ABSTRACT
Durian (Durio zibethinus L.) is susceptible to Phytophthora palmivora, which causes rot disease, leading to significant yield loss. This study aimed to investigate local defense responses of durian to P. palmivora and compare the infection dynamics of high virulence (HV) and low virulence (LV) isolates. Durian leaves on 1-year-old seedlings were inoculated with mycelial plugs. Symptoms were observed within 24 h. The HV-isolate was more aggressive, leading to larger lesions, earlier and denser colonization, and higher sporulation. P. palmivora lifestyle related gene (PpEF1α, PpHmp1, PpCdc14, and PpOPEL) were expressed at a higher level in HV compared to LV. Local defense response showed intense accumulation of H2O2, with a stronger response to the HV. Callose deposition increased initially but declined after inoculation, with different diameters between isolates, but no significantly different intensity patterns. These findings provide insights into the P. palmivora-durian interaction, with potential applications in improving disease management strategies.
Introduction
Phytophthora palmivora is a highly destructive pathogen that causes severe diseases in a broad range of economically important crops such as cocoa (Perrine-Walker Citation2020), citrus (Ahmed et al. Citation2014), rubber (Krishnan et al. Citation2019), oil palm (Torres et al. Citation2010), and durian (O’Gara et al. Citation2004). P. palmivora has been reported to have a hemibiotrophic lifestyle, which enables it to infect and overcome host defenses by first acting as a biotroph and subsequently switching to a necrotroph during the colonization and reproduction process (Fawke et al. Citation2015; Le Fevre et al. Citation2016; Evangelisti et al. Citation2017). A complex life cycle characterized by various stages of development, enabling it to successfully infect and colonize host plants (Judelson and Blanco Citation2005). The life cycle begins with the growth of mycelium, which is the vegetative stage of the organism, wherein the filamentous structures called hyphae extend and branch to explore the host tissue and acquire nutrients (Kamoun et al. Citation2015). Once P. palmivora establishes a compatible interaction with the host, it produces specialized infection structures called haustoria, which are formed by invaginations of the host cell membrane, allowing the pathogen to penetrate and directly access the host's nutrients (Whisson et al. Citation2007). As the infection progresses, P. palmivora undergoes sporulation, producing sporangia and motile zoospores that are released to the surrounding environment, where they can swim and infect new host tissues, propagating the infection cycle (Judelson and Blanco Citation2005). A critical component of P. palmivora's pathogenicity is the secretion of effector proteins, such as the OPEL (Oomycete PAMP Elicitor) protein, which modulates host defense responses and promotes pathogen virulence (Evangelisti et al. Citation2017). The intricate life cycle of P. palmivora, including stages of mycelial growth, haustoria formation, sporulation, and the secretion of effector proteins like OPEL, contributes to the pathogen's ability to cause devastating diseases in various plant species and highlights the need for effective management strategies to combat this destructive pathogen (Kamoun et al. Citation2015).
The hemibiotrophic lifestyle of P. palmivora makes it highly aggressive, particularly in the case of durian, leading to significant yield loss and high mortality in durian production in Thailand as well as other Southeast Asian countries (Phavakul and Changsri Citation1969; Drenth and Guest Citation2004; Somsri et al. Citation2008). Durian (Durio zibethinus L.) is a highly valued tropical fruit. It is widely cultivated in Southeast Asia, particularly in Thailand, which is the largest producer and exporter of durian in the world (GTA Citation2020). The main durian cultivation areas in Thailand are in the central, the east, and the south of the country. A suitable environment for growing durian requires a hot and humid climate, which is conducive for P. palmivora infection. Almost all commercial durian cultivars, especially Monthong, are susceptible to P. palmivora (Vawdrey et al. Citation2005a, Citation2005b). The high susceptibility of durian to P. palmivora emphasizes the need to study defense mechanisms and early responses of durian to this pathogen in order to improve durian crop production. Previous studies of P. palmivora-durian interaction mostly focused on pathogen virulence, biocontrol, and resistance to fungicides. However, disease progression and plant responses to the infection are less explored. Particularly, detailed comparisons between early responses of durian to low and high virulence pathogens have not been reported.
During pathogen infection, basal defense responses of plants play a crucial role in preventing pathogen invasion and can provide the plant with a first line of defense against pathogens. Understanding these mechanisms can help improve plant health and productivity, and lead to the development of more sustainable and effective plant protection strategies. One of the defense mechanisms utilized by pathogens is the deposition of callose, a plant polysaccharide, which serves as a physical barrier to prevent the pathogen from penetrating the plant cell wall. Callose is one of the most abundant components of cell wall finding in papillae, the cell wall apposition and forms as physical barrier in response against pathogens (Galletti et al. Citation2008; Luna et al. Citation2011; Ellinger and Voigt Citation2014). Additionally, local callose deposition is also induced by wounding and abiotic stress (Jacobs et al. Citation2003). For example, M. polymorpha deposited callose at the peripheral region at invading site response to P. palmivora haustoria-like structure at 3 dpi (Carella et al. Citation2018). However, agroinfiltration-induced callose deposition in tobacco was inhibited by RXLR effector, targeting at host’s callose synthases resulted in enhanced pathogen infection (Tomczynska et al. Citation2020).
Another key defense mechanism is the production of reactive oxygen species (ROS) such as hydrogen peroxide (H2O2), which plays crucial roles in plant defense as antimicrobial activity, cell wall strengthening and signal transduction (Arora et al. Citation2002; Foyer and Noctor Citation2003; Polkowska-Kowalczyk et al. Citation2007; Camejo et al. Citation2016). In addition, the stronger and faster ROS production at early infection followed by decreasing later, known as hypersensitive response (HR) which is necessary to prevent biotrophic and hemibiotrophic infection. In contrast, the high accumulation of ROS particularly at the late infection contributes to necrotrophic colonization (Bastas, 201; Noorbakhsh and Taheri Citation2016; El komy et al. Citation2020). For example, resistant oil palm infected by P. palmivora showed hydrogen peroxide (H2O2) accumulation and catalase activity increase within 24hpi (Ávila-Méndez et al. Citation2019).
The study of durian-P. palmivora interaction and durian defense responses is scant. There was only a report of O’Gara et al. (Citation2004) describing that trichomes on durian leaves acted as the first line of defense against P. palmivora zoospores, but hyphae could still penetrate stomata and wounds to cause infection. Durian deposits suberin, lignin and callose as defense responses to mechanical stress. Exogenous phosphonate induces durian leaf resistance to P. palmivora infection by activating phenylalanine ammonia-lyase (PAL). However, the plant's innate immunity defense strategies are still unclear compared to other P. palmivora host plants.
