird N, López-Moreno ML, Komárek M, Peralta-Videa JR, Gardea-Torresdey JL. 2017. Exposure of engineered nanomaterials to plants: insights into the physiological and biochemical responses - A review. Plant Physiol Biochem. 110:236–264. doi: 10.1016/j.plaphy.2016.05.037