Abstract
The effect of the interaction between myofibrillar protein (MP) and heat-induced soy protein isolates (SPI) on gel properties was examined. To enhance the interaction between MP and SPI, SPI was subjected to thermal treatments at 60, 80, and 95 °C. The results showed that hydrophobic interactions played the most important role in MP-heated SPI (HSPI) gels. Hydrogen bonds played an important role in stabilizing the mixed gels, but this decreased with increasing heat treatment temperature of SPI. Disulfide bonds were not a significant force stabilizing the mixed gels. The gel properties of HSPI-MP were enhanced significantly (P < 0.05) by the inclusion of preheated SPI (95 °C, 30 min), including hardness, springiness, and water-holding capacity (WHC). Dynamic rheological analysis showed that heat treatment decreased the onset temperature of mixed gels and significantly increased the final G’. Notable cross-linked strands formed in MP-HSPI (80 °C) and MP-HSPI (95 °C) gels, while the smoothest and most ordered gel network structure was observed in the MP-HSPI (95 °C) gel.
Se ha examinado el efecto de la interacción entre la proteína miofibrilar y aislados proteicos de soja inducidos con calor en las propiedades del gel. Para mejorar la interacción entre MP y SPI, SPI estuvo sujeto a tratamiento térmico a 60, 80 y 95 ºC. Los resultados mostraron que la interacción hidrofóbica jugó el papel más importante en el gel MP-soja climatizada aislado de proteína (HSPI). Los enlaces de hidrógeno jugaron un papel importante estabilizando la mezcla de geles, aunque disminuyeron con la temperatura del tratamiento de calor de SPI. Los enlaces de disulfuro no fueron una fuerza significativa a la hora de estabilizar la mezcla de geles. Las propiedades del gel de HSPI-MP fueron mejoradas significativamente (P<0,05), incluida la dureza, la ligereza y WHC mediante la inclusión de SPI precalentado (95 ºC, 30 min). El análisis reológico dinámico mostró que el tratamiento de calor redujo las temperaturas de inicio de la mezcla de gel y aumentó significativamente la G’ final. Se formaron áreas de enlaces entrecruzados notables en geles MP-HSPI (80 ºC) y MP-HSPI (95 ºC), mientras que una estructura más ordenada y lisa de gel se observó en el gel MP-HSPI (95 ºC).
1. Introduction
There is a great demand in the sausage manufacturing industry for soy proteins that can reduce formulation cost and improve flavor and textural properties. Soy proteins can interact with meat proteins and occupy the interstitial spaces in a gel or gel matrix, as discrete pockets in the matrix structure (Pietrasik, Jarmoluk, & Shand, Citation2007). Studies have shown that the interaction can occur between dissociated soy 11S protein and myosin heavy chain, but not between myosin and native soy protein (Peng, Dayton, Quass, & Allen, Citation1982). Feng and Xiong (Citation2002, Citation2003) suggested that 7S globulins have an adverse effect on the self-aggregation of myosin heavy chains. Herrero, Carmona, Cofrades, and Jiménez-Colmenero (Citation2008) reported that soy proteins can improve the gel properties of myofibrillar protein (MP), depending on the formulation conditions and the characteristics of the soy protein. The above studies suggested that no interaction occurs between native soy protein and meat protein. Peng and Nielsen (Citation1986) found that the presence of β-conglycinin diminished the aggregation of myosin heavy chains between 50 and 100 °C. Huang et al. (Citation2010) reported that unfolding soy protein isolate (SPI) globulins, cleaving the peptides, and exposing the reactive groups improved interactions between muscle proteins and SPI, and thus improved the gel properties of myofibrillar protein. However, the interaction between denatured soy proteins and muscle proteins, and the effect of such interactions on the gelation of mixed gels, is not yet fully understood.
Evaluating the chemical interactions between myofibrillar proteins and heat-treated soy proteins may clarify the effect of these interactions on the gel properties of mixed proteins. Most meat products are cooked to ~73 °C as a final temperature, at which native soy proteins undergo hardly any structural changes, and hence have minimal interactions with muscle proteins. Once an ordered and homogeneous protein network has been formed, an increase in the temperature to 50–60 °C generates a large increase in hydrophobic interactions, which causes intense “shrinkage” of the network, contributing to the dispersion of the network in a far more heterogeneous way and causing the release of water (Huang et al., Citation2010). At >60 °C, the great majority of protein–protein interactions take place with the head portion of the myosin molecules through disulfide bonds or hydrophobic interactions (Sano, Noguchi, Matsumoto, & Tsuchiya, Citation1990; Taguchi, Ishizaka, Tanaka, Nagashima, & Amano, Citation1987). When extensive bonding occurs, a three-dimensional network is formed, resulting in a gel. The gelation properties of meat paste would therefore be correlated with intermolecular bonds (Liu, Zhao, Xie, & Xiong, Citation2011).