In order to accomplish an effective durian disease management, understanding durian-P. palmivora interaction and the durian innate immunity are necessary. In particular, the different dynamics of defense responses to low-virulence and high-virulence isolates should shed lights on how the infection might be better controlled. The objective of this manuscript is to investigate the interaction between durian and P. palmivora by characterizing disease progression, disease symptoms, and localized basal defense responses including hydrogen peroxide (H2O2) accumulation and callose deposition. Additionally, expression of P. palmivora lifestyle marker genes were evaluated. The ultimate goal is to improve our understanding of the durian innate immunity and to provide insights for effective durian disease management.
Materials and methods
Plant materials
Durian (Durio zibethinus L.) cv. Monthong, a commercial susceptible cultivar to Phytophthora palmivora was chosen to study. Durian seedlings were cultivated in plastic bags (6.5-inch diameter and 7.5-inch soil depth) until approximately 18 months old (100–120 cm in height and 4–6 branches). Seedlings were kept in a greenhouse under daytime temperature of 33–38°C and watering every day to maintain soil humidity. One day prior to starting experiment, durian seedlings were moved into a growth room with average temperature of 25–28°C. All inoculated leaves were covered with plastic bags to maintain high humidity and incubated in the growth room with 12 h Photosynthetic Photon Flux Density (PPFD) 2.5–3.0 µmol/m2/sec above the canopy.
Pathogen isolation and inoculation
Phytophthora palmivora isolates were selected from a culture collection held at the Department of Plant Science, Faculty of Science, Mahidol University. CbP03 and TrP01 isolates were chosen as the representative high-virulence (HV) and low-virulence (LV) isolates, respectively. The HV (CbP03) was isolated from a plantation in Chantaburi province, while the LV (TrP01) was isolated from Trat province, both located in Eastern Thailand. Both were isolated from infected durian bark tissues and further purified with single-spore isolation. On PDA, TrP01 and CbP03 showed similar growth rates, but caused clearly different necrotic lesion sizes, observable from 36 h post inoculation.
For pathogen inoculation, P. palmivora mycelium was subculture on V8 agar and incubated at 25°C for seven days prior to the experiment. Mycelial plugs with 5 mm diameter were cut and inoculated onto the leaves. Each inoculation site was wounded by pricking with a needle and inoculated with either a mycelial plug of P. palmivora isolate or a clean agar plug as a mock control. The first three fully expanded leaves were chosen from each branch; three branches were used per seedling, each for one of three assays: disease progression, H2O2 accumulation, and callose production. Seven seedlings were used for each experiment, one for each time point with six biological replicates per treatment per time point. All experiments were repeated three times independently. All inoculated leaves were covered with plastic bags to maintain high humidity and incubate in the growth room (). Treated leaf samples were collected at 0, 6, 12, 24, 36, 48 and 72 h post inoculation (hpi) and stored at −80°C.
Figure 1. Schematic representation of durian seedling treatments and sample collection. Durian seedlings at about one year old with at least three branches were performed for the experiment. For each seedling, the top branch was used for disease progression, the second for H2O2 accumulation, and the third for callose production analysis. Inoculation process was performed by puncturing each leaf with a sterile needle at six spots, and randomly applying mycelial plugs of P. palmivora (two spots for low virulence and two spots for high virulence) and two for mock control, randomly. Each treatment was collected at 6, 12, 18, 24, 36, 48, and 72 hpi. Each experiment used seven seedlings for a total of 63 leaves. All experiments were repeated three times independently.
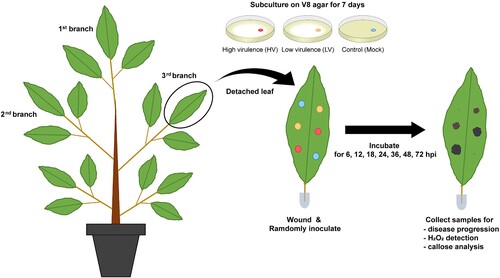
Disease progression and Calculation of disease scores
Disease development on durian leaves were measured from lesion diameters of three replicates per isolate on three separate leaves. Lesion sizes were evaluated from three independent experiments. Disease scores were evaluated on a scale of 0–4, of which 0 = 0–0.5 mm; 1 = 0.5–1 mm; 2 = 1–5 mm, 3 = 5–10 mm, and 4 = ≥ 10 mm as described by Latifah et al. (Citation2018).
RNA extraction, cDNA synthesis, and Gene expression analysis
Treated leaf samples were collected and stored at −80°C. Total mRNA was isolated using CTAB buffer (2% cetyltrimethyl ammonium bromide, 100 mM Tris-HCl pH 8, 25 mM EDTA pH 8, 2 M NaCl, 2% PVP 40, 0.2% 2-mercaptoethanol, 5 µg ml−1 spermidine). The first-strand cDNA synthesis was performed on 200 ng of total RNA using a cDNA synthesis kit (iScript™ Reverse Transcription Supermix, Bio-Rad), according to the manufacturer's recommendations.
Gene expression levels of the oomycete's lifestyle genes (PpEF1α, PpHmp1, PpCdc14, and PpOPEL) were analyzed using real-time, quantitative PCR. All real-time PCR reactions were performed using the ABI 7500 fast real-time PCR system (Applied Biosystems, USA) and the SYBR Green fluorescent dye (Kapa Biosystems, USA). Each sample was run for each primer pair (). Thermal cycling was performed in 96-well plates, with an initiation step at 95°C for 30 s, followed by 40 cycles of denaturation at 95°C for 5 s, extension at 60°C for 15 s, and a final melting curve analysis on each reaction to confirm the specificity of amplification. Relative quantification of gene expression was compared to two housekeeping genes (PpWS21 and PpACT). Gene expression was calculated as the relative expression ratio of each gene after treatment, following the Pfaffl method (Pfaffl Citation2001).
Table 1. Primers used in this study.
Sample staining and microscopy
Trypan blue staining
Trypan blue staining protocol was slightly modified from Keogh et al. (Citation1980). Briefly, the 20 × 20 mm pieces of inoculated tissue were cleared in EAG solution (95% EtOH: Acetic acid: Glycerol with 3:1:1 by volume) to remove chlorophyll, immersed in 50% EtOH for 5 min, and distilled water for 5 min. Subsequently, the leaf pieces were immersed in 0.5 mg/ml trypan blue solution (Lactic acid: Phenol: Glycerol: Distilled H2O with 1:1:1:1 by volume) for 30 min. Stained pieces were observed through a light microscope under 100X magnification.
DAB staining
DAB (3,3′ diaminobenzidine) staining protocol was modified from Thordal-Christensen et al. (Citation1997). Briefly, the pieces of inoculated tissue were immersed in DAB solution (1 mg/ml DAB in distilled H2O, pH 3.0) with vacuum-infiltration for 20 min before incubating overnight in DAB solution at room temperature in the dark. After incubation, tissues were moved into EAG solution and boiled at 90–95°C for 30 min, transferred into fresh EAG solution and incubated at room temperature until clear. The cleared samples were kept in EAG solution until observation.
