ABSTRACT
Soluble aggregates, instead of insoluble gel network, were formed on heat-treated chicken breast myosin through alkaline pH-induced electrostatic regulation. Heat treatment of myosin at pH 9.0 significantly increased solubility, decreased turbidity, and resulted in the highest content at 260 nm particle size compared with those at pH 7.0 and 8.0. Results indicated that soluble myosin aggregates were produced at pH 9.0. The surface hydrophobicity and reactive sulfhydryl (R–SH) of unheated sample revealed gradual increases with increased pH. This increase was related to the decreased α-helical, thereby suggesting myosin unfolding. After heating, treatment at pH 9.0 showed the lowest surface hydrophobicity and R–SH, which may contribute to the highest dispersibility. Moreover, heated myosin (pH 9.0) after reshifting pH still exhibited excellent stability, which indicated the irreversible soluble aggregates formation. The controlled production of aggregates may provide the possibility of using soluble aggregates as ingredients for expanded meat protein application in beverage products.
RESUMEN
En el presente estudio se formaron agregados solubles —en vez de una red de gel insoluble— a partir de la miosina de pechuga de pollo tratada térmicamente mediante regulación electrostática alcalina inducida por el pH. En comparación con tratamientos a un nivel de pH de 7.0 y 8.0, el tratamiento térmico de miosina con un pH de 9.0 aumentó significativamente su solubilidad, disminuyó su turbidez y produjo el contenido más alto, obteniéndose partículas de un tamaño de 260 nm. Los resultados indican que se formaron agregados de miosina solubles a un nivel de pH de 9.0. En la muestra no sujeta a tratamiento térmico, la hidrofobicidad superficial y el sulfhidrilo reactivo (R-SH) mostraron incrementos graduales al elevarse el pH. Este aumento está relacionado con la α-helical disminuida, lo cual sugiere que tuvo lugar el desdoblamiento de la miosina. Tras el calentamiento, el tratamiento a un nivel de pH de 9.0 presentó hidrofobicidad superficial y R-SH más bajas, lo cual puede contribuir a la mayor dispersibilidad. Asimismo, después de variar el pH, la miosina calentada (pH 9.0) siguió exhibiendo una excelente estabilidad, lo que da cuenta de la formación irreversible de agregados solubles. La producción controlada de agregados puede dar pie al posible uso de agregados solubles como ingredientes para la aplicación de mayores cantidades de proteína cárnica en productos de bebidas.
PALABRAS CLAVE:
1. Introduction
Meat is an important source of animal-derived protein for human consumption. Meat also contains all the dietary essential amino acids, and it particularly presents high value of biological protein (Pereira & Vicente, Citation2013). In view of food materials science, considerable attention has been given to the thermal-induced gelation properties of meat protein. Myosin, a major muscle protein, is widely regarded as the bearer of gel properties in meat proteins (Chen, Tume, Xu, & Zhou, Citation2017). This state is composed of two heavy chains of approximately 220 kDa and four light chains reaching from about 16 to 25 kDa each (Kristinsson & Hultin, Citation2003a). The two heavy chains form the backbone of a tail and two globular heads. Although gel meat products are common in the market, the application of meat protein as food material in other fields is considerably rare. Hence, meat proteins should be given special attention in functional studies in food materials science.