Haga and Ohashi (Citation1984) suggested that disulfide bonds were a significant force between SPI and myosin during heat processing. O’Kane, Happe, Vereijken, Gruppen, and Van Boekel (Citation2004) claimed that hydrogen bonds and hydrophobic interactions played the most important roles in network formation by the legumin proteins, rather than disulfide bonds. Chemical forces were examined in gels between non-meat protein and MP, but results and conclusions from various studies have not been consistent. Our objective was to evaluate the chemical forces between myofibrillar proteins and heat-treated soy proteins, and the gel properties of such mixed proteins, to clarify the effect of MP-SPI interactions on the gel properties of mixed-protein gels.
2. Materials and methods
2.1 Materials
Fresh pork meat (local market) with fat and connective tissue removed was used to prepare MP. SPI was prepared from soybeans (Harbin High-Tech Co., Ltd, China) using an alkaline pH (8.0) extraction followed by isoelectric precipitation (pH 4.5) and neutralization (pH 7.0), then was freeze-dried for 24 h. The protein content of the SPI was ~87.89% as determined by the Kjeldahl method.
2.2 Heat treatment of SPI and properties
2.2.1 Heat treatment of SPI
A protein solution was made by redissolving 10 g of SPI in water (w/v = 1:20), and this solution was adjusted to pH 7.0. The dissolved protein solution was heated by stirring for 30 min at 60 °C, 80 °C, or 95 °C. After the heat treatment, the samples were centrifuged at 10,000g for 30 min to remove the insoluble fraction and then freeze-dried for 24 h.
2.2.2 Differential scanning calorimetry (DSC)
A DSC (Perkin-Elmer Instruments, Shelton, CT, USA) equipped with the Pyris accessory software was used to create differential thermograms of native and heat-treated SPI. Samples of aqueous dispersions (~60 mg) were placed in sealed aluminum pans with a temperature gradient from 20 °C to 120 °C at 5 °C/min; an empty aluminum pan was used as a reference. The enthalpy of denaturation (ΔH) and the temperature of denaturation (TD) were calculated using the DSC software after manually setting the start and end points of the endothermic peak.
2.2.3 Surface hydrophobicity
Surface hydrophobicity was determined according to Kato and Nakai (Citation1980), using 1-anilino-8-naphthalene-sulfonate (ANS) as a fluorescent probe.
2.2.4 Raman spectroscopic analysis
Raman spectra were recorded on a PerkinElmer-RamanStation 400 F dispersive Raman spectrometer. Spectral data from the scans of samples were smoothed, baseline-corrected, and normalized against the phenylalanine band at 1003 ± 1 cm−1 using the Grams 32 software (Galactic Industries Corporation, Salem, NH, USA). The Phe band located near 1003 cm−1 was used as an internal standard to normalize the spectra because it has been reported to be insensitive to the microenvironment (Herrero et al., Citation2008). Quantitative estimation of the secondary structure of SPI under specific conditions was performed using the Peakfit 4.12 software (Seasolve Software, Framingham, MA).
2.3 Extraction of MP
MP was extracted from fresh pork muscle by washing three times with 10 mM Na3PO4, 2 mM MgCl2, 0.1 M NaCl, 1 mM EGTA, and 25 mM sodium phosphate buffer (pH 7.0) and centrifuging for 15 min at 3500 g and 4 °C (Park, Xiong, & Alderton, Citation2007). The extracts were washed two more times with a 0.1 M NaCl solution and centrifuged for 15 min at 3500 g and 4 °C.
2.4 Preparation of MP-SPI gels
For the protein gel system, mixtures of MP with native or heat-treated (60, 80, or 95 °C) SPI were prepared in 0.6 M NaCl and 50 mM phosphate (pH 6.25) to prepare mixed MP-SPI (w/w = 3:1) protein solutions. Protein solutions were heated for 30 min at 80 °C in a thermostatic water bath to form mixed MP-SPI gels. The MP-SPI gels were stored at 4 °C for 24 h until analysis.
The mixed gel prepared by MP and native SPI (NSPI) is referred to as MP-NSPI, and the mixed gels prepared by MP and heat-treated SPI at 60, 80, and 95 °C are, respectively, referred to as MP-heated SPI (HSPI) (60°C), MP-HSPI (80°C), and MP-HSPI (95°C).