Aniline blue staining
Aniline blue staining was performed as described by Currier (Citation1957) with slight modification. Briefly, the pieces of inoculated tissue were immersed in EAG solution until clear, and rehydrated in sodium phosphate buffer (Na2HPO4·2H2O, pH 9.0 adjusted by NaH2PO4·2H2O) for 30 min. After rehydration, samples were stained in 0.5% Aniline blue solution (0.5 g of Methyl blue dissolved in sodium phosphate buffer, pH 9.0) for 30 min. Stained pieces were observed using a fluorescence microscope with UV filter under 40X magnification.
Quantification using ImageJ software
ImageJ software was used for quantification of lesion diameter, pathogen colonization and sporulation, and staining intensity and diameter. For pathogen colonization and sporulation, images from trypan blue-stained tissues were cropped into a 1.3 × 1.3 mm2 image, manually adjusted for blue color threshold. Pathogen colonization was calculated from the total stained area subtracted by the average stained area in mock control. All observed sporangia and chlamydospores were manually counted. H2O2 production was indicated by the presence and distribution of dark brown precipitate after DAB staining. DAB staining intensity was measured using the circle selection tool and staining diameters using the free-hand selection tool. DAB intensity was determined separately between inner and outer rings by the mean gray value subtracted from the maximum gray value of 255. Total DAB staining diameters were measured from the longest line drawn across each stain. Callose deposition, indicated by the presence of fluorescence signals after aniline blue staining, were quantified from the captured images. Callose intensity was measured from three 0.1 × 0.1 mm2 randomly chosen areas within the callose ring using the mean gray value function without subtraction. Callose ring diameters were measured from the widest part of the fluorescent ring. All calculations were performed three times per replication, with three replications per treatment.
Statistical analyses
Results are expressed as the mean and standard error. The statistical analyses were performed using GraphPad Prism version 9.0.0 (GraphPad Software, CA, USA). The normality assumption was tested using the Shapiro–Wilk test, and the homogeneity of variance was tested using Levene's test. The statistical analysis of disease progression, H2O2 accumulation, and callose production for each treatment group was performed using one-way analysis of variance (ANOVA) with Tukey's multiple-comparison test. Kruskal–Wallis test with Dunn's multiple-comparison test was applied to assess the significant difference in the expression of the oomycete's lifestyle genes relative to the housekeeping genes, PpWS21 and PpACT. A p-value of less than 0.05 (p < 0.05) was considered statistically significant.
Results
Infection dynamics of low-virulence (LV) and high-virulence (HV) isolates
Both HV (CbP03) and LV (TrP01) isolates of Phytophthora palmivora were able to cause rot symptoms on durian leaves with different severity. Initially, no symptom was observed on leaves inoculated by both isolates. First observable symptom appeared at 24 h post-inoculation (hpi) only for HV isolate. From 36 hpi, dark-brown lesions were noticeable on both LH- and HV-inoculated leaves. Lesions caused by the HV isolate were visibly larger (4.84 ± 0.48 mm) than those caused by the LV isolate (1.21 ± 0.08 mm) ((A)). Lesions on mock-inoculated leaves were limited to the wounded sites.
Figure 2. Disease progression of Phytophthora palmivora isolates on durian leaves. The study used intact durian leaves inoculated with mycelial agar plugs of the isolates high virulence (HV), low virulence (LV), and a mock as a control. The inoculation was performed for 6, 12, 18, 24, 36, 48, and 72 hpi. Disease symptoms (A) and diameter of disease lesions (B) observed on durian leaves. Disease incidence, indicating distribution of each disease score in each scale per sampling unit (C). Disease lesion scores were assessed on a scale of 0 (0–0.5 mm), 1 (0.5–1 mm), 2 (1–5 mm), 3 (5–10 mm), and 4 (≥10 mm). Values are presented as the means ± SEM. Statistical significance between the treatment groups for each time point analyzed was determined using One-way ANOVA with Tukey's multiple comparison test (*p < 0.05, **p < 0.01).
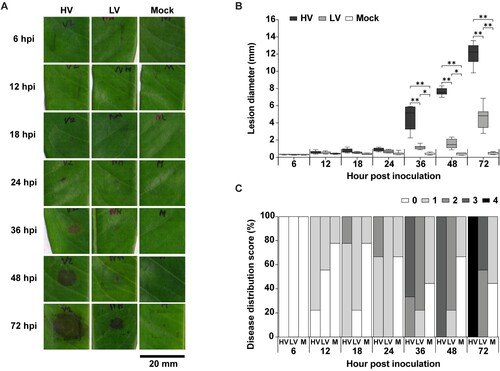
Lesions caused by both isolates continued to extend over the inoculated sites. HV isolate was more aggressive than LV isolate, causing 4-fold larger lesions at 72 hpi ((B)). Distribution of disease scores caused by HV and LV isolates was significantly different. At 12 hpi, disease scores were mostly 1 (0.5–1 mm) for HV. Disease score of 2 was first observed at 18 hpi for HV, and at 36 hpi for LV ((C)). At 48 hpi, all lesions caused by the HV isolate were scored as 3, and at 72 hpi, all lesions were scored as 4 (≥ 10 mm). Meanwhile, 56% lesions caused by LV isolate were scored as 2 and 44% as 3 at this time point. Taken together, these results affirmed that both HV and LV isolates were pathogenic in durian, although with different dynamics.
Successful colonization and sporulation
During D. zibethinus-P. palmivora interaction, hyphae of both HV and LV isolates were seldom observed until 12 hpi, but continually increased after prolonged infection. Durian leaves supported the colonization and growth of both isolates of P. palmivora during their interaction ((A)). At 24 hpi, hyphal growth on HV- and LV-inoculated leaves were clearly observed around the wounding site, with the lesion spreading into 0.09 ± 0.01 mm2 for HV and 0.07 ± 0.02 mm2 for LV. P. palmivora colonization by HV isolate was significantly denser by LV isolate, as seen by the trypan blue stained area, which was 1.6-fold higher at 48 hpi (HV: 0.16 ± 0.02 mm2, LV: 0.11 ± 0.02 mm2) ((B)).
Figure 3. Colonization and sporulation of Phytophthora palmivora on durian leaves. The leaves were inoculated with mycelial agar plugs of the high virulence (HV), low virulence (LV), and a mock as a control, and incubated for 6, 12, 18, 24, 36, 48, and 72 hpi. Oomycete structures, including mycelia, sporangia, chlamydospores, were detected using trypan blue (TB) staining and observed at 40X magnification which resulted in a dark blue color (A). Mycelia colonization (B), number of sporangia (C), and number of chlamydospores (D), were measured. Values are presented as the means ± SEM. Statistical significance between the treatment groups for each time point analyzed was determined using One-way ANOVA with Tukey's multiple comparison test (*p < 0.05, **p < 0.01).
