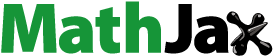
ABSTRACT
This study aimed to investigate the interaction effects of iron oxide nanoparticle (nα-Fe2O3) and its bulk counterpart (bα-Fe2O3) with and/or without citrate interaction on germination and seedling growth of Oenothera biennis L. We prepared buffered suspensions of nα-Fe2O3 and bα-Fe2O3 at 4 levels (0, 0.2, 0.5 and 1 g.L−1). The nα-Fe2O3 increased 89.17% germination percentage, 53.36% germination tolerance index, 82.21% root tolerance index, and 79.42% ferric reducing antioxidant power. The nα-Fe2O3+citrate improved α-Fe2O3 function with a 47% relative hypocotyl length, and 97.32% vigor index2 increase. Leaf area, tolerance index of seedling fresh weight increased at bα-Fe2O3+citrate. Antioxidant enzyme activities are increased in α-Fe2O3+citrate, through which increased biomass and tolerance indices. Oxidative stress control was an important component of this plant’s ability to withstand environmental stress and to succeed in germination and seedling growth. This finding emphasizes that if nα-Fe2O3 were used at low and intermediate concentrations, it would not have toxic effects on this plant, and potentially would result in successful stress tolerance and could act as iron fertilizers for the growth of Oenothera biennis L.
Introduction
As a rapidly developing savoir-faire in the world’s academic and scientific circles, nanotechnology has received considerable interest (Nazerieh et al. Citation2018; Iranbakhsh et al. Citation2018b). Demand for nano-based products in various industries, especially traditional food, medicine, and agriculture is boosting significantly (Babajani et al. Citation2019; Moghanloo et al. Citation2019a; Seddighinia et al. Citation2020). Because of their unique physicochemical properties such as size, surface area, purity, and stability, the interaction between nanoparticles and biomolecules may provoke reactions in exposed plants different from their bulk type (Safari et al. Citation2018; Babajani et al., Citation2019; Moghanloo et al. Citation2019b; Sotoodehnia-Korani et al. Citation2020). Furthermore, these compounds may affect plant cells and transform key vital processes such as seed germination, root initiation and elongation, cellular metabolism, vigor index, and growth rate and biomass in plants at different developmental stages and may modify intracellular redox status (Babajani et al., Citation2019). Seed germination phenomenon and early growth are the crucial developmental phases in the life cycle for the prosperous establishment and performance of plants (Moghanloo et al. Citation2019a; Babajani et al. Citation2018; Seddighinia et al. Citation2020). Many factors influence the onset and progression of seed germination; the germination process is very sensitive to environmental factors such as heavy metals and nanoparticles (Seneviratne et al. Citation2017; Jahani et al. Citation2019).
As an essential nutrient for all cells, iron is one of the fundamental requirements for plant growth, many biological processes including chlorophyll biosynthesis, respiration, and redox reactions (Rui et al. Citation2016; Tripathi et al. Citation2018). Iron is a chief component of cell redox systems and acts as a cofactor of various antioxidant enzymes like catalase (CAT) and peroxidase (POD); iron plays an irreplaceable role in alleviating stress imposed by heavy metal stress (Tripathi et al. Citation2018). This is because it activates plant enzymatic antioxidants like catalase, peroxidase, and an isoform of superoxide dismutase (SOD) that act as a scavenger of reactive oxygen species (ROS), (Tripathi et al. Citation2018). The application of Fe fertilizers (bulk and iron oxide nanoparticles) are still the most effective method to make up Fe deficiency in plants; thus, it is important to improve the utilization efficiency of Fe fertilizers (Rui et al. Citation2016). Nano-fertilizers are an important solution for the gradual release of the required elements and because of the high reactivity of the nanoparticles, they facilitate the intracellular chemical changes and act as catalysts (Elemike et al. Citation2019).
The α-Fe2O3 is the most stable iron oxide polymorph (Pariona et al. Citation2017); therefore, nFe2O3 has been widely applied in biomedicine, agriculture, water and soil treatment and other fields (Rui et al. Citation2016). Nanoparticles are also known as the cause for oxidative stress (Taran et al. Citation2016), increase or decrease in some metals (e.g. Fe), electron transfer to O2 as acceptors to form or to H2O2 to form the extremely reactive OH− radicals. These reactions enhance oxidative stress in the affected cells and damage the plant cells (Dietz and Herth Citation2011).
As a vital organic acid, citric acid has been reported to be closely related to iron stress tolerance (Hu et al. Citation2016). Also, the citrate complex is one of the mobile forms of iron that participate in iron transportation inside plants (Hu et al. Citation2016). The citric acid served utterly well as an antioxidant respiratory metabolism intermediate involving the defense pathways (Hu et al. Citation2016). Citrate can increase the uptake of metals and is a common coating agent for nanoparticles due to its stability and availability (Barrios et al. Citation2015).
Oenothera biennis (Evening primrose, Onagraceae) is a biennial, herbaceous plant grown as an oilseed crop for the production of essential fatty acids (gamma-linoleic acid) and is considered to be a medicinal plant due to its many pharmaceutically active secondary metabolites such as ellagitannins (Granica et al. Citation2013; Greiner and Köhl Citation2014). The evening primrose oil (EPO) has been shown to have several beneficial effects on human health (Granica et al. Citation2013; Greiner and Köhl Citation2014).
The unique and different behaviors of diverse plant species in response to various nano-products have been reported in various plant species (Ghasempour et al. Citation2019). There is no agreed opinion on the impact of nanoparticles upon physiological and biochemical processes in the available literature, both positive and negative effects are noted (Taran et al. Citation2016). These controversial results reiterate the necessity for extensive investigations on the impact of iron oxide nanoparticles (IONPs) on plants (Tombuloglu et al., Citation2019).
The challenge we have today is to determine the amount of stress by different concentrations of these substances. Thus, this study was an attempt to investigate the effects of different levels of nα-Fe2O3 and bα-Fe2O3 with and without citrate interaction on seed germination and seedlings of O.biennis, at early stages of growth by measuring physiological parameters such as germination percentage (GP), germination rate (GR), germination tolerance index (GTI), seed vigor indices, growth and biomass, seed and seedling tolerance indices, and the in vitro evaluation of possible oxidative stress by measuring SOD, CAT, and POD activities, ferric reducing antioxidant power (FRAP), MDA and H2O2 contents. In order to potentially increase the effect of iron oxide on germination and growth indices, it has been used from organic citric acid, which is widely believed to be of great importance for iron mobilization (Hu et al. Citation2016). This research is the first study on the evaluation of the interaction effects of IONP (nα-Fe2O3), bα-Fe2O3, and citrate on germination, physiological changes, and induction of Evening primrose (Oenothera biennis L.) oxidative system.
Materials and methods
Seed preparation and cultivation in plate
Seeds of Oenothera biennis L. were collected from the Barij Essence R&D Department, Kashan, Iran. To sterilize, the seeds were immersed in 3% hydrogen peroxide for 10 min. to surface sterilization and then were rinsed 3 times with sterile deionized water (Greiner and Köhl Citation2014). In this study, 4 levels of nα-Fe2O3 and bα-Fe2O3 (0, 0.2, 0.5, and 1 g.L−1) were prepared, using double-distilled deionized water. IONP to citrate molar ratio in suspensions were 2:3, citrate only solution concentration was 5 mM, and the pH of all solutions and suspensions was adjusted to 6.0-6.3 by NaOH and 0.5 M hydrochloric acid. Since the metal oxides are insoluble in water, to disperse the particles, suspensions of various concentrations were placed twice, 10 min. apart, every time 20 min. in an ultrasonic device (WUC D1OH) at 45°C (Hwang et al. Citation2007). Thirty healthy seeds were placed on each plate (size: 80 * 16 mm) contained a sterile paper filter. Then, 5 ml of the above suspensions were added to each plate and placed in a germinator (Snijders scientific, G120) at 22/18°C day/night temperature, 16h/8h light/dark cycle (photoperiod) with 85% relative humidity for 28 days. The experiment was conducted in a completely randomized design with 3 replications. Seeds whose roots were at least 2 mm long, were considered as germinated seeds (International Rules for Seed Testing, ISTA Citation2009). Seed germination was counted for 10 days with a one-day interval. The first and last days of seed germination were considered on the fourth and the tenth days of cultivation, respectively.