2.5 Textural analysis
Texture profile analysis (TPA) of MP-SPI gels was performed in a texture analyzer (TA-XT.Plus, Stable Micro Systems, UK). The operating conditions were: probe P/0.5, compressed distance: 5 mm, pre-test speed: 1.0 mm/s, post-test speed: 1.0 mm/s, applied force: 5 g, and data acquisition rate: 200 pps. Hardness: peak force (N) required for first compression. Cohesiveness: ratio of active work done under the second compression curve to that done under the first compression curve (dimensionless). Springiness: distance (mm) of sample recovery after the first compression. Chewiness: Hd×Ch×Sp (N×mm).
2.6 Water-holding capacity (WHC)
WHC was determined according to Kocher and Foegeding (Citation1993).
where W1 is the initial mass of the protein sample, W2 is the mass after centrifugation, and W is the initial mass of the centrifuge tube containing the heat-treated sample. Measurements were carried out in triplicate, and the mean values are reported.
2.7 Forces in protein gels
The chemical forces involved in mixed gels were determined according to Jiang and Xiong (Citation2013). The protein content extracted by blending with different dissolving solutions was used to indicate the main forces in the gels. The dissolving solutions were 8 M urea and 50 mM sodium phosphate (pH 7.0) to detect hydrogen bonds, 0.5% dodecyl sulfate-sodium salt (SDS) and 50 mM sodium phosphate (pH 7.0) to detect total non-covalent forces, and 0.25% β-mercaptoethanol (β-ME) and 50 mM sodium phosphate (pH 7.0) to detect disulfide bonds.
2.8 Dynamic rheological properties
Viscoelastic properties of MP-SPI proteins solution were measured using a Bohlin VOR rheometer (Bohlin Instruments, Inc., Cranbury, NJ, USA) during heat-induced gelation. Samples were heated at a rate of 1 °C/min from 20 °C to 80 °C. The samples were loaded between two parallel plates (1 mm gap) during heating, and were examined at a constant frequency of 0.1 Hz with a maximum strain of 0.02. The changes in the storage modulus G′ were recorded.
2.9 Microstructural characteristics of the mixed gels
The morphology of MP-SPI gels was determined using a scanning electron microscope (SEM, Model JMS-6360 LV, Hitachi Corp., Tokyo, Japan). MP-SPI gels were cut into cubic pieces and mounted in 2% glutaraldehyde for 24 h at 4 °C. They were then dehydrated using a graded series of ethanol concentrations (50%, 70%, 80%, 90%, and 100%), and then freeze-dried for 15 min. Samples were fixed on an aluminum mount with a conductive carbon disk and adhesive, then coated with gold.
2.10 Statistical analysis
Each treatment was performed in at least triplicate. The results were subjected to a one-way analysis of variance (ANOVA) according to the general linear model procedure with least square means, to determine the significance of the effects. Significant differences (P < 0.05) between means were identified using Duncan’s test.
3. Results and discussion
3.1 Properties of heat-treated SPI
3.1.1 Thermal characteristics of SPI determined by DSC
DSC thermograms of native and heat-treated SPI are presented in . Two endothermic peaks were shown in the NSPI with β-conglycinin and globulin, and were consistent with previously published work (Lakemond, De Jongh, Hessing, Gruppen, & Voragen, Citation2000). The first peak was observed at 74.2 °C and the second at 93.6 °C, attributable to β-conglycinin and globulin denaturation, respectively. After a thermal treatment at 60 °C, there were no remarkable changes in the endothermic peak or ΔH. However, after heating at 80 °C, the endothermic peak of β-conglycinin disappeared indicating complete denaturation of the β-conglycinin component, and ΔH of the component decreased slightly by ~12%. These results suggest that protein conformation was partially affected by thermal treatment at 80 °C, with hydrophobic cores initially buried in the interior exposed and the partially dissociated globulin components refolded to form more stable aggregates (Tang, Choi, & Ma, Citation2007). The sample of SPI heated for 30 min at 95 °C showed no endothermic peak, which illustrated that β-conglycinin and globulin were completely denatured. The interaction between MP and soy protein seemed to be enhanced by the denaturation of β-conglycinin and globulin, and exposure of hydrophobic cores in the SPI.
Table 1. DSC characteristics and surface hydrophobicity of heat-treated SPI.
Tabla 1. Características de DSC y hidrofobia de la superficie de SPI tratado con calor.
3.1.2 Surface hydrophobicity of SPI
As shown in , heat treatment exhibited a notable influence on surface hydrophobicity of SPI. Increased surface hydrophobicity of SPI heated at 80 °C or 95 °C was mainly related to denaturation of β-conglycinin and globulin. The other factors causing an increase in surface hydrophobicity may be the dissociation of protein subunits or expansion of peptide chains. Heating at 60 °C generally affected the structure of globulin and β-conglycinin, causing disruption of secondary and tertiary structures of SPI, uncoiling of polypeptide chains, and exposure of hydrophobic sites, which lead to an increase in surface hydrophobicity. The increased surface hydrophobicity of SPI may be the primary reason for the improved interaction between MP and soy protein.
