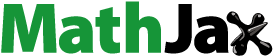
ABSTRACT
The integration of nanoparticles (NPs) into agriculture is altering traditional methods, enhancing productivity and sustainability. This study explores the application of iron oxide nanoparticles (FeONPs) to mitigate salt-alkaline stress in cherry tomatoes. We investigated FeONPs at three concentrations (FeONP25, FeONP50, FeONP100 mg/kg soil) in pot experiments under non-stress (NS) and salt-alkaline stress (SAS) conditions. SAS conditions decreased biomass and nutrients in untreated plants, a trend reversed by FeONPs. FeONPs treatments significantly boosted pigment levels under SAS, thereby increasing chlorophyll a (10.65–43.05%), chlorophyll b (7.19–41.33%), total chlorophyll (9.84–42.49%), and carotenoids (8.97–36.09%) compared to the control. FeONPs also reduced NPQ under stress, indicating enhanced photosynthetic efficiency. Oxidative stress markers (H2O2, O₂−, and MDA) were strongly induced in control plants but significantly declined with FeONPs treatments. Antioxidants and osmoregulatory substances significantly improved with FeONPs, thereby demonstrating their potential to alleviate SAS in cherry tomato plants.
Introduction
Saline-alkaline stress is an increasingly recognized abiotic factor that has significant negative impacts on agricultural productivity and ecological equilibrium at a global level, particularly in regions characterized by arid and semiarid climates (Bai et al. Citation2018). The most common types of soil salts include sodium chloride (NaCl), anhydrous sodium sulphate (Na2SO4), sodium carbonate (Na2CO3), and sodium bicarbonate (NaHCO3). NaCl and Na2SO4 are categorized as neutral salts, while Na2CO3 and NaHCO3 are considered alkaline salts. Plants react more severely to salinity-alkalinity stress than to salt stress alone, primarily owing to the elevated pH levels (Li and Yang Citation2023). The growing predominance of salty and alkaline soils, which is a consequence of the expansion of irrigated regions (Yin et al. Citation2023), presents a complex challenge to the growth and physiology of plants. Around 37% of the world's arable land is classified as sodic and 23% as saline. The SAS is expected to have a significant impact on approximately half of the world's irrigated land, or 2.5 × 108 ha, according to various sources (Sahab et al. Citation2021; Rao et al. Citation2023). Unregulated human activities, contamination of irrigated water, and climatic changes are some of the main factors contributing to a serious threat to arable land by causing saline-alkalization.
The current scientific knowledge emphasizes that SAS greatly disrupts plant growth, development and physiology through a complex interplay involving reduced water and energy fluxes, ionic toxicity, and osmotic stress (Hu et al. Citation2022; Yin et al. Citation2023). A direct precipitation of important ions including Ca2+, Mg2+, and H2PO4− is directly attributed to the concomitant increase in pH, which is responsible for this heightened impact (Sagervanshi et al. Citation2021). This precipitation, on the other hand, causes disruptions in the systems that are responsible for ion absorption and the availability of mineral nutrients, which in turn makes metabolic disorders more severe (Sagervanshi et al. Citation2021).
Studies have also demonstrated that SAS significantly reduces plant photosynthesis, induces photoinhibition, thus negatively affecting plant growth and development (Nie et al. Citation2018; Wang et al. Citation2021). An impaired photosynthetic efficiency and a compromised ability of photosystem II (PSII) to convert light energy into chemical energy during the process of photosynthesis are indicated by a decrease in the net photosynthetic rate (Pn) and the maximum quantum efficiency of PSII (Fv/Fm) due to the presence of saline-alkaline stress (Zhang et al. Citation2019b). Further, the non-photochemical quenching (NPQ) is a very crucial factor which dissipates excitation energy and decreases strain on the PSII reaction center in stressed plants. One of the significant factors contributing to the rise of photoinhibition in plants under high SAS is the suppression of NPQ (Huihui et al. Citation2020). The adverse effects of SAS on the content of photosynthetic pigments, attributed to structural damages of the grana lamellae and thylakoids within the chloroplasts, have also been reported (Zhang et al. Citation2019b).
Furthermore, oxidative stress and high pH during saline-alkalization cause cellular structures to be disrupted and increase the generation of reactive oxygen species (ROS), which ultimately results in ROS-associated damage in plant cells (Yang et al. Citation2022). To keep ROS levels in check, plants rely on their antioxidant defense mechanism. In most cases, superoxide dismutase (SOD) is responsible for converting superoxide anions (O2•−) into hydrogen peroxide (H2O2) (Wang et al. Citation2018). Catalase (CAT), the ascorbate-glutathione (AsA-GSH) cycle, and other antioxidants and antioxidases play a crucial role in regulating the subsequent conversion of H2O2 into water and oxygen (Dumanović et al. Citation2021). Biomolecule oxidation, such as lipid peroxidation, protein degradation, and the oxidation of individual DNA and RNA nucleotides, along with enzyme inhibition and apoptosis activation, may occur in the absence of a sufficient concentration of antioxidants to neutralize ROS (Hasanuzzaman et al. Citation2020). Moreover, the build-up of ROS molecules not only exerts detrimental effects on the cell and its components but also serves as activators of signaling pathways to initiate biological processes (Dumanović et al. Citation2021). Several studies have also highlighted the differential expression pattern of antioxidant genes during the combined effect of salt and alkaline stress (Jin et al. Citation2019; Hou et al. Citation2023), contributing to the fine-tuning of ROS-mediated responses.
Nanotechnology offers innovative approaches to enhance stress tolerance and thus help achieving sustainable agricultural goals. Nanoparticles (NPs) have high surface area, mobility, reactivity, and particle shape, making them a promising tool for sustainable agriculture (Al-Khayri et al. Citation2023). Evidence shows that different types of NPs can cross cellular barriers, making them appropriate for maximizing growth and development (Wang et al. Citation2023b). Under stress conditions, the ameliorating effects of different NPs have been extensively reported. For instance, titanium dioxide NPs (TiO2NPs) significantly boost wheat (Triticum aestivum) growth and biomass under drought stress by maintaining membrane integrity, nutrient absorption, and hormonal balance (Mustafa et al. Citation2021). Application of cerium oxide NPs (CeONPs) decreased drought-induced oxidative damage in sorghum (Sorghum bicolor) leading to higher photosynthesis and grain yield (Djanaguiraman et al. Citation2018a). Zinc oxide nanoparticles (ZnONPs) alleviate cadmium stress in lettuce (Lactuca sativa L.) by modulating phenylalanine content, phenylalanine ammonia lyase activity, and lignin content, while also reducing the translocation of cadmium (Gao et al. Citation2022). The selenium NPs (SeNPs) alleviated the negative impacts of high-temperature stress by enhancing antioxidant enzyme activities in grain sorghum (Djanaguiraman et al. Citation2018b). Chitosan NPs (ChNPs) efficiently mitigated drought-induced oxidative stress in Catharanthus roseus by accumulating proline, enhancing the activity of CAT and ascorbate peroxidase (APX), and regulating key alkaloid biosynthetic genes (Ali et al. Citation2021).
Similarly, evidence shows that FeONPs can be applied to boost the plant growth of peanuts (Arachis hypogaea), a crop highly sensitive to iron (Fe) deficiency (Rui et al. Citation2016). However, a shortcoming of this study was its failure to explore the potential application of FeONPs under given stress conditions. Nonetheless, recent studies demonstrate that FeONPs could mitigate the negative impact of various stress factors, including heavy metals (Hussain et al. Citation2019; Manzoor et al. Citation2023), saline stress (Moradbeygi et al. Citation2020; Tawfik et al. Citation2021), drought stress (Alabdallah et al. Citation2021), and water stress (Waqas Mazhar et al. Citation2022). To date, the potential role of FeONPs in mitigating the effects of SAS has not yet been investigated.
Cherry tomatoes (Lycopersicon esculentum var. cerasiforme) have gained widespread popularity due to their petite size, subtle flavor profile, and exceptional fruit characteristics. Recently, several studies have explored the use of various NPs and/or their biocomposites for preserving cherry tomatoes as a postharvest technique (Xiang et al. Citation2021; Zhang et al. Citation2021; Wu et al. Citation2022; Zheng et al. Citation2022). However, only a few studies have directly applied different NPs to alleviate the adverse effects of abiotic stress factors in cherry tomato plants. These studies include the use of ZnONPs (Gutiérrez-Miceli et al. Citation2021; Almendros et al. Citation2022), SeNPs (Neysanian et al. Citation2021), and TiO2NPs (Choi Citation2021). To date, no studies have characterized the direct application of FeONPs in mitigating SAS, a major yield-limiting factor, in cherry tomatoes.
With a focus on utilizing FeONPs as treatments, this study aimed to investigate their impact on cherry tomato plants under SAS conditions by examining various aspects including growth and biochemical parameters, photosynthetic capacity, and antioxidant potential. To accomplish this objective, we assessed (a) plant growth parameters, (b) macro- and micronutrient content, (c) photosynthesis rate and efficiency, (d) oxidative damage, (e) cellular redox status, (f) total phenolic content, and (g) total flavonoid content, thereby providing insights into the effects of these treatments on cherry tomato plants.
Material and methods
Plant material and growth conditions
The cherry tomato seeds from the ‘Red Ruby’ cultivar were obtained from a local commercial nursery due to its known genetic uniformity, robustness, and popularity among growers, ensuring that our experimental results would be representative and relevant. Additionally, consistent growth characteristics and response to treatments under greenhouse conditions have also been documented for this cultivar (Ahmad et al. Citation2023). The seeds were sown (1/4 inch deep) in nursery bags (15 × 20 cm) filled with a mixture of peat and vermiculite (1:1 v/v). Seeds were first disinfected with sodium hypochlorite (5%) for about 8–10 min, and subsequently washed five times with deionized water. Seeds were allowed to germinate under long-day conditions (∼16 h light photoperiod), 26°C average temperature, 130 µmol m−2 s−1 photosynthetic active radiation (PAR), and nearly 80% relative humidity in a closed glasshouse. When seedlings produced 3–4 true leaves, plants of uniform size were transferred to plastic containers (16 cm width, 14 cm height, and 11 cm bottom) filled with a mixture of soil, peat, and sand (2:1:1 by volume). The potted soil was analyzed, revealing the following fundamental physical and chemical characteristics: pH level of 6.58, organic matter content at 4.19%, alkaline hydrolyzed nitrogen concentration of 118.24 mg⋅Kg−1, available phosphorus content measuring 34.68 mg⋅kg−1, and available potassium content at 190.14 mg⋅kg−1. The cherry tomato plants were allowed to grow in a greenhouse (with relatively stable day/night temperature (25–27°C), and 16/8 light/dark periods) until the end of the experiments. Thirty pots were prepared for each treatment and seedlings were watered uniformly every alternate day until treatments were initiated.
Treatments and experimental design
The NPs (Fe3O4; 97% purity) in the form of black nanopowder was purchased from Sigma-Aldrich (Shanghai, China). According to specifications, Fe3O4 nanopowder had a particle size of 80–100 nm (SEM), an estimated surface area of 6–8 m2/g, and bulk density of 0.94 g/mL.
After one week of seedling growth under greenhouse conditions, the seedlings were treated with FeONPs as pre-treatments. For the application of NPs, a typical soil drench method was used, wherein FeONPs were mixed with distilled water and directly supplied to the soil in pots once per week with uniform distribution at three concentration levels as follows: (i) FeONP25 (25 mg/kg soil), (ii) FeONP50 (50 mg/kg soil), and (iii) FeONP100 (100 mg/kg soil). The control plants were irrigated only with distilled water. After one week of nanoparticle application, the plants were divided into two groups: one group without salt-alkaline stress (NS) and the other group subjected to salt-alkaline stress (SAS).
One week after the nanoparticle applications, stress treatment was exclusively applied to plants in the SAS group, while FeONP treatments continued simultaneously in both groups. For stress treatment, cherry tomato seedlings were irrigated every second day (for a total of 14 days) with 50 mL of 200 mM saline–alkaline mixed solution (2.5 ml 200 mmol/L NaCl, 22.5 ml 200 mmol/L Na2SO4, 22.5 ml 200 mmol/L NaHCO3, and 2.5 ml 200 mmol/L Na2CO3 (pH = 8.90)), as described by others with little modification (Xu et al. Citation2022). The experiment was setup as a randomized complete block design with three replicates for each treatment. Samples were collected from all treatment groups the day after the termination of the stress treatment for downstream physiological, biochemical, and gene expression analyses.
Measuring seedlings growth and biomass
Five plants were randomly selected from each treatment of both groups (NS and SAS). The samples were first gently cleaned with the help of clean toilet papers to remove bulk soil particles. The shoot and root lengths were measured using a measuring tape (cm), and fresh biomass was weighed immediately after harvesting with a digital scale with an accuracy of 0.01 g. Subsequently, samples were dried in an oven at 70°C to determine dry biomass.
Determination of pigments contents
Fresh leaf samples (0.6 g) were obtained from treated plants of both groups (NS and SAS), and extracted by grinding the samples in freshly prepared 80% acetone solution (5 mL). Samples were then incubated in a dark room at 26°C for nearly twelve hours. A UV/VIS spectrophotometer (DU800, Beckman, USA) was used to measure the absorbance of 1 mL extracts at 663 nm for chlorophyll a, 645 nm for chlorophyll b, and 470 nm for carotenoids, as described in our previous study (Shahzad et al. Citation2023). The final calculations were performed using the following equations to measure chlorophyll a, chlorophyll b, total chlorophyll, and carotenoids contents:
(1)
(1)
(2)
(2)
(3)
(3)
(4)
(4) where V is the volume for acetone extract (in mL) and W is the fresh weight of the sample in grams (g).
Determination of macro- and micronutrients
For nitrogen analysis, dry shoot and root samples (0.2 g) were carefully measured on parchment paper. These samples were then placed into a 250 mL glass tube alongside two tablets of 1000 Kjeltabs Se/3.5, while a separate glass tube, containing only parchment paper with one tablet, served as a blank reference. To initiate digestion, a digestion mixture (H2SO4–H2O2) was introduced into each tube. The digestion process took place with the help of a continuous flow auto analyzer (VELP Scientifica, Italy), operating at 420°C for a duration of 2 h. Following the cooling of the samples, the auto Kjeldahl machine (Kjeltec 2300, FOSS, Denmark) was employed to analyze the total nitrogen content.