The nα-Fe2O3 powder was purchased from Nano Pioneer Company of Mashhad (NanoSany Corporation, Mashhad, Iran) with a high purity of 98%, average particle size (APS) 40 nm, specific surface area (SSA) 40–60 nm ( and ). The bα-Fe2O3 powder was purchased from CARLO ERBA Reagents, Italy. Citrate powder was purchased from Merck & Co., Germany. The grains were photographed with a Motic BA 300 Spain Photomicroscope.
Quantitative traits
Factors related to germination percentage and rate
where, G = number of total germinated seeds; and T = number of total seeds in the experiment (Amooaghaie et al. Citation2015). Final germination percentage: It was calculated according to the relationship proposed by Ashkan and Moemeni (Citation2013).
Where, Nʹ = Number of seeds germinated on the last day, N = Total number of seeds.
Factors related to seedling
Root, hypocotyl, and seedling lengths of 28 days’ post-culture were calculated using a millimeter ruler and reported in millimeter units. Each treatment was conducted with 3 replicates and each replicate was considered as the mean of all germinated seeds per plate. The mean fresh weight of seedlings was measured at 28 days’ post-cultivation with scales (KERN) with an accuracy of 0.0001 g and reported in milligram units.
Factors related to stress tolerance
Stress tolerance indices in plants depend on changes in growth and germination parameters. In this study, relative seed germination index (RSGI), root tolerance index (RTI), germination tolerance index (GTI), relative hypocotyl or stem length (RHL), vigor index (VI), allometric coefficient (AC), tolerance index of seedling length (TISL), and tolerance index of seedling fresh weight (TIFW) were evaluated in 28-day old seedlings.
After measuring root length (RL) and hypocotyl length (HL), AC was calculated (Huang Citation2006).
RSG and RTI were calculated from the following relationships (Amooaghaie et al. Citation2015).
RSG = (Seeds germinated with IONPs /Seeds germinated with control) * 100
RTI = (Mean RL with IONPs /Mean RL with control) * 100
GTI was calculated from the following equation (Amooaghaie et al. Citation2015).
RHL, which is an appropriate indicator for monitoring stress conditions, was obtained from the following formula (Amooaghaie et al. Citation2015).
RHL = (mean HL with IONPs/mean HL with control) * 100
The following equations were used to evaluate vigor indices (VI), (Vashisth and Nagarajan Citation2010).
Vigor Index 1 = GP * seedling length (Root + Shoot)
Vigor Index 2 = GP * seedling dry weight (Root + Shoot)
TISL and TIFW were evaluated from the following relationships (Laware and Raskar Citation2014).
TISL = (Mean of seedling length with IONPs/Mean of seedling length with control) * 100
TIFW = (Mean of seedling fresh weight with IONPs/Mean of seedling fresh weight with control) * 100
Oxidative stress evaluation
MDA content assay
The MDA content was determined by the thiobarbituric acid method (Heath and Packer Citation1968). A sample of 0.5 g fresh weight tissues was ground in 2.0 ml of 10% trichloroacetic acid (TCA), and the mixture was centrifuged at 10000 rpm for 10 min. One (1) ml supernatant was added to 5 mL of 20% trichloroacetic acid (TCA) containing 0.5 M thiobarbituric acid (TBA), and the mixture was simmered in the water bath for 15 min and then immediately cooled down in the ice bath. After centrifugation at 10000 rpm for 10 min, the supernatant absorbance was measured at 532 nm by a spectrophotometer (T90+UV/Vis spectrophotometer, PG Instruments Ltd). The MDA content was expressed in µmol per g of fresh weight (FW).
H2O2 content assay
Hydrogen peroxide content was determined according to Alexieva et al. (Citation2001). 0.5 g fresh weight was homogenized in an ice bath with 5 ml of a cold 0.1% (m/v) trichloroacetic acid (TCA). The homogenate was centrifuged (12 000 g, 15 min, 4°C) and 0.5 ml of the supernatant was added to 0.5 ml of 100 mM potassium phosphate buffer (pH 7.0) and 1 ml of 1 M KI. The absorbance was read at 390 nm on a spectrophotometer. The H2O2 content was expressed in µmol per g of fresh weight (FW).
Antioxidant enzyme assay
0.5 g of fresh weight were homogenized in 10 ml of 0.05 M phosphate buffer (pH 7.8). The mixture was centrifuged at 4000 rpm for 10 min and stored at 4°C for further use.
SOD activity assay
The SOD activity was measured through the nitroblue tetrazolium (NBT) method (Wang et al. Citation2004). The reaction mixture consisted of 0.5 ml supernatant, 0.5 ml of 130 mM methionine, 0.5 ml of 750 mM NBT, 0.5 ml of 100 mM EDTA-Na, 0.5 mL of 20 mM riboflavin and 3.5 ml of 0.05 M phosphate buffer (pH 7.8). The mixture was illuminated for 20 min, while the control sample prepared in distilled water was placed in the dark. The absorbance was measured at 560 nm.
CAT activity assay
The CAT activity was determined by adding 0.4 ml of supernatant into 3 ml of a solution containing 0.1% H2O2 and 100 mM phosphate buffer (pH 7.0). the absorbance was measured at 240 nm every 60 s for 120 s (Wang et al. Citation2004).
POD activity assay
The POD activity was estimated by the guaiacol colorimetric method (Zhang et al. Citation1995). The POD reaction mixture was prepared by adding 28 ml guaiacol into 50 mL of 100 mM phosphate buffer (pH 7.0). When completely dissolved and cooled down to room temperature, 19 ml of 30% H2O2 was introduced. The assay mixture contained 0.1 ml of supernatant, 3 ml of POD reaction mixture, and 0.9 ml of 100 mM phosphate buffer (pH 7.0). The absorbance was measured at 470 nm.
Redox assessment
FRAP assay
The FRAP of the leaf extract was determined by the method introduced by Oyaizu (Citation1986).
Statistical analysis
All experiments were carried out as a full factorial experiment with a completely randomized design. For all tests, 3 replications were utilized. The statistical analysis of experimental data was verified with the one-way ANOVA, generalized linear test, and correlations. Duncan’s multiple range tests were used to compare the treatments’ means in the statistical package SPSS Version 22. The significance level was set at 5%.
Results
Germination indices
The results of the present study show that the concentration of α-Fe2O3 affected GP and GR. By increasing the concentration of iron in α-Fe2O3 suspensions, GP and GR decreased significantly as compared with the control (P < 0.05). The GP at the level of 1 g.L−1 in nα-Fe2O3 and bα-Fe2O3 was significantly lower 56.72% and 47.77%, respectively, compared with the control (P < 0.05). In other words, at high concentrations, the nα-Fe2O3 showed a more negative effect than the bα-Fe2O3. The GP with nα-Fe2O3+Cit at the 0.2 g.L−1 level on the fourth day significantly increased by 26.35% compared with the control (P < 0.05). Also, at the 1 g.L−1 level of bα-Fe2O3 on day 6, the expected decrease in GP and GR did not occur, similar to the nα-Fe2O3 level. The nα-Fe2O3 and bα-Fe2O3 alone, as well as citrate alone, did not affect GR and GP. However, both nα-Fe2O3+Cit and bα-Fe2O3+Cit had a significant impact on GP and iron oxide in both methods with citrate increased the GR in the short run. So, in the early days, nα-Fe2O3+Cit at the 0.2 and 0.5 g.L−1 levels improved iron function on GP. However, both nano and bulk forms in interaction with citrate interaction at the 1 g.L−1 level, exacerbates the decreasing effect of iron on this variable (GP). However, at the level of 1 g.L−1 nα-Fe2O3+Cit and bα-Fe2O3+Cit, the GP was significantly reduced by 78.9%, 65.46%, respectively, compared with the control (P < 0.05). The FGP was more successful in nα-Fe2O3 treatments, with a significant increase to 16.83% at the 0.2 g.L−1 level, and 20% at the level of 0.5 g.L-1, compared with the control (P < 0.05). Overall, the highest GR was seen in bα-Fe2O3+Cit at 0.2 g.L−1 (6.31), with a significant increase of 10.31% compared in the control (P < 0.5). This factor (the highest GR) in the nα-Fe2O3 was related to the 0.5 g.L−1 level which showed a significant increase of 8.56% compared with the control (P < 0.05; ).