3.1.3 Raman spectroscopic analysis
lists the secondary structure content percentages from samples analyzed. The results showed that non-HSPI contained 17.87% α-helix, 35.33% β-sheet, 11.59% turns, and 35.21% unordered structures. There was a progressive increase in the α-helical structure and a general decrease in the β-sheet structure of HSPI in comparison with non-HSPI (). The protein secondary structural changes observed upon heating (a decrease in the β-sheet structure) are consistent with the literature (Mills et al., Citation2003).
Table 2. Percentages of protein secondary structure: α-helix, β-sheet, β-turn and unordered structure of soy protein isolates under different heating conditions.
Tabla 2. Porcentajes de la estructura secundaria proteínica: α-hélice, β-lámina beta, β-giro y aislados proteicos de soja desordenados a diferentes coldiciones de calentamiento.
There was a marked increase in the unordered structures and a slight decrease in β-turns in SPI heated at 60 °C. When SPI was heated at 80 °C, there was a significant increase in the α-helical structure accompanied by a decrease in the β-sheet and unordered structure, which may be attributed to denaturation of β-conglycinin. A transformation from the unordered and β-sheet structure to the β-turn structure was observed at 95 °C, which was due to denaturation of 11S globulin. The changes in the SPI structure upon different heat-treatments can affect the interaction between MP and soy protein.
3.2 Chemical forces
When sufficient bonding occurs, a three-dimensional network is formed, resulting in the formation of a gel (Lanier, Yongsawatdigul & Carvajal-Rondanelli, Citation2013). In this study, protein solubility of the mixed MP-SPI gels was measured after blending with dissolving solutions containing different force-disrupting agents, to identify the possible physicochemical bonds and interactions stabilizing the gels (). The mixed gels were treated with β-ME to clarify the role of disulfide linkages. The result showed no substantial difference in protein solubility of the mixed gels treated with β-ME, which suggested that disulfide bonds were not a significant force responsible for stabilizing the mixed gels with HSPI. Renkema and Van Vliet (Citation2002) reported that disulfide bridges, being covalent bonds, have high bond strength compared to non-covalent bonds, and will therefore not be broken during heating of muscle proteins, notably myosin. O’Kane et al. (Citation2004) claimed that the network formation by the legumin proteins was closely related to hydrogen bonds and hydrophobic interactions, but disulfide bonds had minimum involvement.
Figure 1. Protein content extracted of mixed gels in 50 mM phosphate buffer containing different chemicals.Note: samples without a common letter (a–g) differ significantly (P<0.05).
Figura 1. Contenido proteínico extraído de la mezcla de geles en 50 mM tampón fosfato que contenía diferentes sustancias químicas.
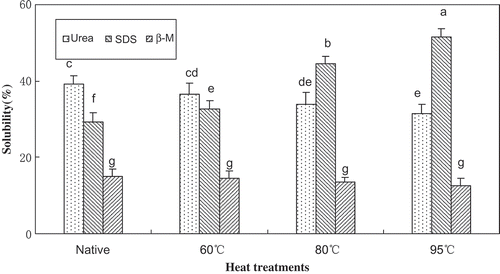
When the gels were blended with 8 M urea, hydrogen bonds, which are known to play an important role in stabilizing protein conformation, were destroyed. The protein solubility of MP-HSPI gels treated with urea was lower than that of MP-NSPI gels, and the protein solubility of MP-HSPI gels treated with urea decreased with increasing treatment temperature. Raman spectroscopic analysis in this study also showed a decrease in the β-sheet structure, indicating the collapse of the intramolecular hydrogen bonds of SPI. Furthermore, a previous study suggested that heating proteins above their thermal denaturation temperature would result in the total destruction of both the tertiary and the hydrogen-bonded secondary structures in proteins (Huang et al., Citation2010), leading to a decrease in protein solubility of MP-HSPI gels treated with urea. In this study as well, hydrogen bonds were shown to play an important role in stabilizing the mixed gels, but were not the predominant force.