In recent years, the formation of soluble protein aggregates has been studied. Thermal aggregation of whey proteins remarkably improves thermal stability and the physicochemical and emulsifying properties (Ryan, Zhong, & Foegeding, Citation2013; Shen, Fang, Gao, & Guo, Citation2017). Considering the potential applications of soluble protein aggregation in beverage industry, such as impaction on the rheology of colloidal systems (Pan & Zhong, Citation2016), research on the soluble aggregation of meat protein is valuable. Furthermore, particles exhibit different network formation models. Thus, if meat protein is modified to soluble particle, novel incorporation effects may occur on the food colloidal system. However, research on heat-induced soluble meat protein aggregates is challenging because of the distinct solubility and thermal aggregation properties of meat proteins. Purified myosin exists in insoluble state unless high concentration salt is used to disrupt interaction (Shimada, Takai, Ejima, Arakawa, & Shiraki, Citation2015). Under salt-soluble condition, myosin can be easily heated to form an aggregate-type gel (Yamamoto, Citation1990). The thermal aggregation of myosin molecules can be divided into two steps: oligomer formation through disulfide bridge and oligomer aggregation to form large clusters (Tazawa, Kato, Katoh, & Konno, Citation2002). According to Mcswiney, Singh, and Campanella (Citation1994), soluble aggregates are described as “protein intermediates between the monomers and the insoluble gel network”. Therefore, the formation of meat protein-based soluble aggregates requires precise parameter control. Protein aggregation can be influenced with many factors, such as temperature, ionic strength, and pH (Kristinsson & Hultin, Citation2003b). The thermal aggregation-induced insolubility of muscle myosin limits its further application. For example, it is unfavorable to the flow of protein in the pipeline and the stability of some emulsion procucts. Nevertheless, similar to milk production, thermal processing is an essential step to extend the shelf life and improve the quality of this complex biological fluid by reducing the microbial load; consequently, the risk of food poisoning is minimized. Therefore, we need to find a way, perhaps pH-shifting, that can inhibit protein aggregation during thermal processing. Gel formation, instead of soluble aggregation, may occur during the thermal aggregation of myosin if sufficient protein concentration is reached. pH is a crucial factor to the structure and function of proteins. When pH is adjusted to alkaline condition, the folded myosin could convert into an unfolded state (Kristinsson & Hultin, Citation2003a). Numerous studies reported that pH-induced conformational changes can qualitatively change the thermal aggregation of proteins (Anema and Li, Citation2003; Howell, Matthews, & Donnelly, Citation1991). Thus, myosin soluble aggregates can also be obtained by regulating pH. Many protein-soluble aggregates were realized in this manner (Giroux, Houde, & Britten, Citation2010; K. N. Ryan & Foegeding, Citation2015). Nonetheless, to the best of our knowledge, considerably few meat protein-related studies have been conducted.
The present study aimed to investigate the effects of pH and heating on myosin aggregation, especially the formation of soluble aggregates. The conformation and physicochemical properties of the protein before and after thermal treatment were elucidated. The correlative phenomenon was also explained.
2. Materials and methods
2.1 Materials
Three-Yellow Chickens (4-month old) were purchased from the local food market (NanJing, China). NaCl, NaOH, HCl, and the chemicals used for buffers were purchased from JianLiang (NanJing, China, analytical grade). All other chemicals used were of analytical or better grade.
2.2 Myosin preparation
After a complete rest, the three yellow chickens were electrically stunned and sacrificed by severance of the jugular veins. Then the chicken breast muscle (Pectoralis major) was removed immediately. All animal procedures were reviewed and approved by the Animal Care and Use Committee of the College of Food Science and Technology of NanJing Agricultural University at the Chinese Academy of Agricultural Sciences. Myosin was prepared by using a modified procedure originally described, previously (Hayakawa, Ito, Wakamatsu, Nishimura, & Hattori, Citation2009). All steps were performed at 0–4°C in a cold chamber. In brief, minced muscle (prerigor state) was extracted with three volumes of modified Guba–Straub solution (0.5 M KCl, 100 mM KH2PO4, 50 mM K2HPO4, 5 mM EDTANa2, and 4 mM sodium pyrophosphate dissolved in Milli-Q water; pH 6.5) for 15 min and centrifuged at 5,000 g for 10 min (Beckman Avanti J-E, Beckman Coulter, Fullerton, CA, USA). The supernatant was obtained by filtering through three layers of cheesecloth and subsequent dilution with 10 volumes of cold distilled water. After standing for 6 h, floating materials were removed via siphoning. Precipitated proteins were collected by centrifugation at 8000 g for 10 min. After centrifugation, the sediments were dissolved in KCl (0.3 M) at pH 7. The corresponding solution was centrifuged at 10,000 g for 20 min. The supernatant was obtained and diluted with 10 volumes of cold distilled water. After standing overnight, the proteins used in this study were obtained by centrifugation at 8,000 g for 10 min. The purity of the proteins was more than 90% according to the densitometry analysis of SDS-PAGE strip (Quantity One 1-D analysis software, Bio-Rad Co., Hercules, CA, USA).
2.3 Sample preparation
Three samples of myosin solution were prepared as follows: Myosin was suspended in 20 mM pH7.0 phosphate buffer (PBS) containing 0.6 M NaCl. To ensure complete rehydration, samples were stirred at a controlled temperature in a storage room overnight. After dissolution, the myosin suspensions were prepared at three different pH values (7.0, 8.0, and 9.0) by using 1M NaOH. To evaluate the thermal aggregation of myosin, proteins (10 mg/ml) from the treatments were maintained in a water bath at 75°C for 30 min. Samples before or after thermal treatment were stored at 4°C prior to analysis.