To analyze other macro- and micronutrients, 0.2 g of the dried sample was meticulously weighed and placed in a 100 mL Erlenmeyer flask. Subsequently, 5 mL of nitric acid was added to the flask, and the sample was left to stand at room temperature overnight. Digestion was then carried out on a hot plate at 140°C under a fume hood until approximately 1 mL of nitric acid remained, and the absence of visible organic residue was confirmed. Following digestion, the samples were allowed to cool at room temperature and then diluted to a volume of 50 mL with deionized water. The resulting diluted sample underwent filtration using a 0.45 μm syringe filter. Based on emitted wavelengths to identify and quantify the elements present in the sample, the final concentrations of elements were determined through analysis using an inductively coupled plasma-optical emission spectrometer (ICP-OES, Avio 500, PerkinElmer, USA), with calibration performed using a standard solution of known concentrations.
Measurement of photosynthetic parameters
The third fully expanded leaves, avoiding the main vein, were used from both control and treated plants in the NS and SAS groups to assess Pn and stomatal conductance (Gs) between 9:30 am and 12:00 pm. Utilizing a portable photosynthesis system (Li-6400; LI-COR Biosciences, USA), measurements were conducted in a controlled chamber set at a constant temperature of 25°C, with an air velocity of 500 mol/s, a CO2 concentration of 400 μmol/mol, and a fixed LED light source emitting 600 μmol photons m m−2 s−1. Before analysis, the chosen leaves were subjected to diverse irradiation levels for a duration of 4–5 min, allowing for the stabilization of the CO2 uptake curve, after which data were systematically gathered.
For fluorescence-related parameter measurement, seedlings underwent a 30-minute dark incubation before analysis. Chlorophyll fluorescence parameters, such as Fv/Fm and NPQ, were analyzed on leaves using a dual portable fluorometer (Dual-PAM-100, Effeltrich, Germany) at room temperature and under a saturating pulse. Measurements were performed at two different points from 5 plants and average values were calculated. Refer to the detailed protocol outlined in a prior report (Zhang et al. Citation2019a) for a comprehensive understanding of the analysis procedure.
Determination of H2O2, O2•−, and MDA levels
For the H2O2 measurement, samples were collected from both control and treated plants from the NS and SAS groups. Each sample, weighing 0.4 g, underwent crushing and subsequent homogenization with 5 ml of 0.1% trichloroacetic acid (TCA), followed by centrifugation at 12,000 × g for 15 min. Post-centrifugation, approximately 0.5 ml of the supernatant was collected, and a mixture of 1 mL of 1 M potassium iodide and 0.5 ml of 10 mM phosphate buffer was added. The H2O2 content was ultimately determined by measuring absorbance at 390 nm using a spectrophotometer and represented in nmol g−1 FW, as described in our previous study (Shahzad et al. Citation2023).
To quantify O2•−, samples weighing 0.4 g were homogenized with 1 ml of 65 mM Bis(2-pyridyl) sulfide (BPS, pH 7.8) and subsequently subjected to centrifugation at 10,000 × g for 10 min. The resulting supernatant was transferred to a new tube, and then 75 µL of BPS along with 25 µL of 10 mM hydroxylamine hydrochloride were added, followed by a 20-minute incubation at room temperature. Subsequently, 1 ml of the supernatant was combined with 1 ml of 7 mM α-naphthalene diamine hydrochloride and 1 ml of 17 mM α-sulphanilamide. To complete the process, 3 ml of ether was introduced, and the mixture was centrifuged at 5000 × g under room temperature. The absorbance was measured at 540 nm using a spectrophotometer for the final calculation of superoxide anion and represented in nmol g−1 FW, as described in our study (Shahzad et al. Citation2023).
To quantify malondialdehyde (MDA) content in leaf samples, the thiobarbituric acid (TBA) reaction was employed following a standardized protocol (Heath and Packer Citation1968). Samples weighing 0.4 g were extracted in 2 ml of a solution containing 0.25% TBA in 10% TCA. The resulting extract was subjected to heating at 95°C for 25 min, followed by rapid cooling on ice. Afterward, the extract was centrifuged at 10,000 g for 10 min, and the absorbance was measured at 532 nm for MDA content and represented in nmol g−1 FW.
Assay of enzymes activity
Frozen leaves weighing 0.6 grams were finely ground using a pre-chilled mortar to form a homogenate in extraction buffer: 5 mL of 50 mM phosphate buffer (pH 7.0) that contains 0.2 mM EDTA and 2% (w/v) polyvinylpyrrolidone (PVP). Subsequently, the homogenate underwent centrifugation at 4°C and 12,000 × g per minute for 20 min. The resulting supernatant was employed for assessing the antioxidant enzyme activities of SOD, CAT, and APX. Briefly, for the assessment of SOD (EC: 1.15.1.1) activity, a 3 mL reaction mixture was meticulously prepared. The mixture contained 50 mM phosphate buffer (pH 7.8), 13.0 μmol L−1 methionine, 63.0 μmol L−1 nitroblue tetrazolium (NBT), and 1.3 μmol L-1 riboflavin, combined with 100 μL of enzyme extract. In the final phase, riboflavin was introduced with gentle agitation, and the reaction proceeded for 10 min under a 15-watt fluorescent illumination. The SOD activity was quantified by assessing the inhibition of the photochemical reduction of NBT at 560 nm, following the methodology outlined by an earlier study (Sarker and Oba Citation2018). Likewise, to determine CAT (EC: 1.11.1.6) activity, 100 μL of enzyme extract was introduced into a one mL solution of potassium phosphate buffer. The reaction commenced with the addition of 100 μL of H2O2, and the absorbance was systematically recorded at 240 nm at 15-second intervals over a two-minute duration, following the procedure outlined in the earlier methodology by Sarker and Oba (Citation2018). The determination of APX (EC 1.11. 1.11) activity involved spectrophotometric measurement at 290 nm, assessing the decline in ascorbic acid content one minute after the introduction of H2O2. The assay was conducted at 25°C in a reaction mixture comprising 50 mM potassium phosphate (pH 7.0), 0.5 mM AsA (with an extinction coefficient of 2.8 mmol/cm), 0.1 mM H2O2, and 10 μL of enzyme extract, as described by Moradbeygi et al. (Citation2020).
Determination of ascorbic acid and glutathione contents
To quantify the concentration of ascorbic acid (AsA), fresh leaf samples weighing 0.5 g were collected. The extraction procedure involved using 3 mL of pre-chilled 5% metaphosphoric acid, added with 1 mM EDTA. Following extraction, the solution was subjected to centrifugation at 11,500 × g for a period of 15 min. The AsA content was finally measured by the reducing method as described by other authors (Wang et al. Citation2022a). In a parallel manner, glutathione (GSH) was extracted from leaf samples (0.5 g) using 5% (v/v) trichloroacetic acid containing 5 mM EDTA, and its determination was achieved through fluorometric methods (Wang et al. Citation2022a).
Measurement of proline, soluble sugars, and soluble proteins
The fully expanded leaf samples from five different plants were used for the proline determination using the method described by Lu et al. (Citation2023). Briefly, the samples were prepared by grinding in liquid nitrogen, and 90-100 mg of the resulting powdered tissue was homogenized in 1.5 mL of 3% sulphosalicylic acid (w/v). Following centrifugation at 12,000 × g for 5 min, 200 µL of the sample supernatant was combined with 200 µL of acetic acid and 200 µL of ninhydrin reagents. This mixture underwent incubation at 95°C for 60 min, with the reaction promptly halted by placing it on ice for 3–5 min. Subsequently, 600 µL of pure toluene was added into the mixture, and after gentle shaking the solution was incubated at room temperature for 30 min. The readings were taken at a wavelength of 520 nm with 10 readings per well using a microplate reader (TECAN Infinite 200 Pro, TECAN, Switzerland) in 96-well plastic plates. The proline content was determined using the standard curve method and expressed in µg·g−1 FW.
Soluble sugar content was assessed following the protocol outlined by Du et al. (Citation2020). Fresh leaves weighing 0.5 g were subjected to extraction in a boiling water bath for 30 min. The remaining residue underwent two additional extractions, and the combined extraction solutions were placed in a volumetric flask (100 mL). Subsequently, 0.5 mL of the extraction solution in a test tube was mixed with a cocktail comprising 0.5 mL of anthrone-ethyl acetate reagent, 5.0 mL of concentrated sulfuric acid, and 1.5 mL of distilled water. The test tube, upon shaking, was immediately immersed in a boiling water bath for 10 min. After cooling, the absorbance was measured three times using the UV3310 spectrophotometer (Hitachi, Japan) at a wavelength of 620 nm. The soluble sugar content was determined through a standard curve (using glucose as a reference) and expressed in mg·g−1 FW.
Soluble protein levels were assessed using the UV-3310 spectrophotometer (Hitachi, Japan) at 595 nm as described by other study (He et al. Citation2022). Firstly, leaf samples (2 g) were ground to homogenate in 5.0 mL of phosphate buffer, followed by centrifugation at 12,000 × g for 20 min at 4°C. The resulting supernatant (1.0 mL) was collected and introduced to a test tube containing 5.0 mL of Coomassie Brilliant Blue G-250 solution, maintained at 30°C for 30 min to determine soluble protein content. The absorbance of the reaction solution was measured at 595 nm using a spectrophotometer. Bovine serum albumin served as a standard for the quantitative analysis of soluble protein content, expressed in mg·g−1 FW.
Determination of total flavonoids and total phenolics
The determination of total phenolic content (TPC) was performed as described by Machado et al. (Citation2023), with minor adaptations. Firstly, a stock solution of gallic acid was prepared. Next, 20 µL of either the sample extract or the standard solution was mixed with 80 µL of Folin–Ciocalteu reagent (diluted in a 1:10 ratio, v/v), supplemented with 100 µL of 7.5% (w/v) sodium carbonate. After an incubation period of 60 min at room temperature in the absence of light, absorbance readings were taken at 750 nm. The findings are expressed as milligrams of gallic acid equivalents per gram of fresh weight (mg GAE/g FW).
The quantification of total flavonoids content (TFC) was conducted using the aluminum chloride method, as described in another study (Sarker and Oba Citation2018). In a concise manner, 40 µL of each extract was combined with 20 µL of 10% (w/v) aluminum chloride and 20 µL of 1 M potassium acetate solutions. The mixture was then brought to a final volume of 200 µL in a 96-well plate with the addition of distilled water. Following a 30-minute incubation in the absence of light at room temperature, the absorbance at 415 nm was measured using a microplate reader. The calibration curve was constructed using quercetin as a standard, and the concentration of flavonoids was determined in µg quercetin equivalents per gram of fresh weight (µg QE/g FW).
The antioxidant activity determined by DPPH and FRAP assays
The assessment of leaf samples antioxidant capacity was measured through two methods: the 2,2-diphenyl-1-picrylhydrazyl free radical (DPPH) scavenging capacity and ferric reducing antioxidant power (FRAP), as described by Wang et al. (2022). The extraction of fresh samples followed the same procedure employed for determining phenolic compounds as mentioned above. In the analysis of DPPH scavenging capacity, mixtures of the extracts and DPPH solution were incubated at 37°C for 30 min, and their absorbance was recorded at 515 nm. The DPPH scavenging capacity was quantified using the formula: DPPH scavenging activity (%) = 1 − (absorbance of sample/absorbance of control) × 100%.
For the FRAP analysis, the extracts were mixed with the FRAP reagent and allowed to maintain at room temperature for about 30 min. After measuring the absorbance at 593 nm, the results were calculated based on the calibration curve derived from the Fe2+ solution and expressed as μM Fe2+ per gram of fresh weight (μM Fe2+/g FW).
RNA extraction and expression analysis
RNA extraction from cherry tomato leaves was carried out using a RNA Reagent kit (Invitrogen) as per the manufacturer's instructions. Subsequently, cDNA synthesis was performed and a total volume mixture of 12 µL was prepared: 0.2-mL tube contained 1 µg of total RNA, 1 µL of 10 µM dT30 (2 µM), 1 µL of 10 mM dNTP (2 mM), and Milli-Q water up to a total volume. The reaction mixture was incubated at 60°C for 10 minutes. Later, 4 µL of 5 × First-Strand Buffer with 0.1 M DTT and 1 µL of RNase were also mixed, and the solution was incubated at 37 °C for about 5 min. Following this step, reverse transcriptase enzyme (1 µL of MMLV) was then added, and the reaction underwent incubation for 50 minutes at 37°C in the warm water bath. As a final step, the reaction was terminated by keeping this mixture solution at 70°C for at least 10 min. Now, the cDNA was diluted 1:20 and real-time PCR (qPCR) was performed in samples to analyze the expression of genes using a CFX96 machine (BioRad). The list of primers is provided in a supplementary file (Supplementary Table 1). For making a qPCR reaction mixture, a total volume of 10 µL was prepared as follows: 2 µL of cDNA (diluted), 5 µL of 2 × GoTaq qPCR Master Mix SYBR Green, 0.5 µL of each primer, and 2 µL of ddH2O. The elongation factor 1 alpha (EF1α) was used as an internal reference gene, and gene expression was performed by the relative quantification method (Schmittgen and Livak Citation2008).
Statistical analysis
The findings are presented as the mean ± standard deviation (SD) from a minimum of three repetitions. Statistical analysis involved a one-way analysis of variance (ANOVA) conducted with SPSS 23.0 (SPSS Inc., Chicago, USA). Differences among treatment means were assessed using Duncan’s multiple range test at a significance level of P < 0.05. Data visualization was carried out using the ggplot2 function in the open-source statistical program R (version 4.1.0) for Windows, accessible at http://CRAN.R-project.org/. To better understand the association between studied traits and experimental factors, Pearson’s correlation analysis was performed. The R package ‘corrplot’ was used to compute a matrix of correlation coefficients and distribution plots at significance levels (0.05*, 0.01**, and 0.001***). In the correlogram, positive correlations are displayed in blue and negative correlations in red.