Table 1. Germination percentage and rate of different nα-Fe2O3 and bα-Fe2O3 treatments at 4 levels (0, 0.2, 0.5, 1 g.L−1) with and without citrate interaction.
Growth indices
The iron oxide (α-Fe2O3) concentrations were effective on all growth variables. Considering the factors affecting the seedling growth, in high iron level treatments, a significant decrease in growth indices occurred. Exposure to α-Fe2O3 at the 1 g.L−1 level; RL in nα-Fe2O3 and bα-Fe2O3 treatment decreased 47.09% and 19.66%, respectively, and also HL in nα-Fe2O3 and bα-Fe2O3 treatments decreased 25.67% and 44 21%, severally, were significant compared with the control (P < 0.05). The highest RL and HL were affected by the nanoforms, so at the 0.2 g.L−1 level, nα-Fe2O3 and nFe2O3+Cit considerably increased RL by 81.62% and HL by 45.19%, respectively, compared with the control (P < 0.05). The LA was influenced by nano iron oxide at all levels, so, at the level of 0.2 g.L−1, nα-Fe2O3+Cit showed a 70.04% significant increase, compared with the control (P < 0.05). However, the maximum LA, similar to that of seedling weight, was related to the bulk iron oxide, which at the 0.5 g.L−1 level with and without citrate resulted in a significant increase to 71.73% compared with the control (P < 0.05). As with other growth factors, at the 1 g.L−1 level, we observed a decrease in LA, in nα-Fe2O3 and bα-Fe2O3, by 42.19% and 27.27%, respectively, compared with the control (P < 0.05). The α-Fe2O3+Cit treatments affected on the HL, LA, RL, and seedling weight, even citrate alone caused a significant increase in HL (P < 0.05) and increased seedling weight by improving iron function. The bα-Fe2O3+Cit at the 0.5 g.L−1 level and nα-Fe2O3+Cit at the 0.2 g.L−1 level significantly increased seed fresh weight by 82.75% and 67.70%, in the order given, compared with the control (P < 0.05; , ).
Figure 3. The effect of different nα-Fe2O3 and bα-Fe2O3 treatments with and without citrate interaction on the morphology of Oenothera biennis seedlings. a (control), b (citrate), c (nα-Fe2O3+Cit 0.5 g.L−1), d (nα-Fe2O3 0.5 g.L−1), e (nα-Fe2O3+Cit 0.2 g.L−1), f (nα-Fe2O3 0.2 g.L−1), g (bα-Fe2O3+Cit 0.5 g.L−1), h (bα-Fe2O3 0.5 g.L−1), i (bα-Fe2O3+Cit 1g.L−1), j (nα-Fe2O3+Cit 1g.L−1), k (bα-Fe2O3 1 g.L−1), l (nα-Fe2O3 1g.L−1). The drawn line in each image is 5 mm.
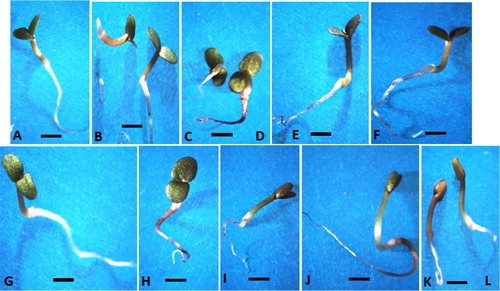
Table 2. Germination growth indices of different nα-Fe2O3 and bα-Fe2O3 treatments at 4 levels (0, 0.2, 0.5, 1 g.L−1) with and without citrate interaction.
Tolerance and vigor indices of germination
Germination indices such as GP and early seedlings growth were affected by α-Fe2O3 treatment. However, by increasing iron concentration, a significant decrease occurred in germination tolerance (P < 0.05). Vigor indices of germination (VI1, VI2) substantially increased at the low and medium levels and decreased at the high levels compared with the control (P < 0.05). The level of 0.5 g.L−1 bα-Fe2O3+Cit and the levels of 0.2 g.L−1 nα-Fe2O3+Cit, significantly increased VI1 (by 77.30%) and VI2 (by 97.39%), respectively, and also citrate alone increased VI2 (by 95%) compared with the control (P < 0.5). Citrate alone significantly increased by 21.26%, RHL and 33.33%, TISL, compared with the control (P < 0.05). The GTI, RTI, RSG, VI2, AC, and RHL peaked at nano-dependent levels. However, the maximum levels of TIFW and VI1 were reported in the bulk forms, the maximum indices of these variables were observed in the nanosized forms at the 0.2 g.L−1 level and in the bulk forms at the 0.5 g.L−1 level ().
Table 3. Tolerance indices of germination at different nα-Fe2O3 and bα-Fe2O3 treatments at 4 levels (0, 0.2, 0.5, 1 g.L−1) with and without citrate interaction.
Physiological indices
The iron oxide (α-Fe2O3) affected all measured physiological parameters and resulted in significant differences in measured parameters, except for CAT and POD, which were negligible when exposed to the 1 g.L−1 level. The iron oxide (α-Fe2O3) increased the activity of antioxidant enzymes. The type of iron oxide used was effective on the CAT and POD enzymes, so that, the nanoforms were effective in CAT and its bulk forms in POD. The maximum activity of antioxidant enzymes was reported in interacting iron oxide with citrate, so CAT activity peaked at the level of 0.2 g.L−1 nα-Fe2O3+Cit with an increase of 89.60%, compared with the control (P < 0.05). Maximum SOD and POD activity was seen at level 0.5 g.L−1 in nα-Fe2O3+Cit and bα-Fe2O3+Cit treatments, respectively, with a significant increase of 41.11% and 43.39%, one by one, compared with the control (P < 0.05; ). According to the results of this study, the lowest antioxidant enzyme activity was reported at the level of 1 g.L−1 nα-Fe2O3+Cit. At all levels of α-Fe2O3, SOD activity varied, and the amount of non-enzymatic antioxidant activity, such as FRAP, also changed accordingly, so that at the level of 0.5 g.L−1 nα-Fe2O3, FRAP increased significantly by 79.42% compared with the control (P < 0.05; ).
Figure 4. The CAT activity of different nα-Fe2O3 and bα-Fe2O3 treatments at 4 levels (0, 0.2, 0.5, 1 g.L−1) with and without citrate interaction. Mean values followed by different letters are significantly different at P < 0.05 according to Duncan’s multiple range test.
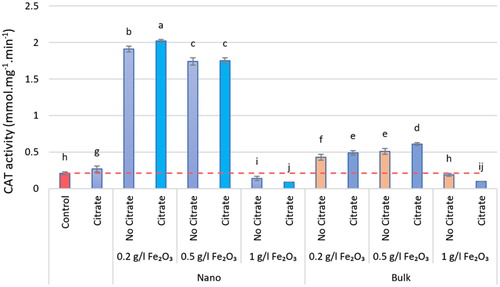
Figure 5. The POD activity of different nα-Fe2O3 and bα-Fe2O3 treatments at 4 levels (0, 0.2, 0.5, 1 g.L−1) with and without citrate interaction. Mean values followed by different letters are significantly different at P < 0.05 according to Duncan’s multiple range test.
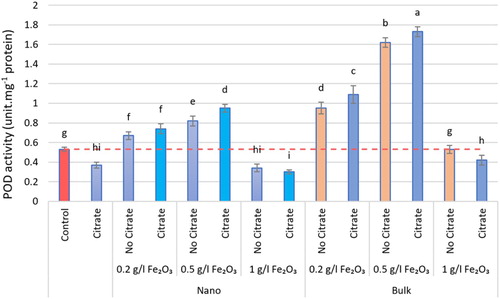
Figure 6. The SOD activity of different nα-Fe2O3 and bα-Fe2O3 treatments at 4 levels (0, 0.2, 0.5, 1 g.L−1) with and without citrate interaction. Mean values followed by different letters are significantly different at P < 0.05 according to Duncan’s multiple range test.