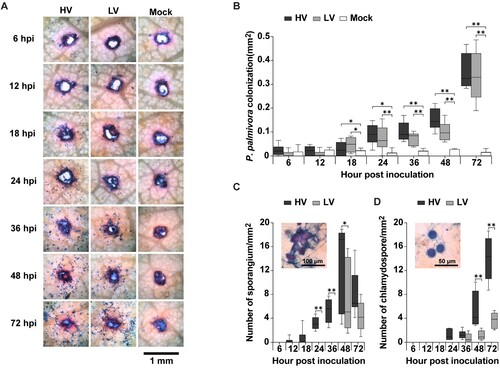
Sporangia of HV isolate were first observed at 12 hpi, prior to the initial occurrence of sporangia of LV isolate at 48 hpi. At 36 hpi, sporangia number of the HV isolate (4.87 ± 0.71 spores/mm2) was significantly greater than that of LV isolates by approximately 4-fold. Chlamydospores of HV isolate were first noticeable at 24 hpi, whereas in LV isolate it was not found until 36 hpi. Chlamydospore of HV isolate was also more prevalent than LV isolate by 4.4-fold at 48 hpi (HV:21.63 ± 3.89, LV: 04.87 ± 1.02 spores/mm2) ((C)). Notably, trypan blue generally stains for plant cell death as well as mycelium growth, but plant cell death was not clearly detected even after 72 hpi with both HV or LV isolates when necrotic lesion was clearly visible.
Evaluation of Phytophthora palmivora lifestyle markers
Expression levels of four genes (PpEF1α, PpHmp1, PpCdc14, and PpOPEL) associated with P. palmivora lifestyle in both HV and LV isolates during infection on detached durian leaves. The expression levels of these genes vary between the two isolates and across different time points (MZ, 0, 6, 12, 24, 36, 48, and 72 h). In general, the gene expression levels of PpEF1α, PpHmp1, and PpCdc14 except PpOPEL are higher in the high virulence strain compared to the low virulence strain. However, there are variations in gene expression levels of PpOPEL across different time points and genes. Level of the P. palmivora biomass marker gene (PpEF1α) significantly increased over time. Haustoria-associated marker gene (PpHmp1) expression in the HV isolate peaked earlier at 24 hpi, while the expression in the LV isolate peaked at 48 h. Sporulation-specific cell-cycle marker gene (PpCdc14) peaked at 48 hpi for the HV isolate, whereas in LV isolate it was not significantly induced. Interestingly, the expression of the oomycete Protein Elicitor-Like marker gene (PpOPEL) increased at early time points (6 hpi) in the LV isolate and then leveled off, while the expression in the HV isolate increased slightly at early time points and rapidly increased at later time points ().
Figure 4. Quantification of the oomycete's lifestyle genes via qRT-PCR analysis. The study inoculated the leaves with mycelial agar plugs of the high virulence (HV) and low virulence (LV), and incubated for 0, 6, 12, 24, 36, 48, and 72 hpi, while MZ represents 14 days old mycelia without inoculation. The genes analyzed were PpEF1α (A), PpHmp1 (B), PpCdc14 (C), and PpOPEL (D) relative to two housekeeping genes (PpWS21 and PpACT). Values are presented as the means ± SEM. Statistical significance between the treatment groups for each time point analyzed was determined using Kruskal-Wallis test with Dunn's multiple-comparison test (*p < 0.05, **p < 0.01).
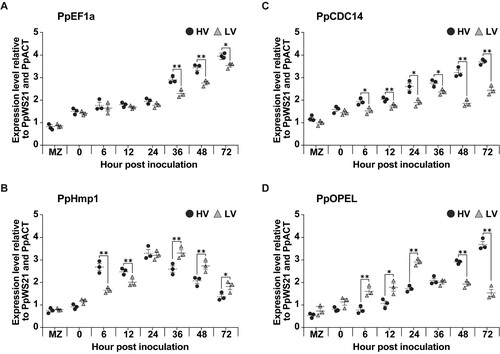
Evaluation of local defense response against low- and high-virulence isolates of Phytophthora palmivora in durian
Hydrogen peroxide accumulation
Oxidative burst is a rapid plant defense against pathogens involving the release of reactive oxygen species (ROS) such as hydrogen peroxide (H2O2). ROS-representing H2O2 were visualized by DAB staining as brown precipitation. Quantitative determination of H2O2 was performed through time intervals. Beginning at 6 hpi, staining could be observed around the wounded sites of both mock-inoculated and P. palmivora-inoculated leaves. In contrast to the constant H2O2 levels in mock-inoculated leaves, H2O2 accumulation continually expanded as a halo beyond the lesion sites in both HV- and LV-inoculated leaves ((A)).
Figure 5. Hydrogen peroxide (H2O2) production in durian leaves in response to P. palmivora infection. The leaves were inoculated with mycelial agar plugs of the high virulence (HV), low virulence (LV), and a mock as a control, and incubated for 6, 12, 18, 24, 36, 48, and 72 hpi. H2O2 production was detected using 3,3’-diaminobenzidine (DAB) staining which resulted in a dark brown precipitate (A). The total diameter of DAB staining was measured (B), and two separate patterns were observed: a high intensity inner area and a low intensity outer area (C). Values are presented as the means ± SEM. Statistical significance between the treatment groups for each time point analyzed was determined using One-way ANOVA with Tukey's multiple comparison test (*p < 0.05, **p < 0.01).
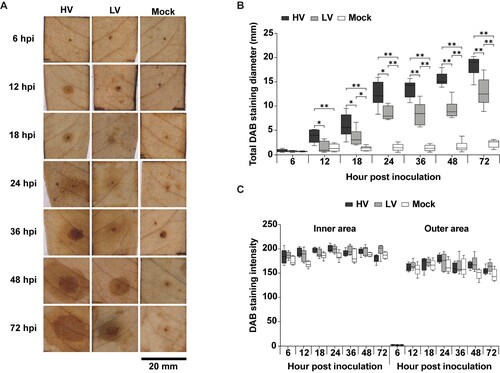
The diameter refers to the total DAB staining diameter of hydrogen peroxide production, which indicates the intensity of reactive oxygen species (ROS) accumulation in response to the infection. Hydrogen peroxide production increases over time and is affected by both P. palmivora infection and needle wounding. DAB staining diameters on HV-inoculated leaves were significantly larger than those on LV-inoculated leaves by about 4-fold at 12 hpi, with mean diameters of 4.99 ± 0.42 mm and 1.26 ± 0.51 mm, respectively. However, the diameters of DAB-stained areas at later time points consecutively increased in both HV and LV-inoculated leaves, and their ratios were reduced to approximately 1.5-fold, remaining stable until 72hpi (HV: 18.39 ± 1.22 mm, LV: 12.94 ± 1.58 mm) ((B)). Interestingly, DAB staining of both HV- and LV-inoculated leaves displayed two separate patterns: high intensity inner ring and low intensity outer ring ((C–D)) However, no statistically significant difference was observed in DAB intensity patterns among HV-and LV-inoculated leaves. Taken together, these results demonstrated that rapid and intense ROS accumulation rapidly occurred in response to P. palmivora inoculation. However, these responses were not sufficient to limit P. palmivora invasion.