Results
FeONPs improve plant growth under SAS
shows the seedling length and fresh and dry weights in control and FeONP treatments at different concentration levels (FeONP25, FeONP50, FeONP100) under SAS and NS conditions. In the context of NS conditions, the application of FeONPs demonstrated positive effects on the growth parameters of cherry tomato seedlings. The FeONP25 treatment showed no difference in shoot length and root length, while only a slight increase in shoot fresh weight (2.44%), shoot dry weight (5.79%), root fresh weight (4.31%), and root dry weight (5.88%) was observed (). The FeONP50 application resulted in moderate enhancements, with notable increases in shoot length (10.19%), shoot fresh weight (9.86%), shoot dry weight (21.01%), root fresh weight (18.10%), and root dry weight (108.82%). The FeONP100 treatment demonstrated the most significant improvements, highlighting its potential to promote plant growth, with substantial increases of 16.60% in shoot length, 15.98% in shoot fresh weight, 38.40% in shoot dry weight, 23.27% in root fresh weight, and 126.47% in root dry weight.
Figure 1. Measurement of plant growth, fresh and dry biomass accumulation under NS and SAS in control (without treatments) and FeONP treatments. (A) Shoot length, (B) root length, (C) shoot fresh weight, (D) shoot dry fresh, (E) root fresh weight, and (F) root dry weight. The data represent the mean values obtained from three separate experiments, each conducted independently, with error bars indicating standard errors. Bars marked with distinct letters denote significant differences, as determined by the Duncan test (p < 0.05).
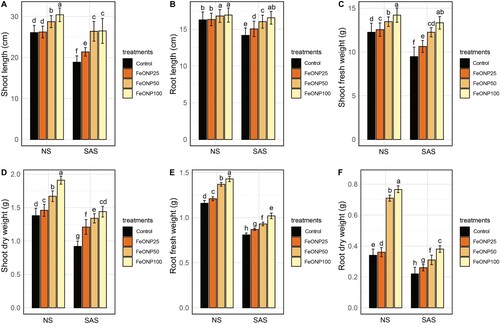
Plants subjected to SAS showed a significant decline in growth indices in the absence of FeONPs treatment (). However, the application of FeONPs effectively mitigate the detrimental effects of SAS on growth parameters. All treatments enhance the shoot and root attributes under SAS conditions in a dose-dependent manner (). In comparison to the control group, the FeONP25 treatment exhibited a moderate increase in shoot length (13.24%), root length (6.21%), shoot fresh weight (12.23%), shoot dry weight (31.52%), root fresh weight (7.42%), and root dry weight (18.21%) (). However, both FeONP50 and FeONP100 consistently outperformed during SAS conditions and displaying significant increase in growth attributes that included shoot length (39.92%, 40.43%), root length (13.11%, 16.85%), shoot fresh weight (29.32%, 40.71%), shoot dry weight (45.65%, 56.52%), root fresh weight (14.81%, 25.92%), and root dry weight (40.91%, 72.64%), respectively, compared to control ().
FeONPs boost photosynthetic pigments under SAS
The influence of FeONPs on photosynthetic pigments under SAS and NS conditions were examined (). In the absence of stress (NS), a positive impact of FeONP treatments on chlorophyll a, chlorophyll b, total chlorophyll, and carotenoid contents was noticed at all concentrations levels compared to control. The SAS caused a sharp decrease in the pigment contents of untreated seedlings: chlorophyll a, chlorophyll b, total chlorophyll, and carotenoid contents decreased by 27.91%, 24.84%, 28.61%, and 30.14%, respectively, in contrast with their corresponding control (). On the other hand, the application of FeONP treatments to tomato seedlings at all concentration levels under SAS conditions led to the restoration of these pigments compared to untreated control. In response to FeONP25 treatment under SAS conditions: chlorophyll a, chlorophyll b, total chlorophyll, and carotenoid contents increased by 10.65%, 7.19%, 9.84%, and 8.97%, respectively. For the FeONP50 treatment, the positive trend continued, revealing significant improvements in chlorophyll a (38.97%), chlorophyll b (32.06%), total chlorophyll (36.71%), and carotenoid (25.64%) contents compared to control under the similar conditions (). At the highest concentration of FeONP100, a remarkable percentage increase of 43.05%, 41.33%, 42.49%, and 36.09% for chlorophyll a, chlorophyll b, total chlorophyll, and carotenoid contents, respectively, was observed as compared to untreated control (). These findings underscore the progressive impact of FeONPs on augmenting photosynthetic pigments, suggesting their potential utility in strengthening plant responses against SAS conditions.
Figure 2. Determination of pigment contents in plants under NS and SAS in control (without treatments) and FeONP treatments. (A) chlorophyll a, (B) chlorophyll b, (C) total chlorophyll, and (D) carotenoids in tomato seedlings. The data represent the mean values obtained from three separate experiments, each conducted independently, with error bars indicating standard errors. Bars marked with distinct letters denote significant differences, as determined by the Duncan test (p < 0.05).
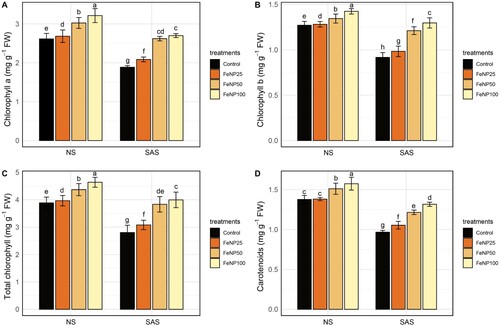
FeONP treatments improve photosynthetic parameters under SAS
Under NS conditions, FeONP treatments gradually increased Pn and Gs as the concentration of FeONP increased (A and B). The Fv/Fm was significantly increased only at FeONP50 and FeONP100, while FeONP25 did not show any significant changes with respect to the control under similar conditions (C). In the case of NPQ under NS conditions, there was a slightly decreasing trend in response to FeONP treatments, and there was no significant difference among the treatments at all concentration levels (C).
Figure 3. Determination of photosynthetic parameters in plants under NS and SAS in control (without treatments) and FeONP treatments. (A) Net photosynthetic rate (Pn), (B) stomatal conductance (Gs), (C) maximum efficiency of PSII (Fv/Fm), and (D) non-photochemical quenching (NPQ) in tomato plants. The data represent the mean values obtained from three independent experiments with error bars indicating standard errors. Bars marked with distinct letters denote significant differences, as determined by the Duncan test (p < 0.05).
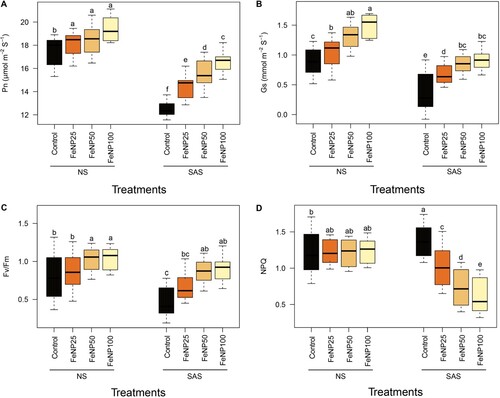
Under SAS conditions, Pn, Gs, and Fv/Fm declined by up to 28.06%, 44.04%, and 32.91%, respectively, in untreated control plants compared to NS conditions (). Interestingly, we observed higher NPQ values, up to 24.77%, in untreated control plants during SAS vs. NS conditions (D). Furthermore, there were more obvious differences between the control and treatment groups in SAS conditions. For the FeONP25 treatment during SAS conditions, a significant increase of 17.98%, 10.63%, and 20.75% in Pn, Gs, and Fv/Fm, respectively, was observed compared to the control. At higher concentrations, Pn, Gs, and Fv/Fm values were further enhanced (for the FeONP50 treatment; 25.89%, 34.04%, 64.15%, and at FeONP100; 33.01%, 51.06%, 71.69%, respectively) compared to the control under similar conditions (). In contrast, we observed a decreasing trend for NPQ values in a dose-dependent manner in response to FeONP treatments. The NPQ values showed a marked decline at FeONP25, FeONP50, and FeONP100 (27.65%, 48.22%, and 58.86%, respectively) compared to untreated control under SAS conditions (D).
Changes in macro- and micronutrients in response to FeONP and SAS
FeONP treatments significantly changed the nutrient contents of macro- and micronutrients in both the shoots and roots of cherry tomato under NS and SAS conditions ( and ). Under NS conditions, the application of FeONPs at all concentration levels increased the contents of macronutrients N, Mg, and Ca in shoots and roots in a dose-dependent manner, with higher concentrations of FeONPs leading to greater increases compared to the control. The levels of macronutrients K, P, and S increased in shoots but gradually declined in the roots as the concentration of FeONPs increased (see ), indicating their dynamic distribution in response to these treatments.
Table 1. Effect of FeONPs and salt-alkaline stress on macronutrients in cherry tomato.
Table 2. Effect of FeONPs and salt-alkaline stress on micronutrients in cherry tomato.
In response to SAS conditions, the contents of macronutrients N, K, Mg, and S were significantly decreased in both shoots and roots of untreated control plants. However, the contents of P and Ca decreased in shoots but increased significantly in the roots of untreated control plants, showing an opposite distribution in the shoot and root system under SAS conditions (). In contrast, FeONP treatments at all levels significantly recovered the contents of all macronutrients in shoots and roots in a dose-dependent manner compared to the control, except for P, which showed a declining trend as the concentration of FeONPs increased under SAS conditions (see ).
Regarding micronutrients, except for Mn, which decreased in shoots but increased in roots, all other nutrient elements (Zn, Fe, and Cu) markedly declined in shoots and roots of control plants under SAS conditions (). Conversely, the application of FeONP treatments at all levels enhanced the contents of micronutrients Fe and Cu in both shoots and roots, while Zn and Mn declined in roots but increased in shoots of treated plants in a dose-dependent manner compared to untreated control under similar conditions (). Overall, FeONP treatments at all levels showed a significant improvement in nutrient elements in both the shoots and roots of tomato seedlings, particularly under SAS.
Modulation of ROS and MDA by FeONP treatments under SAS
Under NS conditions, a slight decrease in the contents of H₂O₂, O₂⁻, and MDA was noticed with the application of FeONP treatments at all concentration levels (A–C). Furthermore, more noticeable differences were observed between control and treatment groups in SAS conditions. Overall, SAS increased the accumulation of these parameters in all treatment groups. However, the contents of H₂O₂, O₂⁻, and MDA were found to be significantly lower in FeONP treatments than in the untreated control.
Figure 4. Determination of changes in oxidative stress indicators in control and FeONP-treated plants under NS and SAS. (A) Hydrogen peroxide (H2O2), (B) superoxide anion (O2•−), and (C) malondialdehyde (MDA). The data represent the mean values obtained from three independent experiments with error bars indicating standard errors. Bars marked with distinct letters denote significant differences, as determined by the Duncan test (p < 0.05).
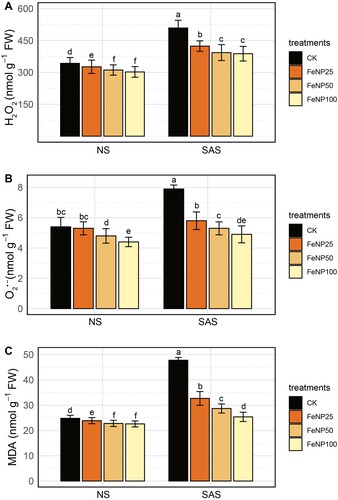
In response to SAS conditions, H₂O₂, O₂⁻, and MDA levels increased by 48.51%, 46.29%, and 92.74%, respectively, in untreated control plants compared to NS conditions (). For the FeONP25 treatment, the levels of H₂O₂, O₂⁻, and MDA decreased by 16.74%, 26.58%, and 31.46%, respectively, during SAS conditions compared to the untreated control (A–C). At higher concentrations, H₂O₂, O₂⁻, and MDA values were further decreased (for the FeONP50 treatment; 22.71%, 32.91%, 40.06%, and at FeONP100; 23.76%, 38.04%, 46.82%, respectively) compared to the untreated control under similar conditions (A–C).
FeONP regulate the activity of antioxidant enzymes and related genes expression under SAS
Based on the differential response of oxidative stress indicators in control and FeONP treatments in cherry tomato, next, we measured the activity of antioxidant enzymes (SOD, CAT, and APX) to gain deeper insights into the potential mechanisms underlying the observed differences. Under both conditions (NS and SAS), the activities of SOD, CAT, and APX were enhanced by FeONP treatments in a dose-dependent manner compared to untreated control (A). Overall, we observed an increase in antioxidant enzyme activities in untreated control plants as well as in response to FeONP treatments, albeit with varying levels of activity.
Figure 5. Measurement of antioxidant enzymes and related gene expression in tomato seedlings under NS and SAS in control (without treatments) and FeONP treatments. (A) Activity of antioxidant enzymes (CAT, SOD and APX), and (B) expression profile of related nine gene isoforms (CAT1, CAT2, CAT3, SOD1, SOD3, SOD4, APX1, APX2, APX3). The data represent the mean values obtained from three separate experiments, each conducted independently, with error bars indicating standard errors. Bars marked with distinct letters denote significant differences, as determined by the Duncan test (p < 0.05).
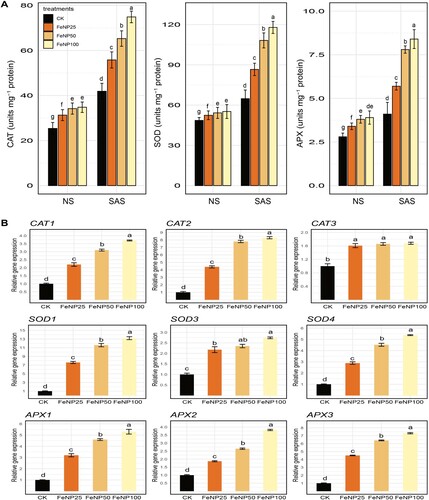
For the FeONP25 treatment, the levels of SOD, CAT, and APX increased by 32.68%, 33.17%, and 39.02%, respectively, under SAS conditions compared to the untreated control under similar conditions. At FeONP50, the levels of SOD, CAT, and APX were increased up to 64.57%, 55.84%, and 90.24%, respectively, under SAS conditions compared to the control. At higher concentration of FeONP100, the levels of SOD, CAT, and APX were further increased up to 81.94%, 78.65%, and 104.87%, respectively, under SAS conditions compared to the control (A). Similarly, we confirmed the expression of nine gene isoforms (CAT1, CAT2, CAT3, SOD1, SOD3, SOD4, APX1, APX2, and APX3) associated with these enzymes during SAS conditions in treatment groups (B). Consistently, we found upregulation in the expression of these genes in response to FeONP25, FeONP50, and FeONP100 compared to the control (B). Overall, these results showed that the antioxidant enzyme activities of SOD, CAT, and APX reached their maximum levels at the concentration of FeONP100 compared to the other treatments.