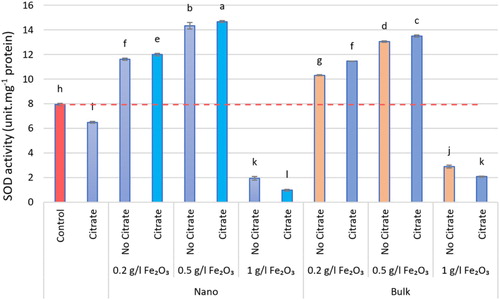
Figure 7. The FRAP assay in different nα-Fe2O3 and bα-Fe2O3 treatments at 4 levels (0, 0.2, 0.5, 1 g.L−1) with and without citrate interaction. Mean values followed by different letters are significantly different at P < 0.05 according to Duncan’s multiple range test.
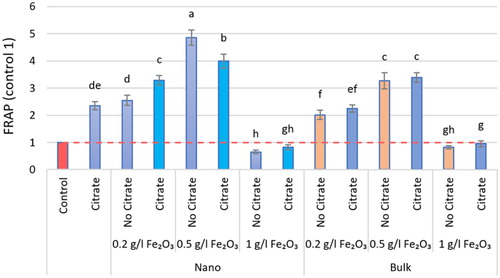
Citrate alone had no effect on physiological indices but was effective in interaction with α-Fe2O3 (α-Fe2O3+Cit) and was associated with a change. Both nα-Fe2O3+Cit and bα-Fe2O3+Cit significantly increased the contents of H2O2 and MDA at the level of 1 g.L−1 compared with the control (P < 0.05; and ). The H2O2 contents in the nα-Fe2O3+Cit and bα-Fe2O3+Cit significantly increased by 78.30% and 76.88%, on an individual basis, compared with the control (P < 0.05). The MDA content for nα-Fe2O3 and bα-Fe2O3 significantly increased by 39.72% and 35.66%, respectively, compared with the control (P < 0.05). Although at the low and medium levels, MDA had a negligible decreasing trend, H2O2 was elevated at all levels, even in the control.
Figure 8. The H2O2 content in different nα-Fe2O3 and bα-Fe2O3 treatments at 4 levels (0, 0.2, 0.5, 1 g.L−1) with and without citrate interaction. Mean values followed by different letters are significantly different at P < 0.05 according to Duncan’s multiple range test.
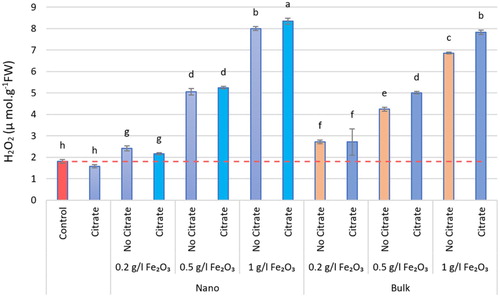
Figure 9. The MDA content in different nα-Fe2O3 and bα-Fe2O3 treatments at 4 levels (0, 0.2, 0.5, 1 g.L−1) with and without citrate interaction. Mean values followed by different letters are significantly different at P < 0.05 according to Duncan’s multiple range test.
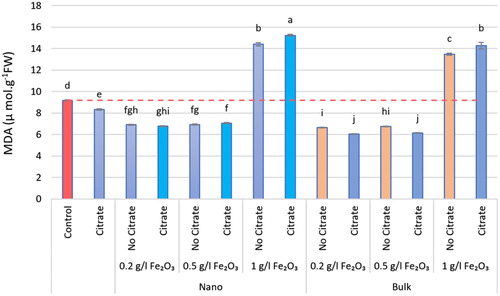
According to and , which is a summary of the correlations table, it was observed that Vig1 (VI1) and SOD are more strongly associated (with a 10% impact) with other indicators. Also, according to , the variables, the farther away from the center of the circle, the more effective they are; and the variables, the nearer the center of the circles, the less impact they have on other indicators. Therefore, during the correlation analysis, four Vig1, SOD, RTI, and FGP indicators were identified with the greatest impact and correlation on the other tested indicators.
Figure 10. Control effect of the variables’ coefficient. The importance of each variable can be summarized in the figure.
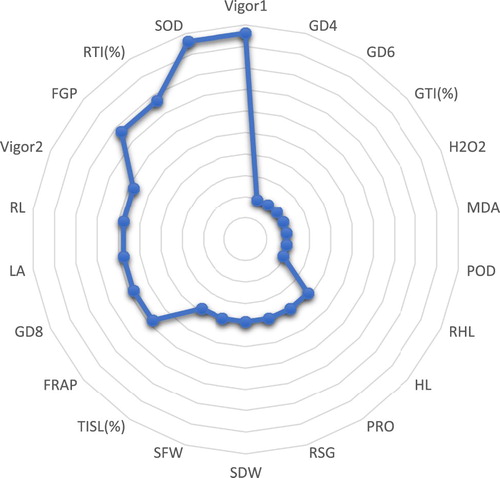
Table 4. Control effect of the variables’ coefficient. The importance of each variable can be summarized in the below table.
Discussion
Based on the results of the present study, the nα-Fe2O3 with a higher solubility advantage in suspension, greater penetration and absorption into the seed coat and subsequently facilitating root emergence as well as higher bioavailability for rootstocks than iron oxide bulk (Alidoust and Isoda Citation2013) led to a greater success in increasing GP and RL. This is consistent with previous studies (Ren et al. Citation2011; Racuciu Citation2012; Pariona et al. Citation2016; Rui et al. Citation2016) on accelerating and enhancing germination by IONPs. The IONPs increased germination, root growth, and chlorophyll content in watermelon planted in quartz-containing soil (Li et al. Citation2013), in Chinese mung bean plants grown in silica (Ren et al. Citation2011) and soybean plants (Alidoust and Isoda Citation2013). The Fe3O4 nanoparticles also increased the GP of Quercus macdougallii by 33% (Pariona et al. Citation2016). In the present study, nα-Fe2O3 at the 0.5 g.L−1 level significantly increased seed germination by 20%, at the 0.2 g.L−1 level increased RL by 81.6% compared with the control (P < 0.05), which may possibly help the root cells to open the water canals, for efficiently absorbing water, inorganic ions, and other nutrients (Ren et al. Citation2011). The nα-Fe2O3+Cit at the 0.2 g.L−1 level was significantly effective in the early days of germination (P < 0.05). The iron-citrate complex is one of the transition forms of iron in the plant and helps to increase the fluidity and mobility of iron (Hell and Stephan Citation2003; Alidoust and Isoda Citation2013). Citrate treatment can induce endogenous citrate production by plants (Darandeh and Hadavi Citation2012); however, an increase in these carboxylates is reported in iron deficiency conditions (Rui et al. Citation2016). Citrate-coated cerium oxide nanoparticles (nCeO2) increased root biomass in radish (Barrios et al. Citation2015). Beta cyclodextrin-coated iron oxide nanoparticles stimulated Zea mays proliferation compared with the control (Racuciu Citation2012).