2.4 Myosin solubility and turbidity
Samples were centrifuged at 20,000 g for 20 min. The original whole suspensions and the supernatants were determined by the Biuret method (Gornall, Bardawill, & David, Citation1949). Myosin solubility (5 mg/ml) was calculated according to the method of Chen et al. (Citation2016). Myosin turbidity (5 mg/ml) was monitored as the increase in optical density at 340 nm in a microplate reader (SpectraMax M2, Molecular Devices Limited, USA). In the present study, each measurement was the mean of three replicates.
2.5 Dynamic light scattering (DLS)
The particle size distribution of myosin in solution was determined using the DLS technique (Chen et al., Citation2016). The measurement was performed at a detection angle of 90° using a Zetasizer Nano ZS 90 (Malvern Instruments, Worcestershire, UK) equipped with a 4 mW He–Ne ion laser (λ = 633 nm). Samples were diluted to a protein concentration of 0.5 mg/ml and subsequently placed in a quartz cuvette with 1 cm path length. In this article, the particle size distribution is a plot of the relative volume ratio of particles with their mean sizes.
2.6 Differential scanning calorimetry (DSC)
The thermodynamic properties of myosin were evaluated using a VP-capillary DSC platform (MicroCal, Malvern Instruments Ltd., CA, USA). The method of the DSC measurement was according to Zhang, Yang, Zhou, Zhang, and Wang (Citation2017), with a few modifications. Myosin in NaCl buffer (0.6 M, pH 7.0) was adjusted to a pH of 7.0, 8.0, and 9.0 using NaOH. Protein concentration (10 mg/ml) was studied from 20°C to 90°C with a scanning rate of 1°C/min. The feedback mode was set to none. The data acquisition frequency was set to 0.125 s−1.
2.7 Reactive sulfhydryl (R–SH) group
The R–SH was measured according to the method of Guo, Peng, Zhang, Liu, and Cui (Citation2015). Each sample was diluted to 1 mg/ml using NaCl (0.6 M) buffer. Afterward, one aliquot (20 µl) of 5,5′-dithiobis-2-nitrobenzoic acid was added to 5 ml of soluble myosin. After fully mixing, the mixture was incubated at 25°C for 1 h. The absorbance at 412 nm was recorded on a microplate reader. The R–SH content was calculated using a molar extinction coefficient of 13,600 M−1cm−1. Each sample was performed in triplicates.
2.8 Surface hydrophobicity
The surface hydrophobicity of myosin was determined by the hydrophobic probe-binding method of Creamer, Zoerb, Olson, and Richardson (Citation1982). 1-Anilinonaphthalene-8-sulphonic acid (ANS) was used as the fluorescence probe to characterize this effect. Similar to the measurement of sulfhydryl group, samples were diluted to 1 mg/ml. Approximately 20 µl of ANS solution (15 mM ANS in 0.1 M phosphate buffer, pH 7.0) was added to 4 ml of the sample. After incubation at 25°C for 20 min, the fluorescence intensity was determined with a microplate reader using an excitation wavelength of 375 nm and an emission wavelength of 410–570 nm. Measurements were conducted at room temperature (25°C).
2.9 Circular dichroism (CD)
Far-UV CD spectra were obtained using a Chriascan spectrometer (Applied Photophysics, Surrey, UK). The method of CD measurement was according to Chen et al. (Citation2016). The myosin solution (0.2 mg/ml) was transferred to a quartz cell with 0.1 cm of path length and subsequently scanned from 200 nm to 260 nm at a scan rate of 100 nm/min. The temperature at the time of measurement was maintained at 20°C with the aid of a control unit. Three scans were performed, and the spectra presented in this study were their average. The α-helix (%) was calculated on the basis of the obtained spectra and the protein secondary structure estimation program (CDNN method) provided with the Chriascan spectrometer.
2.10 Myosin-based solution stability after pH cycling
The samples (10 mg/ml, pH 7.0, 8.0, and 9.0) after thermal treatment (75°C, 30 min) were adjusted to pH 7 using 1 M HCl. Solubility was determined after pH cycling. The visual appearance of the solutions (myosin solution at pH 7 without any treatment) was also used to evaluate stability. Solutions were transferred into glass bottles and stored at room temperature (20°C) in the dark. The visual appearance of the samples after 1 and 5 days was recorded by taking photographs.
Statistical analysis
Unless otherwise specified, three independent tests are performed. The resutls are presented as means ± standard deviations (SD). Analysis of variance was performed on the Statistical Analysis System (Version 8.0). Significant differences between means were identified using Duncan’s multiple range test (p < 0.05).