FeONP treatments improve ascorbate and glutathione levels under SAS
To further analyze the redox status of control and FeONP-treated cherry tomato plants, we measured the contents of non-enzymatic antioxidants (ascorbate; AsA, and glutathione; GSH) under both NS and SAS conditions (). Under NS conditions, there was a slight increase in AsA contents due to FeONP treatments; however, no significant difference was found between FeONP50 and FeONP100 treatments (A). Under SAS conditions, AsA levels were increased by 14.51%, 49.38%, and 62.46% in response to FeONP25, FeONP50, and FeONP100, respectively, compared to untreated control under similar conditions (A).
Figure 6. Determination of changes in redox status of glutathione and ascorbate in tomato leaves in control and FeONP-treated plants under NS and SAS. (A) Ascorbate content (AsA), and (B) glutathione content (GSH). The data represent the mean values obtained from three independent experiments with error bars indicating standard errors. Bars marked with distinct letters denote significant differences, as determined by the Duncan test (p < 0.05).
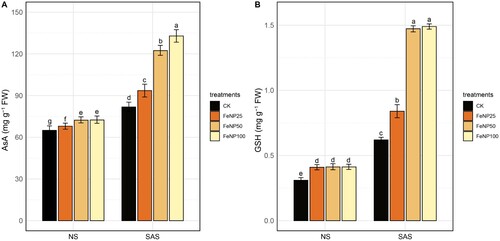
In the case of NS conditions, we also observed a slight increase in GSH contents by FeONP treatments, however, no significant differences were noted among treatment levels (B). Under SAS conditions, the FeONP25 treatment has increased GSH by 35.48% compared to control. The GSH levels under SAS conditions were further elevated at higher concentrations of FeONP50 and FeONP100 (an average increase in GSH levels by 140.02%) compared to control (B). However, a similar effect on GSH levels was found between these two treatments. Overall, these results suggest a positive impact of FeONP treatments in maintaining the redox status by modulating the AsA-GSH pool under SAS.
FeONP treatments improve proline, soluble sugar, and soluble protein levels under SAS
To investigate the impact of FeONP treatments on key osmolytes and their role in scavenging ROS, we assessed the levels of proline, soluble sugars, and soluble proteins in cherry tomato plants under both NS and SAS conditions ().
Figure 7. Quantification of changes in osmolytes in tomato leaves in control and FeONP-treated plants under NS and SAS. (A) Proline, (B) soluble sugars, and (C) soluble proteins. The data represent the mean values obtained from three independent experiments with error bars indicating standard errors. Bars marked with distinct letters denote significant differences, as determined by the Duncan test (p < 0.05).
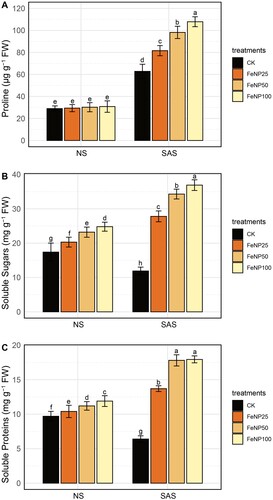
Interestingly, under NS conditions, no significant effect on proline content was observed with FeONP treatments at all concentration levels compared to the untreated control (A). Under SAS conditions, the proline level remarkably increased by 29.93%, 56.31%, and 71.64% in response to FeONP25, FeONP50, and FeONP100, respectively, compared to the untreated control under similar conditions (A).
Under NS conditions, the contents of soluble sugars and soluble proteins were slightly increased by FeONP treatments in a dose-dependent manner (A and B). However, under SAS conditions, the levels of soluble sugars and soluble proteins significantly declined in the untreated control, while they markedly increased with FeONP treatments. The contents of soluble sugars were distinctly increased by 133.61%, 188.23%, and 210.08% in response to FeONP25, FeONP50, and FeONP100, respectively, under SAS conditions compared to the untreated control (B). Similarly, the contents of soluble proteins were prominently increased by 114.06%, 178.12%, and 180.31% in response to FeONP25, FeONP50, and FeONP100, respectively, under SAS conditions compared to the untreated control (C). These results underscore the potential of FeONPs in ameliorating the impact of SAS on cellular osmotic balance and redox homeostasis.
FeONP improve bioactive compounds and antioxidant capacity under SAS
The contents of TPC and TFC from leaf extracts of cherry tomato plants are presented in . The content of TPC was slightly higher for FeONP25, FeONP50, and FeONP100 treatments compared to control under NS conditions (A). However, there was no significant difference in TFC levels after FeONP treatments under NS conditions (B). Under SAS conditions, there was a slight increase in TPC in untreated control plants, however, its content was significantly less than in FeONP treatments.
Figure 8. Determination of changes in total polyphenols and expression profile of flavonoids pathway genes in tomato leaves in control and FeONP-treated plants under NS and SAS. (A) Total phenolic content (TPC), (B) total flavonoid content (TFC), (C) genes involved in side branch of the flavonoids pathway, and (D) heatmap of expression profile for flavonoid pathway genes in tomato. The data represent the mean values obtained from three independent experiments with error bars indicating standard errors. Bars marked with distinct letters denote significant differences, as determined by the Duncan test (p < 0.05).
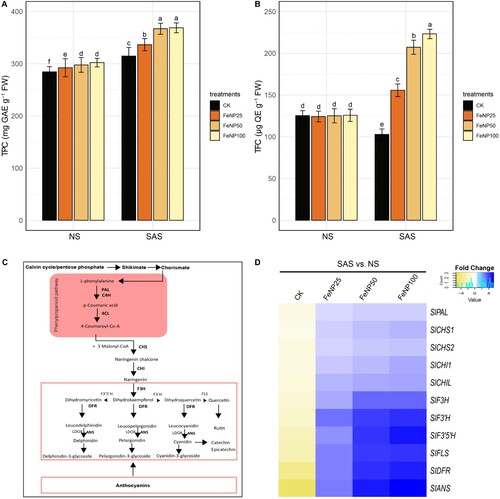
Under SAS conditions, the FeONP25 treatment has slightly increased TPC content by 6.92% compared to control. However, a similar effect on TPC levels was found between FeONP50 and FeONP100 treatments in response to SAS. The TPC levels under SAS conditions were further elevated by FeONP50 and FeONP100 (an average increase in TPC levels by 16.72%) compared to control (A).
In the case of TFC, we noticed a substantial decline in untreated control plants in response to SAS conditions (a decline of 18.26% in control plants in SAS vs. NS) (B). However, a prominent increase in TFC content in FeONP treatments was observed under similar conditions. The TFC content was increased in a dose-dependent manner by 51.40%, 101.45%, and 118.56% in response to FeONP25, FeONP50, and FeONP100, respectively, compared to the untreated control under SAS conditions (B).
Flavonoids are synthesized as secondary metabolites through the phenylpropanoid pathway. To validate the differential accumulation of flavonoid content, we examined the expression profiles of genes in the cherry tomato flavonoid pathway (C). Interestingly, there was a significant downregulation of gene expression in both early and late pathway genes in untreated control plants under SAS conditions (C and D). Conversely, transcripts for structural genes related to flavonoids were upregulated in response to FeONP treatments across all concentration levels (C and D). Notably, a distinct difference was observed in the expression profiles of late structural genes (SlF3H, SlF´3H, SlF3´5´H, SlFLS, SlDFR, and SlANS) between untreated control and FeONP treatments (C and D).
Further, the antioxidant capacity measured in terms of DPPH and FRAP in cherry tomato plants showed significant differences between control and FeONP treatments specifically under SAS conditions (). The DPPH scavenging activity substantially increased by 8.45%, 28.84%, and 31.86% in response to FeONP25, FeONP50, and FeONP100, respectively, under SAS conditions compared to the untreated control (A). Similarly, the FRAP antioxidant power was significantly increased by 26.40%, 34.41%, and 53.46% at FeONP25, FeONP50, and FeONP100, respectively, compared to the untreated control (B). These results strongly suggests that the antioxidant capacity measured by all testing systems substantially enhanced by FeONP treatments at different concentrations under SAS, albeit with varying levels.
Figure 9. Determination of total antioxidant activity in seedling extracts in control and FeONP-treated plants under NS and SAS. (A) Antioxidant activity of 2,2-diphenyl-1-picrylhydrazyl (DPPH), (B) antioxidant activity of ferric reducing antioxidant power (FRAP) assays. The data represent the mean values obtained from three independent experiments with error bars indicating standard errors. Bars marked with distinct letters denote significant differences, as determined by the Duncan test (p < 0.05).
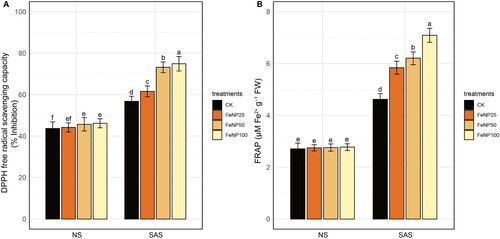
Multivariate analysis of oxidative stress, antioxidants, and osmoregulation under SAS
The Pearson’s correlation coefficient matrix and distribution analysis was used to examine the relationship among oxidative stress parameters, antioxidant enzymes, and other related biochemical markers based on the main outcomes of FeONP treatments under the SAS conditions. As shown in , which clearly indicates a strong negative correlation of enzymatic and non-enzymatic antioxidants (SOD, CAT, APX, AsA, GSH, TPC, TFC), antioxidant power (DPPH, FRAP), and other related osmoregulatory compounds (proline, SS, SP) with stress indicators (H2O2, O2, and MDA).
Figure 10. Pearson correlation analysis of oxidative stress, antioxidants, and osmoregulatory compounds under SAS. (A) Bubble plot for pairwise correlation among the parameters, (B) histogram of scatter matrix and coefficient values among the parameters. The p-values of all correlations were 0.05*, 0.01**, and 0.001***.
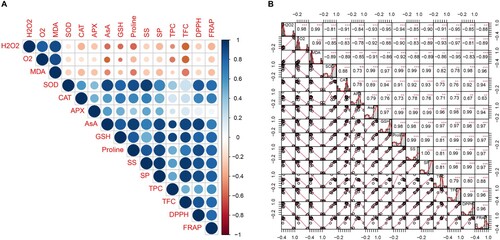
Among them, the H2O2 showed a strong negative correlation (p < 0.0001) with AsA (r = −0.93), GSH (r = −0.95), proline (r = −0.94), SP (r = −0.90), TPC (r = −0.91), and TFC (r = −0.95) (A and B). In the case of O2, a strong negative correlation (p < 0.0001) was observed with proline (r = −0.90), TPC (r = −0.93), and TFC (r = −0.97). Similarly, MDA showed a strong negative correlation (p < 0.0001) with AsA (r = −0.91), proline (r = −0.92), and TFC (r = −0.95) (A and B).
Interestingly, the non-antioxidants AsA and GSH showed a strong positive correlation (p < 0.0001) with both TPC (r = 0.96 and 0.97, respectively) and TFC (r = 0.98 and 0.97, respectively). However, the antioxidant enzymes showed a moderate positive correlation with these parameters (A and B). Further, both SS and SP also showed a strong positive correlation with TFC (r = 0.99 and 0.98, respectively). Additionally, the relationship between the antioxidant capacity measured by DPPH and FRAP exhibited a robust positive correlation with the majority of the parameters. However, in the case of APX, this correlation was found to be weak (as depicted in A and B).
Overall, these results demonstrate a pronounced inverse relationship between oxidative stress indicators and various antioxidants, osmoregulatory compounds, and antioxidant power in cherry tomatoes subjected to FeONP supplementation under SAS. Notably, strong negative correlations between stress indicators and key components highlight the potential ameliorative effects of FeONPs on oxidative stress in tomato plants.
Discussion
The cherry tomato holds a rich nutritional value and is widely cultivated around the world. So far, existing studies have primarily focused on advancing the production of high-quality tomatoes under salt stress (Fibiani et al. Citation2022), however, there is a notable scarcity of research addressing the complexities of salt-alkali mixed stress. For the past few years, numerous investigations have highlighted the significant contribution of nanotechnology approaches to enhance plant tolerance to various environmental stress factors in different plant species, as demonstrated by recent reports (Liu et al. Citation2021a; Singh et al. Citation2022; Song et al. Citation2023). Thus, the primary focus of the current research was to elucidate the impact of FeONPs on modulating SAS tolerance in cherry tomato plants. Notably, this study, like several others, focused on applying FeONPs at different concentrations in a controlled greenhouse environment, which allows for a better understanding of the fundamental interactions between the plants and the nanomaterials before testing them in the more variable and less predictable open-field conditions. Nanofertilizers and their bioformulations, used as nano-agrochemicals, are considered controlled-release nanomaterials. Their efficacy largely depends on environmental properties such as soil, microbes and their active enzymes in the soil, plant species, temperature, pH changes, light, and the redox conditions (Kalwani et al. Citation2022; Sári et al. Citation2023). Therefore, it is essential to consider these factors when evaluating NP-based biofertilizers in open-field conditions.
Fe is an essential micronutrient for various metabolic functions in plants, including chlorophyll synthesis and enzyme activities (Ning et al. Citation2023). In alkaline conditions, Fe tends to form insoluble complexes such as Fe-hydroxides, -phosphates, -sulfides, and -carbonates, rendering it less accessible to plants (Ning et al. Citation2023). Additionally, plants face reduced Fe uptake due to the formation of insoluble Fe compounds at the root surface (Hsieh and Waters Citation2016). These factors collectively impede the plants’ ability to acquire sufficient Fe, leading to deficiencies that can hinder their growth and overall development (Wang et al. Citation2022b). To overcome Fe deficiency, the use of Fe nano-fertilizers demonstrates a targeted and efficient approach to address Fe deficiencies in crops, ultimately contributing to improved agricultural productivity (Mahmoud et al. Citation2022).