Iron is a critical element for plant growth and biomass (Pariona et al. Citation2017). In the present study, the longitudinal growth of Oenothera biennis seedlings exposed to nα-Fe2O3 at the 0.2 g.L−1 level significantly increased compared with the control (P < 0.05). In peanut (Arachis hypogaea) plants, ɣ-Fe2O3 increased root length and plant height (Rui et al. Citation2016). The HL factor affects RHL; RL and the AC factors affect RTI and TISL. The GTI influenced the changes in germination vigor indices (VI1 and VI2). Furthermore, VI1 and SOD, then RTI and FGP had the highest percentage of correlation compared to other indicators ( and ). At the 1 g.L−1, all treatments, and at the level of 0.5 g.L−1 nα-Fe2O3+Cit, the AC decreased due to a more pronounced decrease in RL than HL. This may be due to the high sensitivity of the root apex meristem to heavy metals as was reported by Cui and Zhao (Citation2011). Biomass and LA indices at both low and intermediate levels of α-Fe2O3 showed a significant increase compared with the control (P < 0.05), although the maximum of these indices occurred in the bα-Fe2O3 treatments. Leaf growth is one of the determinants of production (Farrant Citation2000). Downward regulation of FRO2 gene expression (ferric chelate reductase, reduces iron at the root level, then transported via plasma membrane) in ɣ-Fe2O3 and Fe3+ treatments, compared with the control, showed that Citrus maxima plants could utilize both types of iron which sprayed to the leaf. The relative levels of Nramp3 gene expression (a vacuolar membrane transporter that is a carrier of Fe2+) exposed to ɣ-Fe2O3 and Fe3+, were significantly lower than the controls. Both treatments were able to provide iron to Citrus maxima seedlings. Generally, plants can transfer IONPs and Fe3+ to protect themselves and their development by activating many physiological and molecular processes (Hu et al., Citation2017a). According to Alidoust and Isoda’s (Citation2013) study, bulk iron oxide was slightly better able to increase the photosynthesis rate in soybean than in the nano form, and their effects on this index were not size-dependent. Probably in the Oenothera biennis plant, n (0.2 gL−1) / b (0.5 gL−1) α-Fe2O3+Cit increased the materialization and biomass by increasing the amount of chlorophyll and eventually reached the maximum LA by increasing TIFW. Therefore, the effect of α-Fe2O3 on the biomass and leaf area of Oenothera biennis was not size-dependent, and in this regard, the role of citrate in enhancing iron function was important by increasing the mobility of iron from the environment into the plant tissues (Hell and Stephan Citation2003). In this study, citrate also had a significant role in increasing HL, RHL, and TISL, as its foliar spray increased Gazania rigens growth and flowering (Talebi et al. Citation2014). It was also helpful in some physiological parameters of Salvia and Lilium (Eidyan et al. Citation2014), and beans (Ghazijahani et al. Citation2014). The study by Alidoust and Isoda (Citation2013) showed that bulk and nano iron oxide with citrate coating could significantly increase the efficiency of iron oxide as an iron-containing fertilizer.
Nanoparticles can activate anti-stress activities in plants (Taran et al. Citation2016). To eliminate ROS and reduce the toxic effects of heavy metals, plants are equipped with enzymatic antioxidant systems including SOD and POD (Konate et al. Citation2017). According to our results, IONPs induced higher oxidative stress and higher activity of antioxidant enzymes than bα-Fe2O3, which is consistent with Wang et al. (Citation2011) results. Because iron is involved in enzyme activity and RNA synthesis (Dhoke et al. Citation2013), and due to the high reactivity of the nanoparticles, the metal nanoparticles can facilitate intracellular chemical changes and act as catalysts (Lin and Xing Citation2007). The effect of nFe2O3 on transgenic rice resulted in significantly higher SOD and POD activities than in the control, and in addition to increasing seedling growth indices in watermelon plants, increased CAT and SOD activities (Li et al. Citation2013). As in our results, IONPs in Cucurbita pepo (Pariona et al. Citation2017) had a positive effect on plant growth and increased activity of antioxidant enzymes. According to our results, not only did SOD activity increased with increasing H2O2 levels, but also at all treatments, even control, high levels of H2O2 were seen. ROS are required for cellular proliferation during morphogenesis of plant organs and participate in increased germination parameters (Pariona et al. Citation2016). The accumulation of H2O2 may alter plant metabolism in various ways (Ren et al. Citation2011). Therefore, raising the level of H2O2 at all levels, at the beginning of germination and on the first days of Oenothera biennis seedling growth, can be a useful strategy for plant growth. ROS to some extent that they do not disturb plant cell balance, control growth and stress response processes (Miller et al. Citation2007), and affect plant cells as biologically active substances at the molecular and biological level (Iranbakhsh et al. Citation2018a, Citation2020). The MDA was low at the 0.2 and 0.5 g.L−1 levels, which might be initially nano and bulk forms produced ROS, but subsequently, the plant defense system improved inductive stress (Hu et al. Citation2017b), Iron oxides at optimum concentrations had no toxic effects on Citrus maxima plants, and low levels of MDA were useful in protecting cell membrane structure and function (Li et al. Citation2013). On the other hand, the CAT and POD enzymes increased maybe for decreasing SOD-produced H2O2 levels, so in our study, MDA minimized at both 0.2 and 0.5 g.L−1 levels. Despite the high antioxidant levels, increased ROS production, resulting from the metal stresses, reported by Zhao et al. (Citation2005). Plants produce adaptive responses to stress induced by nanoparticles (Barrios et al. Citation2015). Also, the FRAP level, together with FGP and RSG indices, significantly increased at the level of 0.5 g.L−1 nα-Fe2O3. The IONPs increased the reducing activity of watermelon roots and seedlings (Li et al. Citation2013). Thus, the enzymes and metabolites responsible for ROS digestion play an important role in the success of germination (Tommasi et al. Citation2001). The activities of antioxidant enzymes such as POD act as a vital need to deal with stress in plants (Iranbakhsh et al. Citation2017, Citation2018b). According to the results of the present study, the maximum POD activity was at the level of 0.5 g.L−1 in the bα-Fe2O3+Cit treatment. PODs oxidize a wide range of substrates and accelerate plant elongation by producing oxygen radicals, or by regulating H2O2 concentrations (Pariona et al. Citation2016). Probably the POD activity here increased the biomass and LA. Adding both types of iron to the peanut plants, stimulated growth by producing certain amounts of ROS (Rui et al. Citation2016). In the present study, maximum enzyme activity occurred in interaction with citrate. In tomato plants, citrate coated cerium oxide nanoparticles at the 500 mg/kg level increased the CAT activity, and citrate with the nanoparticles affected chemical responses (Barrios et al. Citation2015). In our study, nα-Fe2O3+Cit also increased the activity of the enzymes. The size of nFe2O3 decreases with adsorption of citrate ions, which increases electrostatic drift (Alidoust and Isoda Citation2013), altering chemical and surface properties and interfering with the environment (Barrios et al. Citation2015). Citrate has been reported to migrate with iron during electrophoresis (Rui et al. Citation2016). This suggests that citrate plays a role in the remote transport of iron. However, the formation of different types of iron citrate complexes, and their ratio varies depending on the pH, temperature, and molar ratio of citrate to iron oxide (Alidoust and Isoda Citation2013). Similar to our results, high concentrations of iron-citrate in Arabidopsis (Lee et al. Citation2010) and Triticum vulgare (Lebedev et al. Citation2014) decreased GP, RL, and LA, which these negative dose-dependent effects, were mainly due to the oxidative stress of ROS (Alidoust and Isoda Citation2013). However, nFe2O3 at the 0–5 g.L−1 levels also had no toxic effects on cucumber, lettuce, and radish seeds. These different plant responses depend on various factors such as plant type, different forms of iron oxide, size, and concentration of nanoparticles (Pariona et al. Citation2017) and different conditions in which the experiment is performed. Excess iron leads to mutation increased ROS production, and stimulation of oxidative stress (Pariona et al. Citation2016). The MDA content is closely related to the stress damage and signifies the presence of toxins in the environment; any increase, due to excess radicals, indicates physiological stress (Li et al. Citation2013). A significant increase in MDA, along with a significant increase in H2O2 at 1 g.L−1, occurred with a decrease in FRAP content, antioxidant enzymes, and LA (P < 0.05). SOD, CAT, and POD are the key enzymes in the antioxidant defense system in plants, however, at high concentrations of iron, ROS bursts occur and the plant is unable to regulate the activity of these enzymes (Hu et al. Citation2017a). All of the stress-related factors evaluated were dependent upon germination and growth factors, and based on our results, at high levels, there was a significant decrease in these indices, and the maximum MDA and H2O2 were reported at the level of 1 g.L−1 nα-Fe2O3. Growth inhibition is one of the indicators of metal toxicity in plants (Pariona et al. Citation2017). Excess H2O2, which is not removed at the proper time, reduces shoot growth (Barrios et al. Citation2015). According to the results, the level of H2O2 was greatly increased despite the decrease in SOD activity at level 1 gL−1 α-Fe2O3. Elimination of superoxide anion without the involvement of the SOD enzyme may occur through direct reactions with ascorbate or glutathione and through an alternative route for non-enzymatic removal of superoxide, which may lead to the production of additional H2O2 (Mittler Citation2002; Pinheiro et al. Citation2004). However, accurate measurement of metabolic cascades in biochemical pathways can further determine the imbalance between H2O2 production and consumption (Pinheiro et al. Citation2004). Citrate at high levels had more negative effects on GP, antioxidant enzymes, FRAP, growth, and LA. Even the number of oxidizing metabolites increased, surprisingly, the length of the hypocotyls and roots of Oenothera biennis seedlings increased despite these critical conditions. As previously reported, HL, RHL, and TISL, under the direct influence of citrate alone, were increased in Oenothera biennis seedlings and also, they had an increasing pattern at the level of 1 g.L−1 nano and bulk with citrate. It can be claimed that at these high levels, the bulk and nano-forms in the presence of citrate, probably were not efficiently adsorbed due to the aggregation and deposition phenomena, and citrate was more adsorbed than them. Exogenous citric acid can be released from coated nanoparticles and may accelerate or stimulate growth (Barrios et al. Citation2015) and induce endogenous citrate as mentioned. On the other hand, heavy metal stresses are capable of disrupting the aquatic relationships of plants and, thereby, damaging plants (Barcelo and Poschenrieder Citation2004). Therefore, in this treatment, a severe decrease in antioxidant system activity, biochemical and LA responses may also be caused by a drastic reduction of water potential and induction of secondary environmental and oxidative stresses. The increase of longitudinal parameters under these conditions might be due to the positive effects of citrate. Citrate increased the water conductivity to Lilium cut flowers xylem, and post-harvest longevity and was able to restore green leaf color in chlorosis plants (Darandeh and Hadavi Citation2012). Citrate is one of the intermediate substances in the Krebs cycle and it produces cellular energy through oxidative phosphorylation in the mitochondria. Citrate can serve as a substrate for carbohydrates and fatty acids biosynthesis (Hu et al. Citation2016).