Hydrophobic interactions play an important role in the stabilization of the quaternary structure of proteins and favor the formation of harder gel/emulsion structures. When the samples were solubilized in 0.5% SDS, less protein was solubilized from the MP-SPI gel with NSPI than the other gels. This could be because a significant portion of the SDS was consumed to unravel the compact SPI (which was largely non-gelling) rather than loosening the hydrophobic associations in the gel network (Jiang & Xiong, Citation2013). However, a significantly higher amount (P < 0.05) of protein was extracted from MP-HSPI gels, indicating that hydrophobic residues play a vital role in MP-HSPI gels. The large quantity of undissociated β-conglycinins in MP-NSPI and MP-HSPI (60°C) seemed to hinder the hydrophobic interactions of SPI with myosin heavy chains. However, heating SPI at 80 °C or 95 °C can break the intermolecular hydrogen bonding and lead to denatured β-conglycinin and globulin, increasing protein solubility of the mixed gels significantly. Furthermore, heating SPI at 95 °C can significantly reduce the β-sheet content, which facilitates maintaining the hydrophobic amino acids in the internal structure. The previous study showed that due to their relatively large surface areas for ordered hydrogen bonding, β-sheet structures may be influenced by hydrophobic interactions that are essential to the stability, conformation, and function of proteins (Lee, Choi, & Lee, Citation2002). More hydrophobic amino acid residues can be exposed by increased heating temperatures, which can further improve the formation of aggregates among protein molecules and enhance the interaction between the SPI and MP. The research of Mleko and Foegeding (Citation2000) showed that the presence of hydrophobic forces was responsible for the increase in shear moduli of whey protein gels during heating. Therefore, it can be concluded that hydrophobic interactions in mixed gels containing heat-treated SPI played a crucial role in improving the gel properties.
3.3 Textural properties
Four parameters of the complex gels were obtained by TPA: hardness, springiness, chewiness, and cohesiveness. shows the results of TPA of complex gels. The hardness could be increased significantly (P < 0.05) by 19.9% in MP-HSPI (80°C) and by 44.3% in MP-HSPI (95°C). The springiness was also significantly improved, increasing by 13.16% in MP-HSPI (80°C) and 27.63% in MP-HSPI (95°C). The heat-induced increase in surface hydrophobicity enhanced the hydrophobic interactions between SPI and the heavy chain of myosin (the most important component of meat proteins) forming a stiff, elastic, stable network structure.
Table 3. Texture profile analysis of mixed MP-SPI gels.
Tabla 3. Análisis del perfil de textura de mezclas de geles MP-SPI.
The hardness and springiness of MP-HSPI (95°C) gels were higher than those of the other gels. Heat treatment at 95 °C could improve the rate of mixed protein denaturation in heat-induced gelation. When the rate of protein denaturation is higher than the rate of aggregation, an orderly, stable, and elastic gel network is formed, which is consistent with the SEM study results. In addition, the acid and alkaline subunits of globulin were dissociated at 95 °C, and the dissociated alkaline subunits can interact with myosin via hydrophobic interactions, which enhanced the hardness and springiness. Huang et al. (Citation2010) reported that hydrophobic interactions had a significant positive correlation with gel strength. This study showed that the hardness and springiness of mixed gels were enhanced by strengthening hydrophobic interactions and weakening the hydrogen bonding. It can be deduced that hydrophobic interactions were advantageous to enhance hardness and springiness; however, hydrogen bonding had an adverse effect on the hardness and springiness.
However, the MP-HSPI (60°C) sample had no significant improvement in hardness or springiness. A plausible explanation for this phenomenon is that both NSPI and SPI heated at 60 °C, with their compact structure as shown by ΔH in DSC, had a detrimental effect on MP gelation due to interference with myosin interaction. Moreover, the chewiness of the complex gels was improved (P < 0.05) when heated at 60 °C, 80 °C, and 90°C, increasing by 1.06, 4.02, and 9.71 N×mm respectively. However, the cohesiveness of the complex gels was not affected regardless of the heat-treatment applied.
3.4 Dynamic rheological properties
The mechanical spectra for the gelation behavior of mixed proteins during heating from 20 °C to 80 °C are shown in . G′ of all the mixed solutions started to increase at approximately 40 °C, revealing the formation of an elastic protein network structure or the onset of gelation. G′ reached its maximum value at ~45 °C. Upon further heating, the G′ values decreased significantly and reached their minimum values. The drop in G′ has been attributed to denaturation of the myosin subfragments’ light meromyosin (LMM) and subfragment-1 (S-1), which leads to increased fluidity (Brenner, Johannsson, & Nicolai, Citation2009). The second increase in G′ after 55 °C has been attributed to the formation of permanent, irreversibly cross-linked filaments and a stable three-dimensional structure due to covalent disulfide bonding and hydrophobic interactions (Liu, Bao, Xi, & Miao, Citation2014). In this research, hydrophobic interactions may be one important factor influencing the change in G′ after 55 °C, since little disulfide bonding was found in mixed gels.
Figure 2. Representative rheograms of heat-induced mixed MP-SPI gels.
Figura 2. Reogramas representativos de la mezcla de geles MP-SPI inducida con calor.