Previous studies have clearly demonstrated a substantial reduction in plant biomass caused by alkaline salts, especially NaHCO3, and Na2CO3. These salts significantly lowered both the fresh and dry weights of the roots in sorghum plants (Zhang et al. Citation2018). Furthermore, a similar negative effect of alkaline salts on both shoot and root fresh and dry weights was observed in other plant species (Fatima et al. Citation2021; Ma et al. Citation2022). In agreement to these reports, our results also demonstrated a significant reduction in fresh and dry biomass of cherry tomato plants under SAS (). Interestingly, under both conditions of NS and SAS, the application of FeONPs enhanced plant biomass (shoot and root) and its effect was more pronounced at relatively higher concentrations (FeONP50, and FeONP100) used in this study (). Under stressful conditions, plants employ various mechanisms to enhance their biomass production, and one crucial strategy involves elevating pigment levels and optimizing photosynthetic performance (Lin et al. Citation2022). The study conducted by Manzoor et al. (Citation2021) demonstrated that FeONPs could effectively accumulate total chlorophyll contents and potentially enhanced the photosynthetic performance and biomass yield in wheat plants under salt and cadmium toxicity. In agreement to previous studies, our results clearly indicated a significant increase of chlorophyll a, b, total chlorophyll and carotenoid levels in response to FeONPs treatments under both NS and SAS conditions, as compared to untreated control (). This imply that plants supplied with FeONPs could efficiently perform photosynthetic functions and increase plant biomass under SAS compared to untreated control.
The elevated levels of sodium ions in the soil during saline-alkali stress can disturb the ion equilibrium within plant cells, resulting in detrimental effects such as the breakdown of cell membrane structure, disruption in metabolic activities, and ionic toxicity (Hasegawa Citation2013). The SAS induces a pronounced decline in the accumulation of both macro- and minor nutrient elements by disrupting their uptake and translocation. Hu et al. (Citation2022) found that the application of alkaline salts, particularly Na2CO3 and NaHCO3, caused a notable increase in sodium ions in Leymus chinensis seedlings, disrupting ion flux and reducing both essential macronutrients and micronutrients. As a result, seedling biomass development was significantly hindered by Na2CO3 and NaHCO3 salt-alkaline treatments (Hu et al. Citation2022). In the present study, our results also demonstrate that upon exposure to SAS, most of the macro- and minor nutrient elements were significantly reduced in the shoot and root tissues of cherry tomato plants ( and ). Intriguingly, the application of FeONPs could maintain the accumulation of macro- (N, K, P, Mg, Ca, and S) and minor elements (Zn, Fe, Mn, and Cu) at higher levels under SAS conditions compared to the control ( and ). A recent study demonstrated that FeONPs treatments increased H⁺-ATPase and H⁺-PPase activities in Trachyspermum ammi L. plants under salt stress, enhancing ion transport and maintaining ion homeostasis through vacuolar hydrolytic enzyme activation (Ghassemi-Golezani and Abdoli Citation2021). Additionally, the application of FeONPs, alone or combined with salicylic acid, significantly improved the K/Na ratio and increased levels of essential nutrient elements (Ca, Mg, Fe, Mn, Zn, N, and P) in plant tissues under stress conditions (Ghassemi-Golezani and Abdoli Citation2021). Another interesting study reported in rice revealed that the application of FeONPs during arsenic stress improved the expression level of Fe transporter genes (IRT1, and IRT2) in the roots, consequently, plants accumulate higher Fe and low arsenic contents in roots due to competitive uptake at root surface (Bidi et al. Citation2021). In the same study, higher Fe accumulation in the aerial parts of the plants was attributed to increased expression of long-distance transporter genes, namely FRDL1, YSL2, and YSL13 in rice (Bidi et al. Citation2021). In the present study, we found significantly higher Fe accumulation in the shoot and root of cherry tomatoes under both NS and SAS conditions due to FeONP treatments in a dose-dependent manner (). The Fe deficiency particularly in the leaves is a single limiting factor for reduction in chlorophyll biosynthesis due to chloroplast degeneration in plants (Li et al. Citation2021a). Therefore, in this study the increased Fe accumulation in leaves by the FeONPs treatments under SAS is in line with the higher accumulation of chlorophylls in cherry tomato, potentially contributing to plant growth restoration under stressful conditions. It is also worth mentioning that some researchers have demonstrated a significant effect of NPs on plant-microbe interactions, particularly regarding nutrient transport and availability. For instance, bacteria related to the mineralization of organic matter, such as those from the orders Sphingomonadales and Sphingomonadaceae, were enriched in the rhizosphere by the application of ZnONPs (Liu et al. Citation2021b). Therefore, it is crucial to explore the effect of FeONPs on soil microbes and plant-microbe interactions under given stress conditions to understand their potential impact on nutrient cycling and plant health. Moreover, the effect of FeONPs on various cross-membrane transport channels has not been analyzed in this study, which should be considered in future research to confirm the underlying mechanisms of ion regulation in cherry tomato plants under the given stress conditions.
Plants absorb light energy, allocating a fraction to photosynthesis, another to chlorophyll fluorescence, and yet another portion for heat dissipation (Endo et al. Citation2014). Environmental stress induces harm to plants by altering the activity of the photosynthetic electron transport chain, diminishing the effectiveness of photochemical reactions. Consequently, these processes indirectly generate surplus light energy, exacerbating light inhibition in a complex manner. However, chlorophyll fluorescence serves to alleviate this impact by absorbing and dissipating a portion of the excess light (Bassi and Dall'Osto Citation2021). In this study, the Pn, Gs, and Fv/Fm were markedly enhanced both under NS and SAS conditions by the application of FeONPs at all concentration levels used in this study (). However, a significant decline was observed for all these parameters in untreated control plants under the SAS conditions. The NPQ exhibited an opposite trend under SAS conditions, with higher values observed in the untreated control, while decreasing in a dose-dependent manner upon the application of FeONPs (D). A recent study conducted in Chinese cabbage (Brassica rapa L. ssp. pekinensis) also reported a significant decline in Pn, Gs, and Fv/Fm, while a sharp increase in NPQ values under salt and alkali stress conditions (Li et al. Citation2021c). On the other hand, FeONP treatments could reverse these mechanisms to maximize photosynthetic gain while maintaining low levels of NPQ under SAS conditions. One possible explanation could be that FeONPs might modulate the expression or activity of proteins involved in the photosynthetic machinery and the regulation of NPQ (Tombuloglu et al. Citation2020; Shah et al. Citation2022). This modulation could result in a more balanced energy utilization, with a greater proportion directed toward productive processes like photosynthesis rather than dissipative processes like NPQ. In this context, another recent study indicates that the application of Fe3O4 NPs could effectively enhance the rates of photosynthesis and PSII activity in wheat seedlings, thereby alleviating the negative effects of salt stress (Kreslavski et al. Citation2023).
The SAS disrupts the equilibrium between photosynthetic electron transport and the Calvin cycle. This disturbance results in a diminution of electron carriers within chloroplasts and mitochondria, culminating in the redirection of electrons from cytochrome to oxygen molecules and the concomitant generation of an elevated quantity of ROS, as elucidated by Foyer and Hanke (Citation2022). The excessive production of ROS induces oxidative stress, giving rise to detrimental effects on the cytoplasmic membrane and ultimately resulting in cellular damage and cell death (Guo et al. Citation2023). In the present study, the degree of oxidative stress was quantified by measuring the levels of H2O2, O2, and MDA in response to SAS. Based on our results, we observed a significant trigger in H2O2, O2, and MDA contents in untreated control plants under SAS conditions. Although, their levels were also increased in FeONP treatments under SAS conditions, but their accumulation was significantly lower at all concentrations compared to untreated control under the same conditions ().
We speculate that FeONPs treatments maintain ROS homeostasis in cherry tomato under SAS, and mitigate the damaging effects through the complex regulation of enzymatic and non-enzymatic antioxidant components of the defence system. To verify this hypothesis, we measured the activities of antioxidant enzymes (SOD, CAT, and APX) and expression of associated gene isoforms (A and B). As a stress signal, the activities of these protecting enzymes were triggered in response to SAS both in control and treated plants. However, their activities were significantly higher in FeONPs treatments (the activity of enzymes were increased in proportionate with the concentration of FeONPs) than in untreated control under the same conditions (A). SOD, a pivotal enzyme, regulates the levels of various ROS and reactive nitrogen species (RNS) in different cellular compartments, thereby restricting the potential toxicity of these molecules (Wang et al. Citation2018). Similarly, CAT and APX enzymes also play key roles in antioxidant system, Notably, APX exhibits a higher affinity for H2O2 than CAT, effectively converting it to water (H2O) in various cellular compartments such as chloroplasts, cytosol, mitochondria, and peroxisomes (Sofo et al. Citation2015). Further, APX is a key enzyme in the AsA-GSH cycle and detoxify H2O2 by using AsA as a substrate (Yin et al. Citation2019). Additionally, APX functions in the apoplastic space, utilizing ascorbate as a specific electron donor in this enzymatic process (Li Citation2023). In agreement with the results obtained in this study, previous reports also demonstrate the activation of these protective enzymes by FeONPs, albeit with different activity levels. For example, the effect of foliar application of FeONPs under salinity stress was studied on Dracocephalum moldavica L., a medicinal plant from the Lamiaceae family. The study revealed a higher activity of four antioxidant enzymes (CAT, GPX, GR, and APX) in the shoot and root of plants induced by FeONPs, thereby contributing to neutralizing the negative effects of stress (Moradbeygi et al. Citation2020). In another study, the simultaneous application of FeONPs and selenium to arsenic-stressed muskmelon (Cucumis melo) plants synergistically enhanced the activation of antioxidant enzymes, including SOD, POD, CAT, APX, GR, and DHAR (Shah et al. Citation2022). This combined treatment also resulted in improved photosynthesis, ultimately leading to enhanced plant growth under arsenic stress (Shah et al. Citation2022). In the light of previous reports, our study confirms that FeONP treatments effectively reduce ROS levels during SAS, promoting enhanced photosynthetic performance and plant growth by activating antioxidant enzymes.
In the non-enzymatic antioxidant defense system, AsA and GSH are two major antioxidants that stabilize cell membrane proteins by serving as buffers during redox reactions. A previous report indicates that the accumulation of AsA and GSH, along with genes related to osmoregulation in Trollius chinensis, an important medicinal plant, are crucial mechanisms for protecting plants under SAS conditions (Hou et al. Citation2023). In this study, the levels of these two important antioxidants were quantified in cherry tomato plants under NS and SAS conditions. Interestingly, the contents of AsA and GSH were increased in untreated control plants, however, FeONP treatments could enhance their contents to much higher levels than in control plants under stress conditions. The AsA-GSH cycle, a vital antioxidant-mediated defence mechanism, plays a crucial role in protecting enzymes from oxidation while scavenging surplus reactive oxygen species during stress conditions in plants. Apart from maintaining ROS below the toxic levels, they also interact with other components of the defence system and protect plants from serious damages (Hasanuzzaman et al. Citation2019). In this study, we observed a slight increase in AsA and GSH levels under SAS compared to their respective controls (). The application of FeONPs during SAS further enhanced these levels compared to the untreated control (). This enhancement could be attributed to the reduction of DHA and GSSG to AsA and GSH, respectively, thereby sustaining their high levels under SAS conditions. In tomato, the distinct expression of GST (glutathione S-transferases) gene classes – tau, lambda, theta, dehydroascorbate reductase, and zeta – facilitates this recycling process during salt acclimation (Csiszár et al. Citation2014). Similarly, in lima beans (Phaseolus lunatus), FeONPs alone or combined with silicon reduced cadmium toxicity by increasing GR and MDHAR activity, thus improving AsA and GSH levels and enhancing cadmium tolerance (Ahmad et al. Citation2021). Another recent study demonstrated that FeONPs significantly reduced cadmium toxicity in wheat plants by markedly decreasing oxidative stress markers and strengthening the components involved in the AsA-GSH cycle, namely glutathione, ascorbate, glutathione disulfide, and dehydroascorbic acid (Chen et al. Citation2024). GSH is also important for the biosynthesis of chlorophyll pigments in the chloroplasts and plays an integrated role in metabolic regulation and the detoxification of ROS (Müller-Schüssele et al. Citation2020). In this context, the regulation of cellular redox by the enhancement of AsA and GSH contents in this study explains the increase in total chlorophyll content by FeONPs. Likewise, in tomato, priming seeds with SeNPs was shown to enhance total chlorophyll and total antioxidant capacity, indicating a possible association with the AsA-GSH cycle (García-Locascio et al. Citation2024).
Under SAS conditions, plants trigger antioxidant defense and rely on osmoregulation for effective water absorption, maintaining cell turgor, cell shape, and regulating metabolic activities within plant cells. Research indicates that osmotic stress commonly manifests during the initial phases of saline–alkali stress (Wang et al. Citation2023a). To maintain the intracellular osmotic balance, plants usually accumulate osmoregulatory substances such as proline, soluble sugars, and soluble proteins for regulating osmotic balance and improving the salt or alkali tolerance of plants (Wang et al. Citation2021). In the present study, although proline was found to be increased in control plants during SAS conditions, however, its accumulation was much higher in FeONP-treated plants under similar conditions (A). The levels of soluble sugars and soluble proteins were sharply declined under SAS conditions. In contrast, their contents were significantly increased in response to FeONP treatments compared to the untreated control under SAS conditions (). Functioning as an osmotic regulator, sugar assumes a crucial role in upholding osmotic equilibrium during saline–alkali stress. Sucrose, glucose, and fructose are the main soluble sugar components which act as signaling molecules in plant metabolic processes and defense responses during salt–alkaline stress (Jia et al. Citation2019). Moreover, sucrose, as the primary product of photosynthesis, plays a crucial role in providing both a carbon skeleton and an energy source for the diverse metabolic activities in plants (Göbel and Fichtner Citation2023). The proline biosynthesis through either the glutamate or ornithine pathway plays a pivotal role in plant metabolism, particularly in orchestrating responses to a spectrum of abiotic stresses (Zulfiqar and Ashraf Citation2023). Both proline and the AsA-GSH cycle are interconnected and play significant roles in scavenging ROS. For instance, proline significantly reduces H2O2 and MDA levels and directly induces the accumulation of ASA, GSH, and GSH/GSSG contents, leading to the efficient maintenance of the redox balance within the cell (Gao et al. Citation2023). Additionally, the biosynthesis of proline can act as a signaling molecule, regulating the expression of several downstream genes in plants under stress conditions (Du et al. Citation2023). A previous study demonstrates that both ZnONPs and FeONPs could significantly increase the total protein and sugar content in rice at a very low concentration of 10 mg L−1 (Afzal et al. Citation2022), possibly through cell elongation and expansion, and by enhancing the translocation activity from roots to aerial parts of the plants. Another interesting study reveals that green-synthesized FeONPs could enhance proline levels by up to 115.4% and soluble sugars by up to 55% compared to their respective non-treated control plants, thereby inducing drought resistance in wheat plants (Noor et al. Citation2022). Another recent study demonstrates that FeONPs alone could enhance proline content in arsenic-stressed muskmelon plants and further intensified this effect when supplemented with selenium. Moreover, the authors revealed that this enhanced stress tolerance was achieved by activating multiple protective enzymes, which effectively reduce arsenic toxicity in plants (Shah et al. Citation2022). The regulation of the antioxidant system is a complex process involving the activation of several pathways, which corroborate to minimize oxidative damage. In light of all this evidence, our results confirm that FeONP treatments could significantly enhance osmoregulatory compounds and minimize ROS levels, enabling plants to carry out normal metabolic activities under SAS conditions.