Conclusions
Due to the role of iron in germination and increasing biomass in plants, our physiological results showed that nα-Fe2O3 was effective on seed germination, longitudinal growth, antioxidant enzyme activity, and FRAP, but these effects were concentration-dependent. The IONPs at appropriate concentrations for optimum growth can potentially be an effective iron-containing fertilizer for Oenothera biennis seed germination and seedling growth. This finding emphasizes that if nanoparticles were used at their optimum concentration, they would not have toxic and harmful effects on biological systems such as plants. Overall, the interaction between citrate and iron oxide (α-Fe2O3) at low and intermediate levels increased the GR and GP in the first days of growth and also increased the effective antioxidant capacity of the Oenothera biennis seedlings. The highest CAT activity occurred at the level of 0.2 g.L−1 nα-Fe2O3+Cit. However, induction of the enzymatic antioxidant system, especially SOD, and non-enzymatic antioxidant systems such as FRAP in the presence of IONPs, and the upregulation of POD enzyme activity at the level of 0.5 g.L−1 bα-Fe2O3 reduced the damaging effects of cellular oxidation. This successful antioxidant defense process did not occur at the 1 g.L−1 level. Therefore, oxidative stress control is an important component of the plant’s ability to withstand environmental stress and to succeed in germination and seedling growth.
The authors suggest that, if we treat the level of 0.2 g.L−1 nα-Fe2O3+Cit on Oenothera biennis seeds, for the first five days, it will increase GP and GR initially, then if used nα-Fe2O3 without citrate at the 0.5 g.L−1 level, the GP will be maximized. It is also recommended to use bα-Fe2O3+Cit at 0.5 g.L−1 level at the time of seedling growth to achieve greater biomass and LA. Our results will help answer the question of what physiological changes occur in plants under these conditions. However, under these conditions, chlorophyll content and iron content of plant tissues have to be measured and further studies are needed to explain the mechanism of uptake, transport, and interaction between nα-Fe2O3 and plant tissues at the cellular level. Our results also highlight the need to further investigate the effects of IONPs on environmental systems and plants.
Disclosure statement
No potential conflict of interest was reported by the author(s).
Additional information
Funding
Notes on contributors
Zhika Asadi-Kavan
Zhika Asadi-Kavan, Phd student in plant physiology, her research focuses on the effects of nanoparticles on physiological changes in medicinal plants. Science and Research Branch, Islamic Azad University, Tehran, Iran.
Ramazan Ali Khavari-Nejad
Ramazan Ali Khavari-Nejad, a professor in plant physiology and biochemistry, his primary research interests are, plant physiology and biochemistry, Kharazmi University and Science and Research Branch, Islamic Azad University, Tehran, Iran.
Alireza Iranbakhsh
Alireza Iranbakhsh, a professor in biology, Plant Sciences; Plant Cell and Development, his primary research interests are Cell and Tissue Culture, Molecular Development, Epigenetic, role of Gene Expression. Science and Research Branch, Islamic Azad University, Tehran, Iran.
Farzaneh Najafi
Farzaneh Najafi, Associate Professor in Plant Biology, her primary research interests are the effect of abiotic and biotic stresses on plants, the study of plant secondary metabolites. Kharazmi University, Tehran, Iran.
Notes
* To cite this article: Zhika Asadi Kavan, Ramazan Ali Khavari-Nejad, Alireza Iranbakhsh, Farzaneh Najafi. 2020. Cooperative effects of iron oxide nanoparticle (α-Fe2O3) and citrate on germination and oxidative system of evening primrose (Oenthera biennis L.). Journal of Plant Interactions.
References
- Alexieva V, Sergiev I, Mapelli S, Karanov E. 2001. The effect of drought and ultraviolet radiation on growth and stress markers in pea and wheat. Plant Cell Environ. 24:1337–1344. doi: 10.1046/j.1365-3040.2001.00778.x
- Alidoust D, Isoda A. 2013. Effect of γFe2O3 nanoparticle on the photosynthetic characteristic of soybean (Glycine max (L.) Merr.): foliar spray versus soil amendment. Acta Physiol Plant. 35:3365–3375. doi: 10.1007/s11738-013-1369-8
- Amooaghaie R, Tabatabaei F, Ahadi AM. 2015. Role of hematin and sodium nitroprusside in regulating Brassica nigra seed germination under nanosilver and silver nitrate stresses. Ecotoxicol Environ Saf. 113:259–270. doi: 10.1016/j.ecoenv.2014.12.017
- Ashkan A, Moemeni J. 2013. Effect of salinity stress on seed germination and seedling vigor indices of two halophytic plant species (Agropyron elongatum and A. pectiniforme). Int J Agric Crop Sci. 5:2669–2676.
- Babajani A, Iranbakhsh A, Oraghi Ardebili Z, Eslami B. 2018. Seed priming with non-thermal plasma modified plant reactions to selenium or zinc oxide nanoparticles: cold plasma as a novel emerging tool for plant science. Plasma Chem Plasma Process. 39:21–34. doi:10.1007/s11090-018-9934-y.
- Babajani A, Iranbakhsh A, Oraghi Ardebili Z, Eslami B. 2019. Differential growth, nutrition, physiology, and gene expression in Melissa officinalis mediated by zinc oxide and elemental selenium nanoparticles. Environ Sci Pollut Res. 26:24430–24444. doi:10.1007/s11356-019-05676-z.
- Barcelo J, Poschenrieder C. 2004. Heavy Metal Stress in Plants. From Biomolecules to Ecosystems, Edition: Second Chapter: 9 Publisher: Springer. Editors: Prasad M N V. pp: 223–248.