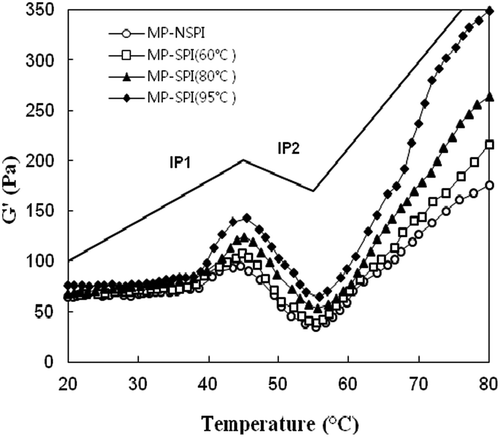
The addition of heat-treated soy protein affected the pattern of rheological transition. The changes in G′ can be divided into three series by two inflection points (IPs) that are characteristic of the specific treatment curve. The temperature of IP1 and IP2, respectively, increased from 44.73 °C to 45.98 °C and from 55.31 °C to 56.22 °C. A similar heating curve was observed by Tahergorabi, Beamer, Matak, and Jaczynski (Citation2012), who reported the influence of TiO2 on the gel-forming ability of proteins recovered by isoelectric solubilization/precipitation. More strikingly, the final G′ of mixed gels was greatly improved upon the addition of heat-treated SPI. A reasonable explanation for the above phenomenon is that soy proteins, heat-treated or not, had an excluded volume effect resulting from their competition for water absorption (McClements, Citation2002), causing MP to aggregate at reduced temperatures. The rigidity of a protein gel is determined by the amount of particles (aggregates) incorporated into the gel network and the strength of the interactions between these particles. However, while the network was continuously formed, the availability of reactive side chain groups in heat-treated SPI promoted the interaction of MP and SPI proteins, leading to a stronger and more elastic final gel structure.
3.5 WHC
The gelling process entails the association of myosin chains, which produce a continuous three dimensional network in which water is trapped. WHC can indicate the ability of protein to bind water, and is generally used to evaluate the quality and yield of meat and its products.
lists the WHC of the mixed gels containing various SPI. It can be seen that the WHC of mixed gels increased significantly (P < 0.05) when made with heat-treated SPI. The WHC of MP-HSPI (60°C), MP-HSPI (80°C), and MP-HSPI (95°C) gels increased 5.89%, 17.94%, and 27.55% respectively, compared with the MP-NSPI gel. This may be attributed to the following two reasons. Firstly, the WHC of gels is related closely with the microstructure. The mixed gel with NSPI had an agglomerate structure, which was adverse to water bonding. Secondly, in general, heating may result in the unfolding of native protein and exposure of buried polar groups, enhancing protein–protein interactions by hydrophobic association. Moderate hydrophobic interactions favored gel matrix formation (Liu et al., Citation2011), and the matrix formed had a greater ability to entrap water.
Table 4. Water-holding capacity of mixed MP-SPI gels.
Tabla 4. Capacidad de retención de agua de la mezcla de geles MP-SPI.
Moreover, WHC improved significantly in MP-HSPI (80°C) and especially in MP-HSPI (95°C) gels, compared to MP-HSPI (60°C). Kristinsson and Hultin (Citation2003) suggested that electrostatic repulsion plays a major role in WHC. It can be deduced that denaturation of β-conglycinin and globulin may enhance repulsion which promotes the extension of the myosin chain. On the other hand, heating at 95 °C can not only induce the dissociation of β-conglycinin, but also globulin subunits, and cause the exposure of additional reactive groups that are beneficial for protein-protein interaction and formation of the gel matrix.
3.6 Scanning electron microscopy
The SEM images of cross sections of MP-SPI gels are shown in . Remarkable variations were observed in the microstructure of complex gels with different heat-treated SPI. The MP-NSPI gel showed an obvious agglomerate and inhomogeneous microstructure without any cross-linked strands. In such cases, the microstructure illustrated that interactions did not occur between NSPI and MP. Moreover, the agglomerate phenomenon also occurred in MP-HSPI (60°C), but some cross-linked strands can be seen.
Figure 3. Scanning electron micrographs (magnification: 1000×) of mixed MP-SPI gels. (a) MP-NSPI; (b) MP-HSPI(60°C); (c) MP-HSPI(80°C); (d) MP-HSPI(95°C).
Figura 3. Micrografías electrónicas de barrido (aumento: 1000×) de la mezcla de geles MP-SPI.