In response to environmental challenges, plants activate numerous biochemical mechanisms to shield themselves from stress-induced harm. Among these mechanisms, phenolic compounds emerge as pivotal players, being both the predominant secondary metabolites in plants and essential antioxidants (Isah Citation2019). Their primary function lies in mitigating the detrimental effects of excessive ROS generated by diverse stressors. Additionally, flavonoids, belonging to the phenolic compound group, also contribute to this antioxidant defense (Agati et al. Citation2020). In the present study, we observed an increase in the accumulation of TPC with the application of FeONPs under both NS and SAS conditions. Interestingly, under non-stress conditions, the TFC remained unchanged at all concentrations of FeONPs applied. However, its levels were significantly enhanced with the application of FeONP treatments under SAS conditions, while TFC levels declined in the untreated control under stress conditions (). We further investigated whether FeONPs could alter the expression of key genes involved in the flavonoid biosynthetic pathway. Consistently, we observed upregulation of pathway genes in response to FeONP treatments, while downregulation was recorded in the untreated control under SAS (). In plants, flavonoids serve as crucial signal molecules within cells, engaging with transcription factors, kinases, and histones to intricately modulate gene expression across various regulatory tiers within the cellular machinery in response to SAS (An et al. Citation2016). In this study, although both upstream and downstream pathway genes were differentially regulated, however, the terminal pathway genes were strongly upregulated, particularly in response to FeONP50 and FeONP100, while strongly downregulated in untreated control (C and D). The MYB and bHLH transcription factor families play essential roles in plant flavonoid metabolism, closely linked to the expression levels of structural genes and influencing flavonoid biosynthesis through regulatory mechanisms (Ma et al. Citation2018). To effectively regulate the defense mechanism, it's crucial to fine-tune stress signals via transcription factors, necessitating the differential expression of genes in both the upstream and downstream pathways of flavonoids. A transcriptomics study conducted in a legume crop, Onobrychis viciifolia L., also unveiled the involvement of MYBs in regulating the differential expression of genes in the flavonoid pathway, particularly in the regulation of Proanthocyanidins (PAs), a substantial group of flavonoid compounds (Jin et al. Citation2022). In a similar study, it was revealed that the PAs accumulated in large quantities in both shoot and root tissues, thereby downregulating initial pathway genes (such as CHS and CHI) and upregulating terminal pathway genes (DFR and ANR) through the action of MYB factors (Jin et al. Citation2022). These MYB factors might serve as key activators or suppressors for flavonoid biosynthesis. In cotton (Gossypium hirsutum) plants, the differential expression of several transcription factors from distinct families, namely GRAS, WRKY, bZIP, C2H2, MYB-like, TCP, and HSF, was essential for scavenging ROS and enhancing SAS tolerance (Fan et al. Citation2021). Plant roots are the initial sensors of SAS cues, which subsequently initiate signaling pathways involving phytohormones. A recent transcriptomics study demonstrate that Na2CO3 stress evoked the most significant hormone signaling response in diploid and a tetraploid rice variety by up- and downregulation of several transcription factors (Wang et al. Citation2023c). In this context, an interesting piece of evidence reveals that Fe-based NPs could regulate the levels of four important phytohormones, including ABA, GA, IAA, and ZR, to alleviate oxidative stress and improve rice growth under Fe-deficient stress conditions (Li et al. Citation2021b). However, there is a need to investigate how FeONPs regulate flavonoid biosynthesis at the transcriptional level by modulating phytohormone signal transduction and various transcription factors under SAS.
Furthermore, our results also demonstrate that the antioxidant scavenging activities, measured in terms of DPPH and FRAP, were higher in FeONP-treated extracts compared to the untreated control under SAS conditions (). In agreement with our results, other researchers have also reported that the application of FeNPs, even at very low concentrations (3 mg L−1), could significantly enhance TPC, flavonol and flavonoids in canola plants under drought stress conditions (Rezayian et al. Citation2023). Other researchers have reported that the application of Fe3O4 NPs could enhance TPC and TFC in Leonurus cardiaca, a medicinal plant from the Lamiaceae family, thereby contributing to an increase in shoot and root biomass (Jafari and Hatami Citation2022). The effect of Fe3O4 NPs alone or in combination with salicylic acid significantly enhances total phenols, flavonoids, flavonols, and anthocyanins in the leaves and hairy roots of Calotropis procera, a medicinally important plant, compared to the control (Adabavazeh et al. Citation2022). Similarly, the antioxidant capacity of DPPH and FRAP, both in seedlings and hairy roots, was markedly higher in Fe3O4 NP treatments compared to control plants, with a significant correlation observed between total phenols and antioxidant capacities in these tissues (Adabavazeh et al. Citation2022). This explains that the application of FeONP treatments was effective in scavenging ROS by enhancing the antioxidant potential and ultimately elevating SAS tolerance in cherry tomatoes.
Conclusion
In conclusion, our study highlights the substantial potential of FeONPs in mitigating SAS in cherry tomatoes. Plants respond to the application of FeONPs in a dose-dependent manner, demonstrating remarkable improvements in biomass, availability of nutrient elements, pigment contents, and photosynthetic efficiency under stress conditions. This highlights their efficacy in enhancing plant resilience. Among different treatments, the application of FeONP100 showed the best performance in terms of growth, physiological, and biochemical parameters, thus significantly improving cherry tomatoes. The notable enhancements observed in enzymatic and non-enzymatic antioxidants, along with osmoregulatory substances, indicate a promising avenue for enhancing antioxidant potential in cherry tomatoes under stress. Moving forward, it is crucial to investigate the underlying mechanisms of FeONP-mediated stress alleviation through omics studies. Additionally, optimizing application strategies for maximum efficacy and economic benefit under field conditions is necessary. Long-term assessments of FeONP impacts on soil health and ecosystem dynamics are also essential. The continued exploration of FeONPs in agriculture holds immense potential for sustainable crop production and stress tolerance in the face of changing environmental conditions.
Author contributions
Conceptualization and design, Raheel Shahzad and Putri W. Harlina; Data curation and interpretation, Raheel Shahzad, Putri W. Harlina, Shahid Ullah Khan, Ivica Djalovic, Sri Koerniati, Rizwan Wahab, Bernadetta R. Hastilestari, Ratih A. Ningrum, PV Vara Prasad; Resources, Ivica Djalovic and PV Vara Prasad; Software, Raheel Shahzad; Validation, Raheel Shahzad and Putri W. Harlina; Visualization, Raheel Shahzad and Putri W. Harlina; Writing – original draft, Raheel Shahzad; Writing – review & editing, Raheel Shahzad, Putri W. Harlina, Shahid Ullah Khan, Ivica Djalovic, Sri Koerniati, Rizwan Wahab, Bernadetta R. Hastilestari, Ratih A. Ningrum, and PV Vara Prasad. All authors read and approved the final manuscript.
Supplemental Material
Download MS Excel (9.8 KB)Acknowledgements
The authors express deep gratitude for the research facilities provided by the National Research and Innovation Agency (BRIN), Indonesia; Southwest University, China; and King Saud University, Saudi Arabia. The author (Ivica Djalovic) would like to thank the Ministry of Science, Technological Development and Innovation of the Republic of Serbia (grant number 451−03−66/2024−03/200032). The author (Rizwan Wahab) would also like to thank the Researchers Supporting Project Number (RSPD2024R1042), King Saud University, Riyadh, Saudi Arabia.
Disclosure statement
No potential conflict of interest was reported by the author(s).
Data availability statement
The original contributions presented in this study are included in the article, further inquiries can be directed to the corresponding author.
Additional information
Funding
Notes on contributors
Raheel Shahzad
Raheel Shahzad, postdoctoral research fellow at BRIN, Indonesia. His work focuses on using biotechnology and nanotechnology approaches to explore the physiological, cellular, and molecular mechanisms of plant adaptations to various environmental stress factors.
Putri Widyanti Harlina
Putri Widyanti Harlina, assistant professor at department of food technology, Universitas Padjadjaran, Indonesia. She focuses on lipidomics and antioxidation functions in foods.
Shahid Ullah Khan
Shahid Ullah Khan, assistant professor at college of agronomy and biotechnology, Southwest University, Chongqing, China.
Sri Koerniati
Sri Koerniati, researcher at BRIN, Indonesia. She focuses on genetic editing approaches to improve crop/horticultural plants.
Bernadetta Rina Hastilestari
Bernadetta Rina Hastilestari, researcher at BRIN, Indonesia. She focuses on genetic editing approaches to improve crop/horticultural plants.
Ratih Asmana Ningrum
Ratih Asmana Ningrum works as senior researcher at research center for genetic engineering BRIN, Indonesia.
Rizwan Wahab
Rizwan Wahab works at Zoology Department, College of Science, King Saud University, Riyadh 11451, Kingdom of Saudi Arabia.
Ivica Djalovic
Ivica Djalovic, professor, works at National Institute of the Republic of Serbia. He focuses on plant physiology and crop production.
P. V. Vara Prasad
PV Vara Prasad, full professor, working at Kansas State University, USA. His expertise is in plant physiology, ecophysiology, and sustainable agriculture.
References
- Adabavazeh F, Pourseyedi S, Nadernejad N, Razavizadeh R, Mozafari H. 2022. Evaluation of synthesized magnetic nanoparticles and salicylic acid effects on improvement of antioxidant properties and essential oils of Calotropis procera hairy roots and seedlings. Plant Cell Tiss Organ Cult. 151:133–148. doi:10.1007/s11240-022-02338-w.
- Afzal S, Aftab T, Singh NK. 2022. Impact of zinc oxide and iron oxide nanoparticles on uptake, translocation, and physiological effects in Oryza sativa L. J. Plant Growth Regul. 41:1445–1461. doi:10.1007/s00344-021-10388-1.
- Agati G, Brunetti C, Fini A, Gori A, Guidi L, Landi M, Sebastiani F, Tattini M. 2020. Are flavonoids effective antioxidants in plants? Twenty years of our investigation. Antioxidants. 9(11):1098. doi:10.3390/antiox9111098.
- Ahmad A, Yasin NA, Khan WU, Akram W, Wang R, Shah AA, Akbar M, Ali A, Wu T. 2021. Silicon assisted ameliorative effects of iron nanoparticles against cadmium stress: attaining new equilibrium among physiochemical parameters, antioxidative machinery, and osmoregulators of Phaseolus lunatus. Plant Physiol Biochem. 166:874–886. doi:10.1016/j.plaphy.2021.06.016.
- Ahmad F, Kusumiyati K, Soleh MA, Khan MR, Sundari RS. 2023. Watering volume and growing design’s effect on the productivity and quality of cherry tomato (solanum lycopersicum cerasiformae) cultivar ruby. Agronomy. 13(9):2417. doi:10.3390/agronomy13092417.
- Alabdallah NM, Hasan MM, Hammami I, Alghamdi AI, Alshehri D, Alatawi HA. 2021. Green synthesized metal oxide nanoparticles mediate growth regulation and physiology of crop plants under drought stress. Plants. 10(8):1730. doi:10.3390/plants10081730.
- Ali EF, El-Shehawi AM, Ibrahim OHM, Abdul-Hafeez EY, Moussa MM, Hassan FAS. 2021. A vital role of chitosan nanoparticles in improvisation the drought stress tolerance in Catharanthus roseus (L.) through biochemical and gene expression modulation. Plant Physiol Biochem. 161:166–175. doi:10.1016/j.plaphy.2021.02.008.
- Al-Khayri JM, Rashmi R, Ulhas R, Sudheer WN, Banadka A, Nagella P, Aldaej MI, Rezk AA, Shehata WF, Almaghasla MI. 2023. The role of nanoparticles in response of plants to abiotic stress at physiological, biochemical, and molecular levels. Plants. 12(2):292. doi:10.3390/plants12020292.
- Almendros P, González D, Fernández MD, García-Gomez C, Obrador A. 2022. Both Zn biofortification and nutrient distribution pattern in cherry tomato plants are influenced by the application of ZnO nanofertilizer. Heliyon. 8:e09130. doi:10.1016/j.heliyon.2022.e09130.
- An Y-M, Song L-L, Liu Y-R, Shu Y-J, Guo C-H. 2016. De novo transcriptional analysis of alfalfa in response to saline-alkaline stress. Front Plant Sci. 7:931. doi:10.3389/fpls.2016.00931.
- Bai L, Wang C, Zang S, Wu C, Luo J, Wu Y. 2018. Mapping soil alkalinity and salinity in northern songnen plain, China with the HJ-1 hyperspectral imager data and partial least squares regression. Sensors. 18(11):3855. doi:10.3390/s18113855.
- Bassi R, Dall'Osto L. 2021. Dissipation of light energy absorbed in excess: the molecular mechanisms. Annu Rev Plant Biol. 72:47–76. doi:10.1146/annurev-arplant-071720-015522.
- Bidi H, Fallah H, Niknejad Y, Barari Tari D. 2021. Iron oxide nanoparticles alleviate arsenic phytotoxicity in rice by improving iron uptake, oxidative stress tolerance and diminishing arsenic accumulation. Plant Physiol Biochem. 163:348–357. doi:10.1016/j.plaphy.2021.04.020.
- Chen F, Jiang F, Okla MK, Abbas ZK, Al-Qahtani SM, Al-Harbi NA, Abdel-Maksoud MA, Gómez-Oliván LM. 2024. Nanoparticles synergy: enhancing wheat (Triticum aestivum L.) cadmium tolerance with iron oxide and selenium. Sci. Total Environ. 915:169869. doi:10.1016/j.scitotenv.2024.169869.