- Barrios AC, Rico CM, Trujillo-Reyes J, Medina-Velo IA, Peralta-Videa JR, Gardea-Torresdey JL. 2015. Effects of uncoated and citric acid coated cerium oxide nanoparticles, bulk cerium oxide, cerium acetate, and citric acid on tomato plants. Sci Total Environ. doi:10.1016/j.scitotenv.2015.11.143.
- Cui Y, Zhao N. 2011. Oxidative stress and change in plant metabolism of maize (Zea mays L.) growing in contaminated soil with elemental sulfur and toxic effect of zinc. Plant Soil Environ. 57:34–39. doi: 10.17221/193/2010-PSE
- Darandeh N, Hadavi E. 2012. Effect of pre-harvest foliar application of citric acid and malic acid on chlorophyll content and post-harvest vase life of Lilium cv. Brunello. Front Plant Sci. January. Volume 2: article106.
- Dhoke SK, Mahajan P, Kamble R, Khanna A. 2013. Effect of nanoparticles suspension on the growth of mung (Vigna radiata) seedlings by foliar spray method. Nanotechnol Dev. 3:e1. doi:10.4081/nd.2013.e1.
- Dietz KJ, Herth S. 2011. Plant nanotoxicology. Trends Plant Sci. 16(11):582–589. doi: 10.1016/j.tplants.2011.08.003
- Eidyan B, Hadavi E, Moalemi N. 2014. Pre-harvest foliar application of iron sulfate and citric acid combined with urea fertigation affects growth and vase life of tuberose (Polianthes tuberosa L.) ‘por-par’. Hortic Environ Biotechnol. 55:9–13. doi: 10.1007/s13580-014-0061-2
- Elemike EE, Uzoh IM, Onwudiwe DC, Babalola OO. 2019. The role of nanotechnology in the Fortification of plant nutrients and improvement of crop production. Appl Sci. 9:499. doi: 10.3390/app9030499
- Farrant JM. 2000. A comparison of mechanisms of desiccation tolerance among three angiosperm resurrection plant species. Plant Ecol. 151:29–39. doi: 10.1023/A:1026534305831
- Ghasempour M, Iranbakhsh A, Ebadi M, Oraghi Ardebili Z. 2019. Multi-walled carbon nanotubes improved growth, anatomy, physiology, secondary metabolism, and callus performance in Catharanthus roseus: an in vitro study. 3 Biotech. 9:404. doi:10.1007/s13205-019-1934-y.
- Ghazijahani N, Hadavi E, Jeong BR. 2014. Foliar sprays of citric acid and salicylic acid alter the pattern of root acquisition of some minerals in sweet basil (Ocimum basilicum L.). Front Plant Sci. 5. article 573. doi:10.3389/fpls.2014.00573.
- Granica S, Czerwińska ME, Piwowarski JP, Ziaja M, Kiss AK. 2013. Chemical composition, antioxidative and anti-inflammatory activity of extracts prepared from aerial parts of Oenothera biennis L. and Oenothera paradoxa Hudziok obtained after seeds cultivation. J Agric Food Chem. 61:801–810. doi: 10.1021/jf304002h
- Greiner S, Köhl K. 2014. Growing evening primroses (Oenothera). Front. Plant sci. 5. article 38. doi:10.3389/fpls.2014.00038.
- Heath RL, Packer L. 1968. Photoperoxidation in isolated chloroplasts: Kinetics and stoichiometry of fatty acid peroxidation. Arch Biochem Biophys. 125:189–198. doi: 10.1016/0003-9861(68)90654-1
- Hell R, Stephan UW. 2003. Iron uptake, trafficking, and homeostasis in plants. Planta. 216(4):541–551. doi: 10.1007/s00425-002-0920-4
- Hu J, Guo H, Li J, Gan Q, Wang Y, Xing B. 2017a. Comparative impacts of iron oxide nanoparticles and ferric ions on the growth of Citrus maxima. Environ Pollut. 221:199–208. doi: 10.1016/j.envpol.2016.11.064
- Hu J, Guo H, Li J, Wang Y, Xiao L, Xing B. 2017b. Interaction of γ-Fe2O3 nanoparticles with Citrus maxima leaves and the corresponding physiological effects via foliar application. J Nanobiotechnol. 15:51. doi: 10.1186/s12951-017-0286-1
- Hu L, Zhang Z, Xiang Z, Yang Z. 2016. Exogenous application of citric acid Ameliorates the adverse effect of heat stress in Tall Fescue (Lolium arundinaceum). Front Plant Sci. 7:179.
- Huang B. 2006. Plant-environment interactions. 3rd ed. CRC Press. Taylor and Francis group; p. 388. ISBN-13: 978-0849337277.
- Hwang SS, Park JS, Namkoong W. 2007. Ultrasonic-assisted extraction to release heavy metals from contaminated soil. J Ind Eng Che. 13(4):650–656.
- Iranbakhsh A, Ardebili NO, Ardebili ZO, Shafaati M, Ghoranneviss M. 2018a. Non-thermal plasma-induced expression of heat shock factor A4A and improved wheat (Triticum aestivum L.) growth and resistance against salt stress. Plasma Chem Plasma Process. 38(1):29–44. doi: 10.1007/s11090-017-9861-3
- Iranbakhsh A, Ardebili ZO, Molaei H, Ardebili NO, Amini M. 2020. Cold plasma up-regulated expressions of WRKY1 transcription factor and genes involved in biosynthesis of cannabinoids in Hemp (Cannabis sativa L.). Plasma Chem Plasma Process. 40(2):527–537. doi:10.1007/s11090-020-10058-2.
- Iranbakhsh A, Ghoranneviss M, Oraghi Ardebili Z, Oraghi Ardebili N, Hesami Tackallou S, Nikmaram H. 2017. Non-thermal plasma modified growth and physiology in Triticum aestivum via generated signaling molecules and UV radiation. Biol Plant. 61:702–708. doi: 10.1007/s10535-016-0699-y
- Iranbakhsh A, Oraghi Ardebili Z, Oraghi Ardebili N, Ghoranneviss M, Safari N. 2018b. Cold plasma relieved toxicity signs of nano zinc oxide in Capsicum annuum cayenne via modifying growth, differentiation, and physiology. Acta Physiol Plant. 40:154. doi: 10.1007/s11738-018-2730-8
- ISTA. ISTA rules. 2009. International seed testing association. Zurich, Switzerland: International Seed Testing Association. seedtest.org.
- Jahani S, Saadatmand S, Mahmoodzadeh H, Khavari-Nejad RA. 2019. Effect of foliar application of cerium oxide nanoparticles on growth, photosynthetic pigments, electrolyte leakage, compatible osmolytes, and antioxidant enzymes activities of Calendula officinalis L. Biologia. 74:1063–1075. doi:10.2478/s11756-019-00239-6.
- Konate A, He X, Rui Y, Zhang Z. 2017. Magnetite (Fe3O4) nanoparticles alleviate growth inhibition and oxidative stress caused by heavy metals in young seedlings of cucumber (Cucumis Sativus L). ITM Web of Conferences. 12:03034. doi:10.1051/itmconf/20171203034.
- Laware S, Raskar S. 2014. Influence of zinc oxide nanoparticles on growth, flowering, and seed productivity in onion. Int J Curr Microbiol Appl Sci. 3(7):874–881.