Callose deposition
Another active defense mechanism that potentially prevents pathogen invasion is callose deposition. The results show that callose deposition occurred in response to both HV and LV inoculation, as well as needle wounding. Callose deposition in durian leaves were detected as a ring-shape fluorescent signal beyond the wounded site at 6 hpi. During 72 hpi, a callose ring was constant over the wounded area of mock-inoculated leaves, whereas callose of both HV- and LV-inoculated leaves continually expanded around the lesion site. There was no available data for callose deposition over 36 hpi for HV-inoculated leaves, the callose ring extended beyond the observable area under the microscope ((A)).
Figure 6. Callose deposition in durian leaves in response to P. palmivora infection. The leaves were inoculated with mycelial agar plugs of the high virulence (HV), low virulence (LV), and a mock as a control, and incubated for 6, 12, 18, 24, 36, 48, and 72 hpi. Callose deposition was detected by aniline blue staining, which appeared as a ring-shaped fluorescent signal under the microscope with an ultraviolet (UV) filter (A). The total expanding diameter of the callose ring (B) and callose ring intensity (C) were measured around the perforated site on a leaf area of about 3.5 × 2.5 mm2.‘N.d.’ indicates no available data, meaning that the callose ring extended beyond the observable area under the microscope. Values are presented as the means ± SEM. Statistical significance between the treatment groups for each time point analyzed was determined using One-way ANOVA with Tukey's multiple comparison test (*p < 0.05, **p < 0.01).
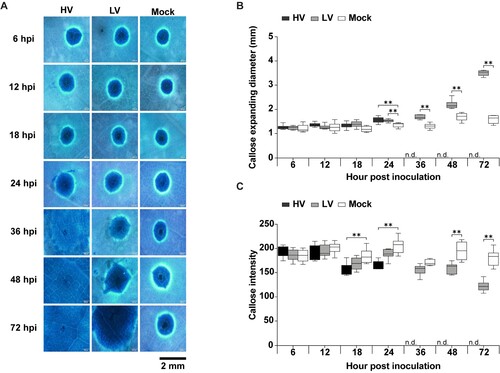
Both HV- and LV-inoculated leaves had larger callose deposition compared to mock-inoculated leaves, with HV-inoculated leaves generally having a slightly larger diameter than LV-inoculated leaves, although the difference was not statistically significant at all time points. At 24 hpi, there was a significant increase in callose deposition in HV- and LV-inoculated leaves compared to mock, with HV showing a slightly higher diameter than LV. However, for LV-inoculated leaves, there was a significant increase in callose deposition over 36 hpi compared to mock-inoculated leaves ((B)). Additionally, the callose intensity at all time points of the LV-inoculated leaves had slightly higher intensity values compared to the HV-inoculated leaves, although the difference was insignificant at all time points. From 36 hpi onwards, LV-inoculated leaves had a significant increase in callose intensity compared to mock, whereas there was no data available for HV-inoculated leaves ((C)). These results indicated that durian could induce rapid and intense callose deposition in response to wounding and to P. palmivora. However, even combined with the H2O2 production, callose deposition was still not sufficient in limiting P. palmivora invasion.
Discussions
This research proposed to lay the groundwork to understand durian-P. palmivora interaction, specifically the local and early responses. Infection dynamics, lifestyle marker gene expression, and host defense responses to high-virulence (HV) and low-virulence (LV) isolates were characterized and compared. Previous research showed that the virulence of P. palmivora isolates could depend on several factors, including the source tissue of pathogen isolation and host plants. For instance, isolates from durian root and trunk canker were shown to be more aggressive than the fruit isolate (Vawdrey et al. Citation2005a, Citation2005b). Virulence of P. palmivora isolates from different hosts varied. Interestingly, almost all isolates under cross-pathogenicity were shown to cause larger lesions than on the native host (Latifah et al. Citation2018). In this study, the HV and LV isolates were both obtained from the durian trunk cankers. Yet, their virulence differed significantly, and it was unclear at which stage of infection and to what extent.
Our results showed that although both the HV and LV could cause rot symptoms, the HV isolate displayed a more aggressive behavior, causing larger lesions and faster disease progression. The difference between lesions caused by HV and LV could be observed as early as 24 hpi. Similarly, high-virulence isolates of P. palmivora could cause necrotic lesion by 24 hpi on coconut and pear fruit (Mohamed-Azni et al. Citation2017; Karyath Palliyath et al. Citation2021). Both HV and LV isolates similarly generated dark-brown necrotic lesions, whereas isolates of P. palmivora did not only cause different lesion sizes, but also different lesion colors, either light-brown or dark-brown, on oil palm (Mohamed-Azni et al. Citation2017).
Successful colonization was observed during the interaction between durian leaves and both isolates of P. palmivora. However, the HV isolate displayed a significantly earlier and denser colonization than the LV isolate, further highlighting the differences in their pathogenicity. The differences between rates of colonization by HV and LV were observed even though the two strains exhibited similar growth rates on PDA. Thus, the differences seem to be host-dependent. Since the inoculation was performed with an agar plug with little observable sporulation, it was assumed that the differences were due to mycelial growth and not the germination or germination tube growth process. Perhaps, the HV isolate had a higher ability to dodge, overcome, or suppress plant defense and/or it was better at extracting nutrients from the host plants.
Sporulation was found as early as 12 hpi for the HV isolate, compared to 48 h for the LV isolate. HV isolate also produced chlamydospores with approximately four times higher density compared to LV. Similarly, a previous study by Taylor and Grünwald (Citation2021) also reported that most high virulence Phytophthora sp., pathogens on Rhododendron leaves, had high ability to sporulate, although some species could cause large lesions with low sporulation. The early and dense sporulation of the HV isolate could be an indirect result from its high ability to extract nutrients from the host and grow rapidly and/or additional mechanism(s) that directly enhanced its reproduction efficiency.