- Choi HG. 2021. Effect of TiO2 nanoparticles on the yield and photophysiological responses of cherry tomatoes during the rainy season. Horticulturae. 7(12):563. doi:10.3390/horticulturae7120563.
- Csiszár J, Horváth E, Váry Z, Gallé A, Bela K, Brunner S, Tari I. 2014. Glutathione transferase supergene family in tomato: salt stress-regulated expression of representative genes from distinct GST classes in plants primed with salicylic acid. Plant Physiol Biochem. 78:15–26. doi:10.1016/j.plaphy.2014.02.010.
- Djanaguiraman M, Belliraj N, Bossmann S, Prasad PVV. 2018. High temperature stress alleviation by selenium nanoparticles treatment in grain sorghum. ACS Omega. 3(3):2479–2491. doi:10.1021/acsomega.7b01934.
- Djanaguiraman M, Nair R, Giraldo JP, Prasad PVV. 2018. Cerium oxide nanoparticles decrease drought-induced oxidative damage in sorghum leading to higher photosynthesis and grain yield. ACS Omega. 3(10):14406–14416. doi:10.1021/acsomega.8b01894.
- Du L, Huang X, Ding L, Wang Z, Tang D, Chen B, Ao L, Liu Y, Kang Z, Mao H. 2023. TaERF87 and TaAKS1 synergistically regulate TaP5CS1/TaP5CR1-mediated proline biosynthesis to enhance drought tolerance in wheat. New Phytol. 237:232–250. doi:10.1111/nph.18549.
- Du Y, Zhao Q, Chen L, Yao X, Zhang W, Zhang B, Xie F. 2020. Effect of drought stress on sugar metabolism in leaves and roots of soybean seedlings. Plant Physiol Biochem. 146:1–12. doi:10.1016/j.plaphy.2019.11.003.
- Dumanović J, Nepovimova E, Natić M, Kuča K, Jaćević V. 2021. The significance of reactive oxygen species and antioxidant defense system in plants: A concise overview. Front Plant Sci. 11:552969. doi:10.3389/fpls.2020.552969.
- Endo T, Uebayashi N, Ishida S, Ikeuchi M, Sato F. 2014. Light energy allocation at PSII under field light conditions: how much energy is lost in NPQ-associated dissipation? Plant Physiol Biochem. 81:115–120. doi:10.1016/j.plaphy.2014.03.018.
- Fan Y, Lu X, Chen X, Wang J, Wang D, Wang S, Guo L, Rui C, Zhang Y, Cui R, et al. 2021. Cotton transcriptome analysis reveals novel biological pathways that eliminate reactive oxygen species (ROS) under sodium bicarbonate (NaHCO3) alkaline stress. Genomics. 113:1157–1169. doi:10.1016/j.ygeno.2021.02.022.
- Fatima A, Hussain S, Hussain S, Ali B, Ashraf U, Zulfiqar U, Aslam Z, Al-Robai SA, Alzahrani FO, Hano C, et al. 2021. Differential morphophysiological, biochemical, and molecular responses of maize hybrids to salinity and alkalinity stresses. Agronomy. 11(6):1150. doi:10.3390/agronomy11061150.
- Fibiani M, Paolo D, Leteo F, Campanelli G, Picchi V, Bianchi G, Lo Scalzo R. 2022. Influence of year, genotype and cultivation system on nutritional values and bioactive compounds in tomato (Solanum lycopersicum L.). Food Chem. 389:133090. doi:10.1016/j.foodchem.2022.133090.
- Foyer CH, Hanke G. 2022. ROS production and signalling in chloroplasts: cornerstones and evolving concepts. Plant J. 111(3):642–661. doi:10.1111/tpj.15856.
- Gao F, Zhang X, Zhang J, Li J, Niu T, Tang C, Wang C, Xie J. 2022. Zinc oxide nanoparticles improve lettuce (Lactuca sativa L.) plant tolerance to cadmium by stimulating antioxidant defense, enhancing lignin content and reducing the metal accumulation and translocation. Front Plant Sci. 13:1015745. doi:10.3389/fpls.2022.1015745.
- Gao Y, Zhang J, Wang C, Han K, Hu L, Niu T, Yang Y, Chang Y, Xie J. 2023. Exogenous proline enhances systemic defense against salt stress in celery by regulating photosystem, phenolic compounds, and antioxidant system. Plants. 12(4):928. doi:10.3390/plants12040928.
- García-Locascio E, Valenzuela EI, Cervantes-Avilés P. 2024. Impact of seed priming with selenium nanoparticles on germination and seedlings growth of tomato. Sci Rep. 14:6726. doi:10.1038/s41598-024-57049-3.
- Ghassemi-Golezani K, Abdoli S. 2021. Improving ATPase and PPase activities, nutrient uptake and growth of salt stressed ajowan plants by salicylic acid and iron-oxide nanoparticles. Plant Cell Rep. 40:559–573. doi:10.1007/s00299-020-02652-7.
- Göbel M, Fichtner F. 2023. Functions of sucrose and trehalose 6-phosphate in controlling plant development. J Plant Physiol. 291:154140. doi:10.1016/j.jplph.2023.154140.
- Guo W, Xing Y, Luo X, Li F, Ren M, Liang Y. 2023. Reactive oxygen species: a crosslink between plant and human eukaryotic cell systems. Int J Mol Sci. 24(17):13052. doi:10.3390/ijms241713052.
- Gutiérrez-Miceli FA, Oliva-Llaven MÁ, Luján-Hidalgo MC, Velázquez-Gamboa MC, González-Mendoza D, Sánchez-Roque Y. 2021. Zinc oxide phytonanoparticles’ effects on yield and mineral contents in fruits of tomato (Solanum lycopersicum L. cv. Cherry) under field conditions. Scientific World J. 2021:5561930. doi:10.1155/2021/5561930.
- Hasanuzzaman M, Bhuyan M, Anee TI, Parvin K, Nahar K, Mahmud JA, Fujita M. 2019. Regulation of ascorbate-glutathione pathway in mitigating oxidative damage in plants under abiotic stress. Antioxidants. 8(9):384. doi:10.3390/antiox8090384.
- Hasanuzzaman M, Bhuyan MHMB, Parvin K, Bhuiyan TF, Anee TI, Nahar K, Hossen MS, Zulfiqar F, Alam MM, Fujita M. 2020. Regulation of ROS metabolism in plants under environmental stress: a review of recent experimental evidence. Int J Mol Sci. 21(22):8695. doi:10.3390/ijms21228695.
- Hasegawa PM. 2013. Sodium (Na+) homeostasis and salt tolerance of plants. Environ Exp Bot. 92:19–31. doi:10.1016/j.envexpbot.2013.03.001.
- He X, Wan Z, Jin N, Jin L, Zhang G, Lyu J, Liu Z, Luo S, Yu J. 2022. Enhancement of cucumber resistance under salt stress by 2, 4-epibrassinolide lactones. Front Plant Sci. 13:1023178. doi:10.3389/fpls.2022.1023178.
- Heath RL, Packer L. 1968. Photoperoxidation in isolated chloroplasts. I. Kinetics and stoichiometry of fatty acid peroxidation. Arch Biochem Biophys. 125(1):189–198. doi:10.1016/0003-9861(68)90654-1.
- Hou R, Yang L, Wuyun T, Chen S, Zhang L. 2023. Genes related to osmoregulation and antioxidation play important roles in the response of Trollius chinensis seedlings to saline-alkali stress. Front Plant Sci. 14:1080504. doi:10.3389/fpls.2023.1080504.
- Hsieh EJ, Waters BM. 2016. Alkaline stress and iron deficiency regulate iron uptake and riboflavin synthesis gene expression differently in root and leaf tissue: implications for iron deficiency chlorosis. J Exp Bot. 67(19):5671–5685. doi:10.1093/jxb/erw328.
- Hu X, Wang D, Ren S, Feng S, Zhang H, Zhang J, Qiao K, Zhou A. 2022. Inhibition of root growth by alkaline salts due to disturbed ion transport and accumulation in Leymus chinensis. Environ Exp Bot. 200:104907. doi:10.1016/j.envexpbot.2022.104907.
- Huihui Z, Xin L, Yan-hui C, Yue W, Ma-bo L, Rong-yi Y, Nan X, Guang-yu S. 2020. A study on the effects of salinity and pH on PSII function in mulberry seedling leaves under saline–alkali mixed stress. Trees. 34:693–706. doi:10.1007/s00468-019-01949-9.
- Hussain A, Ali S, Rizwan M, Rehman M, Qayyum MF, Wang H, Rinklebe J. 2019. Responses of wheat (Triticum aestivum) plants grown in a Cd contaminated soil to the application of iron oxide nanoparticles. Ecotoxicol Environ Saf. 173:156–164. doi:10.1016/j.ecoenv.2019.01.118.
- Isah T. 2019. Stress and defense responses in plant secondary metabolites production. Biol Res. 52:39. doi:10.1186/s40659-019-0246-3.
- Jafari A, Hatami M. 2022. Foliar-applied nanoscale zero-valent iron (nZVI) and iron oxide (Fe3O4) induce differential responses in growth, physiology, antioxidative defense and biochemical indices in Leonurus cardiaca L. Environ Res. 215(2):114254. doi:10.1016/j.envres.2022.114254.
- Jia XM, Zhu YF, Hu Y, Zhang R, Cheng L, Zhu ZI, Zhao T, Zhang X, Wang YX. 2019. Integrated physiologic, proteomic, and metabolomic analyses of Malus halliana adaptation to saline–alkali stress. Hortic Res. 6:91. doi:10.1038/s41438-019-0172-0.
- Jin X, Liu T, Xu J, Gao Z, Hu X. 2019. Exogenous GABA enhances muskmelon tolerance to salinity-alkalinity stress by regulating redox balance and chlorophyll biosynthesis. BMC Plant Biol. 19(1):48. doi:10.1186/s12870-019-1660-y.
- Jin Z, Jiang W, Luo Y, Huang H, Yi D, Pang Y. 2022. Analyses on flavonoids and transcriptome reveals Key MYB gene for proanthocyanidins regulation in Onobrychis Viciifolia. Front Plant Sci. 13:941918. doi:10.3389/fpls.2022.941918.
- Kalwani M, Chakdar H, Srivastava A, Pabbi S, Shukla P. 2022. Effects of nanofertilizers on soil and plant-associated microbial communities: emerging trends and perspectives. Chemosphere. 287:132107. doi:10.1016/j.chemosphere.2021.132107.
- Kreslavski VD, Shmarev AN, Ivanov AA, Zharmukhamedov SK, Strokina V, Kosobryukhov A, Yu M, Allakhverdiev SI, Shabala S. 2023. Effects of iron oxide nanoparticles (Fe3O4) and salinity on growth, photosynthesis, antioxidant activity and distribution of mineral elements in wheat (Triticum aestivum). Funct Plant Biol. 50(11):932–940. doi:10.1071/FP23085.
- Li J, Cao X, Jia X, Liu L, Cao H, Qin W, Li M. 2021a. Iron deficiency leads to chlorosis through impacting chlorophyll synthesis and nitrogen metabolism in Areca catechu L. Front Plant Sci. 12:710093. doi:10.3389/fpls.2021.710093.
- Li J, Yang Y. 2023. How do plants maintain pH and ion homeostasis under saline-alkali stress? Front Plant Sci. 14:1217193. doi:10.3389/fpls.2023.1217193.
- Li M, Zhang P, Adeel M, Guo Z, Chetwynd AJ, Ma C, Bai T, Hao Y, Rui Y. 2021b. Physiological impacts of zero valent iron, Fe3O4 and Fe2O3 nanoparticles in rice plants and their potential as Fe fertilizers. Environ Pollut. 269:116134. doi:10.1016/j.envpol.2020.116134.
- Li N, Zhang Z, Gao S, Lv Y, Chen Z, Cao B, Xu K. 2021c. Different responses of two Chinese cabbage (Brassica rapa L. ssp. pekinensis) cultivars in photosynthetic characteristics and chloroplast ultrastructure to salt and alkali stress. Planta. 254(5):102. doi:10.1007/s00425-021-03754-6.
- Li S. 2023. Novel insight into functions of ascorbate peroxidase in higher plants: more than a simple antioxidant enzyme. Redox Biol. 64:102789. doi:10.1016/j.redox.2023.102789.
- Lin S, Song X-F, Mao H-T, Li S-Q, Gan J-Y, Yuan M, Zhang Z-W, Yuan S, Zhang H-Y, Su Y-Q, et al. 2022. Exogenous melatonin improved photosynthetic efficiency of photosystem II by reversible phosphorylation of thylakoid proteins in wheat under osmotic stress. Front Plant Sci. 13:966181. doi:10.3389/fpls.2022.966181.
- Liu J, Li G, Chen L, Gu J, Wu H, Li Z. 2021a. Cerium oxide nanoparticles improve cotton salt tolerance by enabling better ability to maintain cytosolic K+/Na+ ratio. J Nanobiotechnology. 19(1):153. doi:10.1186/s12951-021-00892-7.
- Liu Y, Li Y, Pan B, Zhang XY, Zhang H, Steinberg CEW, Qiu H, Vijver MG, Peijnenburg W. 2021b. Application of low dosage of copper oxide and zinc oxide nanoparticles boosts bacterial and fungal communities in soil. Sci Total Environ. 757:143807. doi:10.1016/j.scitotenv.2020.143807.
- Lu X, Wu Q, Nie K, Wu H, Chen G, Wang J, Ma Z. 2023. Exogenous phthalanilic acid induces resistance to drought stress in pepper seedlings (Capsicum annuum L.). Front Plant Sci. 14:1156276. doi:10.3389/fpls.2023.1156276.
- Ma C, Yuan S, Xie B, Li Q, Wang Q, Shao M. 2022. IAA plays an important role in alkaline stress tolerance by modulating root development and ROS detoxifying systems in rice plants. Int J Mol Sci. 23(23):14817. doi:10.3390/ijms232314817.
- Ma D, Reichelt M, Yoshida K, Gershenzon J, Constabel CP. 2018. Two R2R3-MYB proteins are broad repressors of flavonoid and phenylpropanoid metabolism in poplar. Plant J. 96(5):949–965. doi:10.1111/tpj.14081.
- Machado J, Vasconcelos MW, Soares C, Fidalgo F, Heuvelink E, Carvalho SMP. 2023. Enzymatic and Non-enzymatic antioxidant responses of young tomato plants (cv. Micro-Tom) to single and combined mild nitrogen and water deficit: not the sum of the parts. Antioxidants. 12(2):375. doi:10.3390/antiox12020375.