- Lebedev SV, Korotkova AM, Osipova EA. 2014. Influence of Fe0 nanoparticles, magnetite Fe3O4 nanoparticles, and iron (II) sulfate (FeSO4) solutions on the content of photosynthetic pigments in Triticum vulgare. Russ J Plant Physl+. 61(4):564–569. doi: 10.1134/S1021443714040128
- Lee CW, Mahendra S, Zodrow K, Li D, Chang Y, Braam J, Alvarez PJJ. 2010. Developmental phytotoxicity of metal oxide nanoparticles to Arabidopsis Taliana. Environ Toxicol Chem. 29:669–675. doi: 10.1002/etc.58
- Li J, Chang PR, Huang J, Wang Y, Yuan H, Ren O. 2013. Physiological effects of magnetic iron oxide nanoparticles towards watermelon. J Nanosci Nanotechno. 13:5561–5567. doi: 10.1166/jnn.2013.7533
- Lin D, Xing B. 2007. Phytotoxicity of nanoparticles: inhibition of seed germination and root growth. Environ Pollut. 150(2):243–250. doi: 10.1016/j.envpol.2007.01.016
- Miller G, Suzuki N, Rizhsky L, Hegie A, Koussevitzky S, Mittler R. 2007. Double mutant deficient in cytosolic and thylakoid ascorbate peroxidase reveals a complex mode of interaction between reactive oxygen species, plant development and response to abiotic stresses. Plant Physiol. 144:1777–1785. doi: 10.1104/pp.107.101436
- Mittler R. 2002. Oxidative stress, antioxidants and stress tolerance. Trends Plant Sci. 7:405–410. doi: 10.1016/S1360-1385(02)02312-9
- Moghanloo M, Iranbakhsh A, Ebadi M, Nejad Satari T, Oraghi Ardebili Z. 2019a. Seed priming with cold plasma and supplementation of culture medium with silicon nanoparticle modified growth, physiology, and anatomy in Astragalus fridae as an endangered species. Acta Physiol Plant. 41:54. doi:10.1007/s11738-019-2846-5.
- Moghanloo M, Iranbakhsh A, Ebadi M, Oraghi Ardebili Z. 2019b. Differential physiology and expression of phenylalanine ammonia-lyase (PAL) and universal stress protein (USP) in the endangered species Astragalus fridae following seed priming with cold plasma and manipulation of culture medium with silica nanoparticles. 3 Biotech. 9:288. doi:10.1007/s13205-019-1822-5.
- Nazerieh H, Oraghi Ardebili Z, Iranbakhsh A. 2018. Potential benefits and toxicity of nano selenium and nitric oxide in peppermint. Acta Agric Slov. 111:357–368. doi: 10.14720/aas.2018.111.2.11
- Oyaizu M. 1986. Studies on products of browning reaction: antioxidative activities of products of browning reaction prepared from glucosamine. Japanese Journal of Nutrition and Dietetics. 44:307–315. doi: 10.5264/eiyogakuzashi.44.307
- Pariona N, Martinez AI, Hdz-Garci HM, Cruz LA, Hernandez-Valdes A. 2017. Effects of hematite and ferrihydrite nanoparticles on germination and growth of maize seedlings. Saudi J Biol Sci. 24:1547–1554. doi: 10.1016/j.sjbs.2016.06.004
- Pariona N, Martinez AI, Hernandez-Flores H, Ricardo CT. 2016. Effect of magnetite nanoparticles on the germination and early growth of Quercus macdougallii. Sci Total Environ. 575:869–875. doi:10.1016/j.scitotenv.2016.09.128.
- Pinheiro HA, DaMatta FM, Chaves ARM, Fontes EPB, Loureiro ME. 2004. Drought tolerance in relation to protection against oxidative stress in clones of Coffea canephora subjected to long-term drought. Plant Sci. 167:1307–1314. doi: 10.1016/j.plantsci.2004.06.027
- Racuciu M. 2012. Iron oxide nanoparticles coated with β-cyclodextrin polluted of Zea mays plantlets. Nanotechnol Dev. 2:e6. doi: 10.4081/nd.2012.e6
- Ren HX, Liu L, Ch L, He SY, Huang J, Li JL, Zhang Y, Huang XJ, Gu N. 2011. Physiological investigation of magnetic iron oxide nanoparticles towards Chinese mung bean. J Biomed Nanotechnol. 7:677–684. doi: 10.1166/jbn.2011.1338
- Rui M, Ma C, Hao Y, Guo J, Rui Y, Tang X, Zhao Q, Fan X, Zhang Z, Hou T, Zhu S. 2016. Iron oxide nanoparticles as a potential iron fertilizer for peanut (Arachis hypogaea). Front Plant Sci. 7:815. doi: 10.3389/fpls.2016.00815
- Safari M, Oraghi Ardebili Z, Iranbakhsh A. 2018. Selenium nano-particle induced alterations in expression patterns of heat shock factor A4A (HSFA4A), and high molecular weight glutenin subunit 1Bx (Glu-1Bx) and enhanced nitrate reductase activity in wheat (Triticum aestivum L.). Acta Physiol Plant. 40:117. doi:10.1007/s11738-018-2694-8.
- Seddighinia FS, Iranbakhsh A, Oraghi Ardebili Z, Nejad Satari T, Soleimanpour S. 2020. Seed priming with cold plasma and multi-walled carbon nanotubes modified growth, tissue differentiation, anatomy, and yield in bitter melon (Momordica charantia). J Plant Growth Regul. 39:87–98. doi:10.1007/s00344-019-09965-2.
- Seneviratne M, Rajakaruna N, Rizwan M, Madawala HMSP, Ok YS, Vithanage M. 2017. Heavy metal-induced oxidative stress on seed germination and seedling development: a critical review. Environ Geochem Health. doi:10.1007/s10653-017-0005-8.
- Sotoodehnia-Korani S, Iranbakhsh A, Ebadi M, Majd A, Ardebili ZO. 2020. Selenium nanoparticles induced variations in growth, morphology, anatomy, biochemistry, gene expression, and epigenetic DNA methylation in Capsicum annuum; an in vitro study. Environ Pollut. doi:10.1016/j.envpol.2020.114727.
- Talebi M, Hadavi E, Jaafari N. 2014. Foliar sprays of citric acid and malic acid modify growth, flowering, and root to shoot ratio of Gazania (Gazania rigens L.): A comparative analysis by ANOVA and structural equation modeling. Adv Agric. Article ID 147278, 6 pages.
- Taran N, Batsmanova L, Kovalenko M, Okanenko A. 2016. Impact of metal nanoform colloidal solution on the adaptive potential of plants. Nanoscale Res Lett. 11:89. doi: 10.1186/s11671-016-1294-z
- Tombuloglu H, Slimani Y, Tombuloglu G, Korkmaz AD, Baykal A, Almessiere M, Ercan I. 2019. Impact of superparamagnetic iron oxide nanoparticles (SPIONs) and ionic iron on the physiology of summer squash (Cucurbita pepo): A comparative study. Plant Physiol Bioch. 139:56–65. doi: 10.1016/j.plaphy.2019.03.011
- Tommasi F, Paciolla C, De Pinto MC, De Gara L. 2001. A comparative study of glutathione and ascorbate metabolism during germination of Pinus pinea L. seeds. J Exp Bot. 52(361):1647–1654. doi: 10.1093/jexbot/52.361.1647
- Tripathi DK, Singh S, Gaur S, Singh SW, Yadav V, Liu S, Singh VP, Sharma S, Srivastava P, Prasad SM, Dubey NK, Chauhan DK, Sahi S. 2018. Acquisition and homeostasis of iron in higher plants and their Probable role in Abiotic stress tolerance. Front Environ Sci. February, Volume 5, article 86.
- Vashisth A, Nagarajan S. 2010. Effect on germination and early growth characteristics in sunflower (Helianthus annuus) seeds exposed to the static magnetic field. J. Plant Physiol. 167:149–156. doi: 10.1016/j.jplph.2009.08.011
- Wang H, Kou X, Pei Z, Xiao JQ, Shan X, Xing B. 2011. Effects of magnetite (Fe3O4) nanoparticles on perennial ryegrass (Lolium perenne L.) and pumpkin (Cucurbita maxima). Plant Nanotoxicol. 5:30–42. doi: 10.3109/17435390.2010.489206
- Wang YH, Ying Y, Chen J, Wang XC. 2004. Transgenic Arabidopsis overexpressing Mn-SOD enhanced salt-tolerance. Plant Sci. 167:671–677. doi: 10.1016/j.plantsci.2004.03.032
- Zhang J, Cui S, Li J, Kirkham MB. 1995. Protoplasmic factors, antioxidant responses, and chilling resistance in maize. Plant Physiol Bioch. 33:567–575.
- Zhao J, Davis LC, Verpoorte R. 2005. . Elicitor signal transduction leading to the production of plant secondary metabolites. Biotechnol Adv. 23:283–333. doi: 10.1016/j.biotechadv.2005.01.003