The investigation of lifestyle marker genes confirmed morphological observations that the HV isolate had a more accelerated lifestyle, including earlier expression of PpHmp1, the haustoria marker. Levels of the P. palmivora biomass marker PpEF1α significantly increased over time. Haustorium-associated marker gene (PpHmp1) peaked at initial stage while the cell-cycle regulator marker gene Cdc14 increased at later stages. PpEF1a and PpCdc14 marker genes were expressed at higher levels in the HV isolate. The expression levels of these marker genes were normalized to the expression levels of the housekeeping gene PpACTIN and PpWS21 in each isolate, which should not depend on the isolate density and growth rate. Therefore, it appeared that the HV isolate not only had a more accelerated growth and development, but also had more vigor in each hypha. These findings are consistent with the previous studies that the timing and nature of lifestyle transitions during P. palmivora infection can vary depending on the host plant. These findings suggest that P. palmivora exerts a hemibiotrophic lifestyle in different plant hosts (Evangelisti et al. Citation2017; Carella et al. Citation2018). Interestingly, expression patterns of the PpOPEL (Oomycete PAMP Elicitor) were different in the LV and HV isolates. In the LV isolate, the gene expression increased early and then leveled off, while in the HV isolate, expression increased slightly early on, followed by a rapid increase at later time points. This difference in expression patterns could indicate different roles of PpOPEL in the infection process and/or differences in the plant response. However, the exact function and mechanism of PpOPEL in P. palmivora infection is not yet fully understood and further research is needed to gain more insight.
Local defense responses of durian leaves against P. palmivora isolates such as hydrogen peroxide (H2O2) accumulation and callose deposition were detected, confirming the ability to perceive the pathogen and/or pathogen-induced damage. ROS such as H2O2 play important role as early signaling molecules activating downstream defense mechanisms as well as having antimicrobial activity, which is directly toxic to invading pathogens, especially biotrophs and hemibiotrophs (Torres et al. Citation2006; Bastas Citation2015; Sarria et al. Citation2016). H2O2 accumulation was observed around the wounded sites in both HV- and LV-inoculated leaves, with larger areas of accumulation in HV-inoculated leaves. Callose deposition was also observed in response to both isolates of P. palmivora. Although there were differences in callose ring diameters, the intensity of the callose rings induced by HV and LV isolates were not significantly different. Interestingly, the HV isolate showed either a reduced or depleted wound-induced callose deposition upon challenge with P. palmivora, with no noticeable expansion in intensity. Both the H2O2 and callose responses expanded, presumably as the edge of the infection extended, but did not adjust the intensity according to the virulence of the attacking isolate. Nonetheless, these defense responses were not sufficient to limit P. palmivora invasion.
Previous studies have shown that H2O2 intensity is related to its concentration, and interestingly, the H2O2 intensity in wounds was higher than that induced by the pathogen (Carril et al. Citation2020). In addition, increased activity of ROS scavenging enzymes, such as ascorbate peroxidase, catalase, peroxidase, glutathione reductase, and superoxide dismutase, were observed in the interaction between P. palmivora and oil palm with high disease symptoms (Moreno-Chacón et al. Citation2013). Callose is one of the most abundant components of cell wall acting as a physical barrier in response to pathogens (Luna et al. Citation2011; Ellinger and Voigt Citation2014). Nakashima et al. (Citation2003) reported that the response of callose deposition differed between wounding and P. palmivora infection in mung bean. Wounding induced a rapid and strong intensity of callose deposition early on, forming a contracted expanding ring around the wound site. In contrast, no distribution of elevated callose was observed in response to P. palmivora infection.
A diverse array of P. palmivora-secreted effector proteins, which includes RxLR, CRN, and CWDEs could suppress plant defense (Carella et al. Citation2018). Blackman et al. (Citation2014) showed that secreted glycosyl hydrolases could cause plant cell wall damage, one of which was β−1,3-glucanase that promoted callose degradation in plant cell wall during P. parasitica infection (Lee et al. Citation2011). Tomczynska et al. (Citation2020) also demonstrated that RxLR3 effectors of P. brassicae could inhibit callose deposition in Arabidopsis by specifically targeting callose synthase. Although a β−1,3-glucanase protein was induced and greatly accumulated in P. palmivora-inoculated rubber leaves, the accumulation levels in the resistant and susceptible clones were not different (Sunpapao and Pornsuriya Citation2016). However, effectors employed during P. palmivora-durian interaction have not been reported.
In conclusion, this study provides valuable insights into the interaction between durian leaves and P. palmivora isolates with varying virulence levels. The differential infection dynamics, colonization, and sporulation of HV and LV isolates, as well as the local defense responses of durian, highlight the complexity of the host–pathogen interaction. Despite the rapid and intense ROS production and callose deposition as defense responses, these mechanisms were not sufficient to protect durian leaves from P. palmivora infection. These findings emphasize the need for a better understanding of the P. palmivora virulence mechanisms and host defense to develop more effective strategies for durian cultivation, ultimately helping to maintain its high economic value and ensuring long-term productivity.
Acknowledgements
The authors acknowledge all durian plantation owners for providing research materials. We are grateful to Thomas Neal Stewart for his suggestions and proofreading of the manuscript. We thank to Sunipa Detpitthayanan, Woranuch Bunnag, Wanichaya Chaiwimol, and Yotin Juprasong for technical help. Author contributions: NP designed the study, performed experiments, processed the data, interpreted the results, and write manuscript; US performed experiments, processed the data, interpreted the results, prepare data visualization, and write manuscript; SP interpreted the results and write manuscript; JN and KS supervised the study, reviewed and edited the manuscript; AP and WS provided conceptualization, supervised the study, and reviewed and edited the manuscript All authors have read and approved the final version of the manuscript.
Additional information
Funding
Notes on contributors
Nussara Putaporntip
Nussara Putaporntip received her M.Sc. degree in Plant Science from Mahidol University in 2014. Her thesis focused on rice interaction with plant growth- promoting bacteria (PGPB). She is currently pursuing her Ph.D. at Mahidol University. Her research interests include plant-microbe interactions, plant physiology, especially about plant stresses.
Umaporn Siriwattanakul
Umaporn Siriwattanakul received her M.Sc. degree in Plant Science from Mahidol University with the focus on respiratory allergies triggered by pollen, especially allergies to Amaranth pollen in Thailand. She is currently pursuing her Ph.D. in Biotechnology. Her research focused on plant pathology and plant-microbe interaction.
Supawadee Phetkhajone
Supawadee Phetkhajone received her M.Sc. degree in Plant Science from Mahidol University with the focus on the development of metalaxyl detection methods and assessment of dissipation kinetics in durian. She is currently pursuing her Ph.D. in Biology. Her research focused on development of RNA-based bio-fungicide for sustainable farming.
Jarunya Narangajavana
Jarunya Narangajavana is an associate professor at Department of Biotechnology, Faculty of Science, Mahidol University. Her research interests include epigenetic control in plants, plant functional genomics study for industrial applications, plant biochemical and molecular genetics related to yield and stress responses.
Kanyarat Supaibulwatana
Kanyaratt Supaibulwatana achieved her Ph.D. in Plant Biotechnology from Chiba University, Japan, in 1997. Currently, she is an academic staff in the Department of Biotechnology, Faculty of Science, Mahidol University. She serves in the Agricultural Biotechnology group, contributing significantly to plant science research with her expertise in plant responses to environmental stress, biosynthesis of plant secondary metabolites, and bioengineering and mutation technologies for plant breeding.