- Mahmoud AWM, Ayad AA, Abdel-Aziz HSM, Williams LL, El-Shazoly RM, Abdel-Wahab A, Abdeldaym EA. 2022. Foliar application of different iron sources improves morpho-physiological traits and nutritional quality of broad bean grown in sandy soil. Plants. 11(19):2599. doi:10.3390/plants11192599.
- Manzoor N, Ahmed T, Noman M, Shahid M, Nazir MM, Ali L, Alnusaire TS, Li B, Schulin R, Wang G. 2021. Iron oxide nanoparticles ameliorated the cadmium and salinity stresses in wheat plants, facilitating photosynthetic pigments and restricting cadmium uptake. Sci Total Environ. 769:145221. doi:10.1016/j.scitotenv.2021.145221.
- Manzoor N, Ali L, Al-Huqail AA, Alghanem SMS, Al-Haithloul HAS, Abbas T, Chen G, Huan L, Liu Y, Wang G. 2023. Comparative efficacy of silicon and iron oxide nanoparticles towards improving the plant growth and mitigating arsenic toxicity in wheat (Triticum aestivum L.). Ecotoxicol Environ Saf. 264:115382. doi:10.1016/j.ecoenv.2023.115382.
- Moradbeygi H, Jamei R, Heidari R, Darvishzadeh R. 2020. Investigating the enzymatic and non-enzymatic antioxidant defense by applying iron oxide nanoparticles in Dracocephalum moldavica L. plant under salinity stress. Sci Hortic. 272:109537. doi:10.1016/j.scienta.2020.109537.
- Müller-Schüssele SJ, Wang R, Gütle DD, Romer J, Rodriguez-Franco M, Scholz M, Buchert F, Lüth VM, Kopriva S, Dörmann P, et al. 2020. Chloroplasts require glutathione reductase to balance reactive oxygen species and maintain efficient photosynthesis. Plant J. 103:1140–1154. doi:10.1111/tpj.14791.
- Mustafa H, Ilyas N, Akhtar N, Raja NI, Zainab T, Shah T, Ahmad A, Ahmad P. 2021. Biosynthesis and characterization of titanium dioxide nanoparticles and its effects along with calcium phosphate on physicochemical attributes of wheat under drought stress. Ecotoxicol Environ Saf. 223:112519. doi:10.1016/j.ecoenv.2021.112519.
- Neysanian M, Ahmadvand R, Oraghi Ardebili Z, Ebadi M. 2021. The effect of selenium nanoparticles on some morphological and anatomical features of cherry tomato plants (Solanum lycopersicum L. var. cerasiforme). Dev Biol. 13:39–54. doi:10.30495/jdb.2021.686361.
- Nie W, Gong B, Chen Y, Wang J, Wei M, Shi Q. 2018. Photosynthetic capacity, ion homeostasis and reactive oxygen metabolism were involved in exogenous salicylic acid increasing cucumber seedlings tolerance to alkaline stress. Sci Hortic. 235:413–423. doi:10.1016/j.scienta.2018.03.011.
- Ning X, Lin M, Huang G, Mao J, Gao Z, Wang X. 2023. Research progress on iron absorption, transport, and molecular regulation strategy in plants. Front Plant Sci. 14:1190768. doi:10.3389/fpls.2023.1190768.
- Noor R, Yasmin H, Ilyas N, Nosheen A, Hassan MN, Mumtaz S, Khan N, Ahmad A, Ahmad P. 2022. Comparative analysis of iron oxide nanoparticles synthesized from ginger (Zingiber officinale) and cumin seeds (Cuminum cyminum) to induce resistance in wheat against drought stress. Chemosphere. 292:133201. doi:10.1016/j.chemosphere.2021.133201.
- Rao Y, Peng T, Xue S. 2023. Mechanisms of plant saline-alkaline tolerance. J Plant Physiol. 281:153916. doi:10.1016/j.jplph.2023.153916.
- Rezayian M, Niknam V, Arabloo M. 2023. Iron nanoparticle regulate succinate dehydrogenase activity in canola plants under drought stress. Sci Rep. 13(1):9628. doi:10.1038/s41598-023-36105-4.
- Rui M, Ma C, Hao Y, Guo J, Rui Y, Tang X, Zhao Q, Fan X, Zhang Z, Hou T, et al. 2016. Iron oxide nanoparticles as a potential iron fertilizer for peanut (Arachis hypogaea). Front Plant Sci. 7:815. doi:10.3389/fpls.2016.00815.
- Sagervanshi A, Naeem A, Kaiser H, Pitann B, Mühling KH. 2021. Early growth reduction in Vicia faba L. under alkali salt stress is mainly caused by excess bicarbonate and related to citrate and malate over accumulation. Environ Exp Bot. 192:104636. doi:10.1016/j.envexpbot.2021.104636.
- Sahab S, Suhani I, Srivastava V, Chauhan PS, Singh RP, Prasad V. 2021. Potential risk assessment of soil salinity to agroecosystem sustainability: current status and management strategies. Sci Total Environ. 764:144164. doi:10.1016/j.scitotenv.2020.144164.
- Sári D, Ferroudj A, Abdalla N, El-Ramady H, Dobránszki J, Prokisch J. 2023. Nano-management approaches for salt tolerance in plants under field and in vitro conditions. Agronomy. 13:2695. doi:10.3390/agronomy13112695.
- Sarker U, Oba S. 2018. Drought stress enhances nutritional and bioactive compounds, phenolic acids and antioxidant capacity of Amaranthus leafy vegetable. BMC Plant Biol. 18(1):258. doi:10.1186/s12870-018-1484-1.
- Schmittgen TD, Livak KJ. 2008. Analyzing real-time PCR data by the comparative C(T) method. Nat Protoc. 3(6):1101–1108. doi:10.1038/nprot.2008.73.
- Shah AA, Yasin NA, Mudassir M, Ramzan M, Hussain I, Siddiqui MH, Ali HM, Shabbir Z, Ali A, Ahmed S, et al. 2022. Iron oxide nanoparticles and selenium supplementation improve growth and photosynthesis by modulating antioxidant system and gene expression of chlorophyll synthase (CHLG) and protochlorophyllide oxidoreductase (POR) in arsenic-stressed Cucumis melo. Environ Pollut. 307:119413. doi:10.1016/j.envpol.2022.119413.
- Shahzad R, Harlina PW, Gallego PP, Flexas J, Ewas M, Leiwen X, Karuniawan A. 2023. The seaweed Ascophyllum nodosum-based biostimulant enhances salt stress tolerance in rice (Oryza sativa L.) by remodeling physiological, biochemical, and metabolic responses. J Plant Interact. 18:2266514. doi:10.1080/17429145.2023.2266514.
- Singh A, Sengar RS, Rajput VD, Minkina T, Singh RK. 2022. Zinc oxide nanoparticles improve salt tolerance in rice seedlings by improving physiological and biochemical indices. Agriculture. 12(7):1014. doi:10.3390/agriculture12071014.
- Sofo A, Scopa A, Nuzzaci M, Vitti A. 2015. Ascorbate peroxidase and catalase activities and their genetic regulation in plants subjected to drought and salinity stresses. Int J Mol Sci. 16:13561–13578. doi:10.3390/ijms160613561.
- Song Y, Zheng C, Li S, Chen J, Jiang M. 2023. Chitosan-Magnesium oxide nanoparticles improve salinity tolerance in rice (Oryza sativa L.). ACS Appl Mater Interfaces. 15(17):20649–20660. doi:10.1021/acsami.3c00043.
- Tawfik MM, Mohamed MH, Sadak MS, Thalooth AT. 2021. Iron oxide nanoparticles effect on growth, physiological traits and nutritional contents of Moringa oleifera grown in saline environment. Bull Natl Res Cent. 45:177. doi:10.1186/s42269-021-00624-9.
- Tombuloglu H, Slimani Y, Tombuloglu G, Alshammari T, Almessiere M, Korkmaz AD, Baykal A, Samia ACS. 2020. Engineered magnetic nanoparticles enhance chlorophyll content and growth of barley through the induction of photosystem genes. Environ Sci Pollut Res Int. 27(27):34311–34321. doi:10.1007/s11356-020-09693-1.
- Wang Q, Xu W, Ren C, Zhan C, Wang C, Li J, Ren Q, Liang X, Wei L, Xiang D, et al. 2023a. Physiological and biochemical mechanisms of exogenous melatonin regulation of saline and ash; alkali tolerance in oats. Agronomy. 13(5):1327. doi:10.3390/agronomy13051327.
- Wang W, Zhang C, Shang M, Lv H, Liang B, Li J, Zhou W. 2022a. Hydrogen peroxide regulates the biosynthesis of phenolic compounds and antioxidant quality enhancement in lettuce under low nitrogen condition. Food Chem. X. 16:100481. doi:10.1016/j.fochx.2022.100481.
- Wang X, Xie H, Wang P, Yin H. 2023b. Nanoparticles in plants: uptake, transport and physiological activity in leaf and root. Materials (Basel). 16(8):3097. doi:10.3390/ma16083097.
- Wang Y, Branicky R, Noë A, Hekimi S. 2018. Superoxide dismutases: dual roles in controlling ROS damage and regulating ROS signaling. J Cell Biol. 217(6):1915–1928. doi:10.1083/jcb.201708007.
- Wang Y, Kang Y, Zhong M, Zhang L, Chai X, Jiang X, Yang X. 2022b. Effects of iron deficiency stress on plant growth and quality in flowering Chinese cabbage and its adaptive response. Agronomy. 12(4):875. doi:10.3390/agronomy12040875.
- Wang Y, Wang J, Guo D, Zhang H, Che Y, Li Y, Tian B, Wang Z, Sun G, Zhang H. 2021. Physiological and comparative transcriptome analysis of leaf response and physiological adaption to saline alkali stress across pH values in alfalfa (Medicago sativa). Plant Physiol Biochem. 167:140–152. doi:10.1016/j.plaphy.2021.07.040.
- Wang Y, Zhou Y, Liu K, Wang N, Wu Y, Zhang C, Ma J. 2023c. Transcriptome-Based comparative analysis of transcription factors in response to NaCl, NaOH, and Na2CO3 stresses in roots of autotetraploid rice (Oryza sativa L.). Agronomy. 13(4):959. doi:10.3390/agronomy13040959.
- Waqas Mazhar M, Ishtiaq M, Maqbool M, Akram R, Shahid A, Shokralla S, Al-Ghobari H, Alataway A, Dewidar AZ, El-Sabrout AM, et al. 2022. Seed priming with iron oxide nanoparticles raises biomass production and agronomic profile of water-stressed flax plants. Agronomy. 12(5): 982. doi:10.3390/agronomy12050982.
- Wu X, Hu Q, Liang X, Fang S. 2022. Fabrication of colloidal stable gliadin-casein nanoparticles for the encapsulation of natamycin: molecular interactions and antifungal application on cherry tomato. Food Chem. 391:133288. doi:10.1016/j.foodchem.2022.133288.
- Xiang F, Xia Y, Wang Y, Wang Y, Wu K, Ni X. 2021. Preparation of konjac glucomannan based films reinforced with nanoparticles and its effect on cherry tomatoes preservation. Food Packag Shelf Life. 29:100701. doi:10.1016/j.fpsl.2021.100701.
- Xu Z, Wang J, Zhen W, Sun T, Hu X. 2022. Abscisic acid alleviates harmful effect of saline–alkaline stress on tomato seedlings. Plant Physiol Biochem. 175:58–67. doi:10.1016/j.plaphy.2022.01.018.
- Yang J, Wang P, Li S, Liu T, Hu X. 2022. Polyamine oxidase triggers H2O2-mediated spermidine improved oxidative stress tolerance of tomato seedlings subjected to saline-alkaline stress. Int J Mol Sci. 23(3):1625. doi:10.3390/ijms23031625.
- Yin B, Zhang J, Liu Y, Pan X, Zhao Z, Li H, Zhang C, Li C, Du X, Li Y, et al. 2019. PtomtAPX, a mitochondrial ascorbate peroxidase, plays an important role in maintaining the redox balance of Populus tomentosa Carr. Sci Rep. 9(1):19541. doi:10.1038/s41598-019-56148-w.
- Yin X, Feng Q, Liu W, Zhu M, Zhang J, Li Y, Yang L, Zhang C, Cui M, Zheng X, et al. 2023. Assessment and mechanism analysis of plant salt tolerance regulates soil moisture dynamics and controls root zone salinity and sodicity in seasonally irrigated agroecosystems. J Hydrol. 617:129138. doi:10.1016/j.jhydrol.2023.129138.
- Zhang H-h, Xu N, Wu X, Wang J, Ma S, Li X, Sun G. 2018. Effects of four types of sodium salt stress on plant growth and photosynthetic apparatus in sorghum leaves. J Plant Interact. 13:506–513. doi:10.1080/17429145.2018.1526978.
- Zhang J, Cao C, Wang Y, Xie L, Li W, Li B, Guo R, Yan H. 2021. Magnesium oxide/silver nanoparticles reinforced poly(butylene succinate-co-terephthalate) biofilms for food packaging applications. Food Packag Shelf Life. 30:100748. doi:10.1016/j.fpsl.2021.100748.
- Zhang M, He S, Zhan Y, Qin B, Jin X, Wang M, Zhang Y, Hu G, Teng Z, Wu Y. 2019a. Exogenous melatonin reduces the inhibitory effect of osmotic stress on photosynthesis in soybean. PLoS One. 14(12):e0226542. doi:10.1371/journal.pone.0226542.
- Zhang Z, He K, Zhang T, Tang D, Li R, Jia S. 2019b. Physiological responses of Goji berry (Lycium barbarum L.) to saline-alkaline soil from Qinghai region, China. Sci Rep. 9:12057. doi:10.1038/s41598-019-48514-5.
- Zheng H, Wang J, Zhang Y, Xv Q, Zeng Q, Wang J. 2022. Preparation and characterization of carvacrol-loaded Caseinate/Zein-composite nanoparticles using the anti-solvent precipitation method. Nanomaterials. 12(13):2189. doi:10.3390/nano12132189.
- Zulfiqar F, Ashraf M. 2023. Proline alleviates abiotic stress induced oxidative stress in plants. J Plant Growth Regul. 42:4629–4651. doi:10.1007/s00344-022-10839-3.