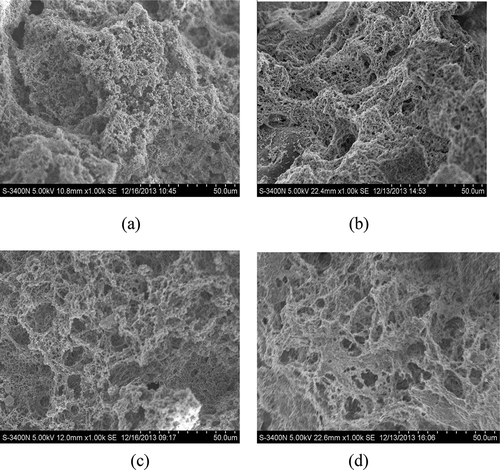
Obvious cross-linked strands can be found in MP-HSPI (80°C) and MP-HSPI (95°C) gels. A smoother and more ordered network structure was shown in the MP-HSPI (95°C) gel, while the MP-HSPI (80°C) gel showed a coarse gel network with irregular holes. Hermansson (Citation1979) reported that gel properties of proteins depend on the relative speeds of unfolding and aggregation. When the rate of protein aggregation is slower than denaturation, heat-denatured proteins are allowed to align in an ordered fashion to form a fine gel network, which can hold more water. In contrast, a coarse gel network would be formed if the rate of aggregation was faster than denaturation. Thus, preheating treatments at 95 °C increase the rate of denaturation of β-conglycinin and globulin, endowing the MP-HSPI (95°C) gel with a smoother and more ordered network structure. It can be deduced that denaturation of globulin may play an important role in the formation of cross-linking and a more regular gel network.
4. Conclusion
Heat treatment of SPI can significantly enhance the interaction between MP and SPI, improving the gel properties of a mixed gel. The results showed that hydrophobic interactions played the more important role in stabilizing MP-HSPI gels, which were advantageous to enhance textural properties and G′. In addition, hydrogen bonds played a subordinate role in stabilizing the mixed gels, but disulfide bonds had minimal involvement.
Disclosure statement
No potential conflict of interest was reported by the authors.
Additional information
Funding
References
- Brenner, T., Johannsson, R., & Nicolai, T. (2009). Characterisation and thermo-reversible gelation of cod muscle protein isolates. Food Chemistry, 115(1), 26–31. doi:10.1016/j.foodchem.2008.11.046
- Feng, J., & Xiong, Y. L. (2002). Interaction of myofibrillar and preheated soy proteins. Journal of Food Science, 67(8), 2851–2856. doi:10.1111/j.1365-2621.2002.tb08827.x
- Feng, J., & Xiong, Y. L. (2003). Interaction and functionality of mixed myofibrillar and enzyme-hydrolyzed soy proteins. Journal of Food Science, 68(3), 803–809. doi:10.1111/j.1365-2621.2003.tb08246.x
- Haga, S., & Ohashi, T. (1984). Heat-induced gelation of a mixture of myosin B and soybean protein. Agricultural and Biological Chemistry, 48, 1001–1007. doi:10.1271/bbb1961.48.1001
- Hermansson, A. M. (1979). Aggregation and denaturation involved in gel formation. In A. Pour-El (Ed.), Functionality and protein structure (pp. 81–103). Washington, DC: American Chemical Society.
- Herrero, A. M., Carmona, P., Cofrades, S., & Jiménez-Colmenero, F. (2008). Raman spectroscopic determination of structural changes in meat batters upon soy protein addition and heat treatment. Food Research International, 41(7), 765–772. doi:10.1016/j.foodres.2008.06.001
- Huang, X., Li, C., Yang, F., Xie, L., Xu, X., Zhou, Y., … Pan, S. (2010). Interactions and gel strength of mixed myofibrillar with soy protein, 7S globulin and enzyme-hydrolyzed soy proteins. European Food Research and Technology, 231(5), 751–762. doi:10.1007/s00217-010-1329-0
- Jiang, J., & Xiong, Y. L. (2013). Extreme pH treatments enhance the structure-reinforcement role of soy protein isolate and its emulsions in pork myofibrillar protein gels in the presence of microbial transglutaminase. Meat Science, 93(3), 469–476. doi:10.1016/j.meatsci.2012.11.002
- Kato, A., & Nakai, S. (1980). Hydrophobicity determined by fluorescence probe method and its correlation with surface properties of proteins. Biochimica et Biophysica Acta (BBA)-Protein Structure, 624(1), 13–20.
- Kocher, P., & Foegeding, E. (1993). Microcentrifuge-based method for measuring water-holding of protein gel. Journal of Food Science, 58(5), 1040–1046.
- Kristinsson, H. G., & Hultin, H. O. (2003). Role of pH and ionic strength on water relationships in washed minced chicken-breast muscle gels. Journal of Food Science, 68(3), 917–922. doi:10.1111/j.1365-2621.2003.tb08265.x
- Lakemond, C. M., De Jongh, H. H., Hessing, M., Gruppen, H., & Voragen, A. G. (2000). Heat denaturation of soy glycinin: Influence of pH and ionic strength on molecular structure. Journal of Agricultural and Food Chemistry, 48(6), 1991–1995. doi:10.1021/jf9908704
- Lanier, T. C., Yongsawatdigul, J., & Carvajal-Rondanelli, P. (2013). Surimi gelation chemistry. In J. W. Park (Ed.), Surimi and surimi seafood (pp. 101). New York, NY: Marcel Dekker.