Aussanee Pichakum
Aussanee Pichakum has been a lecturer in the Plant Sciences program at Mahidol University, Thailand since 1996. She completed her Ph.D. in Plant Science at Chiba University, Japan, following her B.Sc. and M.S. degrees in Agriculture from Kasetsart University, Thailand. Her research interest is expanding into the role of plant bioregulators and cultural management to improve yield and fruit quality of kiwifruits longan and durian in Thailand.
Wisuwat Songnuan
Wisuwat Songnuan received the B.S. degree in biology from Duke University, Durham, NC, USA, in 2002 and the Ph.D. degree in Genetics from Harvard Medical School and Graduate School of Arts and Sciences, Cambridge, MA, USA, in 2009. After her Ph.D., she became a faculty member at the Department of Plant Science, Faculty of Science, Mahidol University, Bangkok, Thailand. Her research interests include plant genetics and molecular biology, plant-microbe interaction, and plant-related allergies.
References
- Ahmed Y, D'Onghia AM, Ippolito A, Yaseen T. 2014. First report of citrus root rot caused by Phytophthora palmivora in Egypt. Plant Dis. 98(1):155. doi:10.1094/PDIS-02-13-0206-PDN.
- Arora A, Sairam RK, Srivastava GC. 2002. Oxidative stress and antioxidative system in plants. Curr Sci. 82(10):1227–1238. https://www.jstor.org/stable/24107045.
- Ávila-Méndez K, Avila-Diazgranados R, Pardo A, Herrera M, Sarria G, Romero HM. 2019. Response of in vitro obtained oil palm and interspecific OxG hybrids to inoculation with Phytophthora palmivora. For Path. 49(2):e12486. doi:10.1111/efp.12486.
- Baştaş K. 2015. Importance of reactive oxygen species in plants-pathogens interactions. Selcuk J Agr Food Sci. 28(1):11–21. https://dergipark.org.tr/en/pub/selcukjafsci/issue/21054/226668.
- Blackman LM, Cullerne DP, Hardham AR. 2014. Bioinformatic characterisation of genes encoding cell wall degrading enzymes in the Phytophthora parasitica genome. BMC Genom. 15:785. doi:10.1186/1471-2164-15-785.
- Camejo D, Guzmán-Cedeño Á, Moreno A. 2016. Reactive oxygen species, essential molecules, during plant–pathogen interactions. Plant Physiol Biochem. 103:10–23. doi:10.1016/j.plaphy.2016.02.035.
- Carella P, Gogleva A, Tomaselli M, Alfs C, Schornack S. 2018. Phytophthora palmivora establishes tissue-specific intracellular infection structures in the earliest divergent land plant lineage. Proc Natl Acad Sci. 115(16):E3846–E3855. doi:10.1073/pnas.1717900115.
- Carril P, Da Silva AB, Tenreiro R, Cruz C. 2020. An optimized in situ quantification method of leaf H2O2 unveils interaction dynamics of pathogenic and beneficial bacteria in wheat. Front. Plant Sci. 11:889. doi:10.3389/fpls.2020.00889.
- Currier HB. 1957. Callose substance in plant cells. Am J Bot. 44(6):478–488. doi:10.1002/j.1537-2197.1957.tb10567.x.
- Drenth A, Guest DI. 2004. Diversity and management of Phytophthora in Southeast Asia. Australian Centre for International Agricultural Research Monograph, 114, Australia, 238p.
- El Komy MH, Saleh AA, Ibrahim YE, Molan YY. 2020. Early production of reactive oxygen species coupled with an efficient antioxidant system play a role in potato resistance to late blight. Trop Plant Pathol. 45(1):44–55. doi:10.1007/s40858-019-00318-8.
- Ellinger D, Voigt CA. 2014. Callose biosynthesis in Arabidopsis with a focus on pathogen response: what we have learned within the last decade. Ann Bot. 114(6):1349–1358. doi:10.1093/aob/mcu120.
- Evangelisti E, Gogleva A, Hainaux T, Doumane M, Tulin F, Quan C, Yunusov T, Floch K, Schornack S. 2017. Time-resolved dual transcriptomics reveal early induced Nicotiana benthamiana root genes and conserved infection-promoting Phytophthora palmivora effectors. BMC Biol. 15:39. doi:10.1186/s12915-017-0379-1.
- Fawke S, Doumane M, Schornack S. 2015. Oomycete interactions with plants: infection strategies and resistance principles. Microbiol Mol Biol Rev. 79(3):263–280. doi:10.1128/MMBR.00010-15.
- Foyer CF, Noctor G. 2003. Redox sensing and signalling associated with reactive oxygen in chloroplasts, peroxisomes and mitochondria. Physiol Plant. 119:355–364. doi:10.1034/j.1399-3054.2003.00223.x.
- Galletti R, Denoux C, Gambetta S, Dewdney J, Ausubel FM, De Lorenzo G, Ferrari S. 2008. The AtrbohD-mediated oxidative burst elicited by oligogalacturonides in Arabidopsis is dispensable for the activation of defense responses effective against Botrytis cinerea. Plant physiol. 148(3):1695–1706. doi:10.1104/pp.108.127845.
- Global Trade Atlas. 2020. Average market share of global durian market 2017–2019; [accessed 2020 Apr 7]. https://www.gtis.com/gta/.
- Jacobs AK, Lipka V, Burton RA, Panstruga R, Strizhov N, Schulze-Lefert P, Fincher GB. 2003. An Arabidopsis callose synthase, GSL5, is required for wound and papillary callose formation. Plant Cell. 15(11):2503–2513. doi:10.1105/tpc.016097.
- Judelson HS, Blanco FA. 2005. The spores of Phytophthora: weapons of the plant destroyer. Nat Rev Microbiol. 3:47–58. doi:10.1038/nrmicro1064.
- Kamoun S, Furzer O, Jones JD, Judelson HS, Ali GS, Dalio RJ, Roy SG, Schena L, Zambounis A, Panabières F, et al. 2015. The Top 10 oomycete pathogens in molecular plant pathology. Mol Plant Pathol. 16(4):413–434. doi:10.1111/mpp.12190.
- Karyath Palliyath G, Kilingar Subrahmanya M, Antony G, Binod Bihari S, Hegde V, Muliyar Krishna R. 2021. A rapid in vitro leaf inoculation assay to investigate Phytophthora palmivora–coconut interactions. J Phytopathol. 169(5):316–328. doi:10.1111/jph.12988.
- Keogh RC, Deverall BJ, McLeod S. 1980. Comparison of histological and physiological responses to Phakopsora pachyrhizi in resistant and susceptible soybean. Trans Br Mycol Soc. 74(2):329–333. doi:10.1016/S0007-1536(80)80163-X.