- Lee, H.-J., Choi, C., & Lee, S.-J. (2002). Membrane-bound α-synuclein has a high aggregation propensity and the ability to seed the aggregation of the cytosolic form. Journal of Biological Chemistry, 277(1), 671–678. doi:10.1074/jbc.M107045200
- Liu, Q., Bao, H., Xi, C., & Miao, H. (2014). Rheological characterization of tuna myofibrillar protein in linear and nonlinear viscoelastic regions. Journal of Food Engineering, 121, 58–63. doi:10.1016/j.jfoodeng.2013.08.016
- Liu, R., Zhao, S.-M., Xie, B.-J., & Xiong, S.-B. (2011). Contribution of protein conformation and intermolecular bonds to fish and pork gelation properties. Food Hydrocolloids, 25(5), 898–906. doi:10.1016/j.foodhyd.2010.08.016
- McClements, D. J. (2002). Modulation of globular protein functionality by weakly interacting cosolvents. Critical Reviews in Food Science and Nutrition, 42(5), 417–471. doi:10.1080/20024091054210
- Mills, E. N., Marigheto, N. A., Wellner, N., Fairhurst, S. A., Jenkins, J. A., Mann, R., … Belton, P. S. (2003). Thermally induced structural changes in glycinin, the 11S globulin of soya bean (Glycine max)—An in situ spectroscopic study. Biochimica et Biophysica Acta (BBA)-Proteins and Proteomics, 1648(1), 105–114.
- Mleko, S., & Foegeding, E. A. (2000). pH induced aggregation and weak gel formation of whey protein polymers. Journal of Food Science, 65(1), 139–143. doi:10.1111/j.1365-2621.2000.tb15969.x
- O’Kane, F. E., Happe, R. P., Vereijken, J. M., Gruppen, H., & Van Boekel, M. A. (2004). Heat-induced gelation of pea legumin: Comparison with soybean glycinin. Journal of Agricultural and Food Chemistry, 52(16), 5071–5078. doi:10.1021/jf035215h
- Park, D., Xiong, Y. L., & Alderton, A. L. (2007). Concentration effects of hydroxyl radical oxidizing systems on biochemical properties of porcine muscle myofibrillar protein. Food Chemistry, 101(3), 1239–1246. doi:10.1016/j.foodchem.2006.03.028
- Peng, I. C., Dayton, W. R., Quass, D. W., & Allen, C. E. (1982). Studies on the subunits involved in the interaction of soybean 11S protein and myosin. Journal of Food Science, 47(6), 1984–1990.
- Peng, I. C., & Nielsen, S. S. (1986). Protein-protein interactions between soybean beta-conglycinin (B1-B6) and myosin. Journal of Food Science, 51(3), 588–59. doi:10.1111/j.1365-2621.1986.tb13886.x
- Pietrasik, Z., Jarmoluk, A., & Shand, P. J. (2007). Effect of non-meat proteins on hydration and textural properties of pork meat gels enhanced with microbial transglutaminase. LWT-Food Science and Technology, 40(5), 915–920. doi:10.1016/j.lwt.2006.03.003
- Renkema, J. M., & Van Vliet, T. (2002). Heat-induced gel formation by soy proteins at neutral pH. Journal of Agricultural and Food Chemistry, 50(6), 1569–1573. doi:10.1021/jf010763l
- Sano, T., Noguchi, S. F., Matsumoto, J. J., & Tsuchiya, T. (1990). Effect of ionic strength on dynamic viscoelastic behavior of myosin during thermal gelation. Journal of Food Science, 55(1), 51–54. doi:10.1111/j.1365-2621.1990.tb06014.x
- Taguchi, T., Ishizaka, H., Tanaka, M., Nagashima, Y., & Amano, K. (1987). Protein-protein interaction of fish myosin fragments. Journal of Food Science, 52(4), 1103–1104. doi:10.1111/j.1365-2621.1987.tb14287.x
- Tahergorabi, R., Beamer, S. K., Matak, K. E., & Jaczynski, J. (2012). Functional food products made from fish protein isolate recovered with isoelectric solubilization/precipitation. LWT-Food Science and Technology, 48(1), 89–95. doi:10.1016/j.lwt.2012.02.018
- Tang, C.-H., Choi, S.-M., & Ma, C.-Y. (2007). Study of thermal properties and heat-induced denaturation and aggregation of soy proteins by modulated differential scanning calorimetry. International Journal of Biological Macromolecules, 40(2), 96–104. doi:10.1016/j.ijbiomac.2006.06.013