- Krishnan A, Joseph L, Roy CB. 2019. An insight into Hevea – Phytophthora interaction: the story of Hevea defense and Phytophthora counter defense mediated through molecular signalling. Curr Plant Biol. 17:33–41. doi:10.1016/j.cpb.2018.11.009.
- Latifah M, Kamaruzaman S, Abidin MZ, Nusaibah SA. 2018. Identification of Phytophthora spp. from perennial crops in Malaysia, its pathogenicity and cross-pathogenicity. Sains Malays. 47(5):909–921. doi:10.17576/jsm-2018-4705-06.
- Lee JY, Wang X, Cui W, Sager R, Modla S, Czymmek K, Zybaliov B, van Wijk K, Zhang C, Lu H, Lakshmanan V. 2011. A plasmodesmata-localized protein mediates crosstalk between cell-to-cell communication and innate immunity in Arabidopsis. Plant Cell. 23(9):3353–3373. doi:10.1105/tpc.111.087742.
- Le Fevre R, O'Boyle B, Moscou MJ, Schornack S. 2016. Colonization of barley by the broad-host hemibiotrophic pathogen Phytophthora palmivora uncovers a leaf development-dependent involvement of Mlo. Mol Plant-Microbe Interact. 29(5):385–395. doi:10.1094/MPMI-12-15-0276-R.
- Luna E, Pastor V, Robert J, Flors V, Mauch-Mani B, Ton J. 2011. Callose deposition: a multifaceted plant defense response. Mol Plant Microbe Interact. 24(2):183–193. doi:10.1094/MPMI-07-10-0149.
- Mohamed-Azni INA, Sundram S, Ramachandran V, Abu Seman I. 2017. An in vitro investigation of Malaysian Phytophthora palmivora isolates and pathogenicity study on oil palm. J Phytopathol. 165(11-12):800–812. doi:10.1111/jph.12620.
- Moreno-Chacón AL, Camperos-Reyes JE, Ávila Diazgranados RA, Romero HM. 2013. Biochemical and physiological responses of oil palm to bud rot caused by Phytophthora palmivora. Plant Physiol Biochem. 70:246–251. doi:10.1016/j.plaphy.2013.05.026.
- Nakashima J, Laosinchai W, Cui X, Malcolm Brown R. 2003. New insight into the mechanism of cellulose and callose biosynthesis: proteases may regulate callose biosynthesis upon wounding. Cellulose. 10:369–389. doi:10.1023/A:1027336605479.
- Noorbakhsh Z, Taheri P. 2016. Nitric oxide: a signaling molecule which activates cell wall-associated defense of tomato against Rhizoctonia solani. Eur J Plant Pathol. 144:551–568. doi:10.1007/s10658-015-0794-5.
- O’Gara E, Sangchote S, Fitzgerald L, Wood D, Seng A, Guest DI. 2004. Infection biology of Phytophthora palmivora Butl. in Durio zibethinus L. (Durian) and responses induced by phosphonate. In: Drenth A, Guest DI, editors. Diversity and management of Phytophthora in Southeast Asia. 114:42–52.
- Perrine-Walker F. 2020. Phytophthora palmivora-cocoa interaction. J Fungi. 6(3):167. doi:10.3390/jof6030167.
- Pfaffl MW. 2001. A new mathematical model for relative quantification in real-time RT–PCR. Nucleic Acids Res. 29(9):45e–e45. doi:10.1093/nar/29.9.e45.
- Phavakul K, Changsri V. 1969. Root rot of durian tree. Proceedings of a seminar on plant protection. Thai J Agric Sci. 60–61.
- Polkowska-Kowalczyk L, Wielgat B, Maciejewska U. 2007. Changes in the antioxidant status in leaves of Solanum species in response to elicitor from Phytophthora infestans. J Plant Physiol. 164(10):1268–1277. doi:10.1016/j.jplph.2006.08.012.
- Sarria GA, Martinez G, Varon F. 2016. Histopathological studies of the process of Phytophthora palmivora infection in oil palm. Eur J Plant Pathol. 145:39–51. doi:10.1007/s10658-015-0810-9.
- Somsri S, Vichitrananda S, Kengkat P, Koonjanthuk P, Chunchim S, Sesuma S, Salakphet S. 2008. Three decades of durian breeding program in Thailand and its three newly recommended F1 hybrids. Acta Hortic. 787:77–88. doi:10.17660/ActaHortic.2008.787.6.
- Sunpapao A, Pornsuriya C. 2016. Overexpression of β-1, 3-glucanase gene in response to Phytophthora palmivora infection in leaves of Hevea brasiliensis clones. Walailak J Sci Tech. 13(1):35–43. doi:10.14456/WJST.2016.4.
- Taylor CR, Grünwald NJ. 2021. Growth, infection and aggressiveness of Phytophthora pathogens on Rhododendron leaves. CABI Agric Biosci. 2(1):1–15. doi:10.1186/s43170-021-00048-5.
- Thordal-Christensen H, Zhang Z, Wei Y, Collinge DB. 1997. Subcellular localization of H2O2 in plants. H2O2 accumulation in papillae and hypersensitive response during the barley—powdery mildew interaction. Plant J. 11(6):1187–1194. doi:10.1046/j.1365-313X.1997.11061187.x.
- Tomczynska I, Stumpe M, Doan TG, Mauch F. 2020. A Phytophthora effector protein promotes symplastic cell-to-cell trafficking by physical interaction with plasmodesmata-localised callose synthases. New Phytol. 227(5):1467–1478. doi:10.1111/nph.16653.
- Torres GA, Sarria GA, Varon F, Coffey MD, Elliott ML, Martinez G. 2010. First report of bud rot caused by Phytophthora palmivora on African oil palm in Colombia. Plant Dis. 94(9):1163. doi:10.1094/PDIS-94-9-1163A.
- Torres MA, Jones JDG, Dangl JL. 2006. Reactive oxygen species signaling in response to pathogens. Plant Physiol. 141(2):373–378. doi:10.1104/pp.106.079467.
- Vawdrey L, Langdon P, Martin T. 2005a. Incidence and pathogenicity of Phytophthora palmivora and Pythium vexans associated with durian decline in far northern Queensland. Australas Plant Pathol. 34(1):127–128. doi:10.1071/AP04093.
- Vawdrey LL, Martin TM, De Faveri J. 2005b. A detached leaf bioassay to screen Durian cultivars for susceptibility to Phytophthora palmivora. Australas Plant Pathol. 34(2):251–253. doi:10.1071/AP05005.
- Whisson SC, Boevink PC, Moleleki L, Avrova AO, Morales JG, Gilroy EM, Armstrong MR, Grouffaud S, van West P, Chapman S, et al. 2007. A translocation signal for delivery of oomycete effector proteins into host plant cells. Nature. 450(7166):115–118. doi:10.1038/nature06203.