3. Results and discussion
3.1 Effect of alkaline pH on the optical properties of myosin solution before and after thermal treatment
3.1.1 Solubility and turbidity of myosin in solution
The solubility of myosin in the unheated group slightly increased from 80.5% to 84.5% with the increase of pH to approximately 9. In the heated group, the solubility apparently increased from 6.8% to 90.8% (). Using NaOH to raise the pH from netural to away from the isoelectric point of myosin (pI = 5.5) can increase negative charges and then enhance the electrostatic repulsion of proteins. Generally, high pH results in a strong force. These net charges would provide many binding sites for water, and the electrostatic repulsion can resist the aggregation of myosin. This phenomenon may be an important reason for the increased solubility prior to thermal treatment. In addition to the effects of pH, salt can also contribute remarkably to the solubility of myosin. From the point of view of chemistry, proteins, as weak electrolytes, are difficult to be observed between anions and cations under the addition of NaCl (0.6 M, strong electrolyte). In a salting-in effect, Cl− ions can bind to the filaments of myosin and increase the electrostatic repulsive force (Offer & Trinick, Citation1983). Despite the significant relationship in value, the solubility of the unheated myosin at pH values of 7.0, 8.0, and 9.0 showed no substantial change, whereas the heated one varied evidently. Therefore, salt may play a major role when it is not heated. However, this occurrence cannot explain the large difference among solubilities at pH 7.0, 8.0, and 9.0 after heating. High temperature can increase the energy of the system and the probability of collision. Consequently, the salt effect may not be primarily dominated, which may be accounted to the pH, because of the repulsion induced by net charges. Hence, the thermal aggregation of myosin largely occurred in our experiments (75°C, 30 min). Many myosin monomer aggregates were formed; some of these aggregates were soluble, and others were insoluble. On the basis of this finding, we may speculate that high pH (high electrostatic repulsion, pH 9.0) can promote the formation of soluble aggregates. Thus, the remarkable solubility of myosin at pH 9.0 after heating may be due to the high content of soluble aggregates.
Figure 1. Solubility and turbidity of myosin in solution: (A) Solubility of myosin (5 mg/ml in 0.6 M NaCl PBS solutions) as related to pH with or without thermal treatment (75ºC water bath for 30 min). (B) Turbidity of myosin solution at λ=340 nm. Values are means ± SD (n >= 3), a-e indicates that the different letters are significantly different (P < 0.05).
Figura 1. Solubilidad y turbidez de la miosina en solución: (A) Solubilidad de la miosina (5mg/ml en soluciones de 0.6M NaCl PBS) en función del pH, con o sin tratamiento térmico (baño de agua a 75°C durante 30 min). (B). Turbidez de la solución de miosina a λ = 340 nm. Los valores son medias ± DE (n ≥ 3), a-e indican que las distintas letras son significativamente diferentes (P < 0.05).
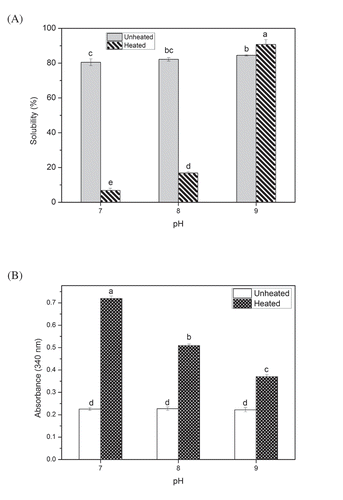
Similar to solubility, turbidity can provide a useful optical information for myosin aggregation. As depicted in , the absorbance values of unheated myosin were essentially unchanged, but those of the heated group were decreased from 0.72 to approximately 0.37 with increased pH. The low turbidity values of myosin in the unheated group showed no significant difference (p > 0.05) among one another, thereby suggesting the high solubility and low degree of aggregation of myosin. Nevertheless, a significant difference was observed among the turbidity values in the heated group. Evidently, the myosin aggregate formation was severely affected with prolonged water bath heating. Notably, this kind of aggregation was subject to pH limitation. Moreover, pH 9.0 showed the lowest turbidity. Aggregates occur only when the protein reaches a certain concentration (Ferrone, Citation1999). According to the point of view of molecular dynamics, water bath heating increased the effective concentration of the protein instantaneously, which induced the aggregate formation. However, the pH-induced electrostatic repulsion can impede the formation of this effective concentration (Militello et al., Citation2004). In addition, Vetri, Librizzi, Leone, and Militello (Citation2007) reported that the aggregation mechanism is considerably disordered when the electrostatic repulsion increases (i.e. when the pH of the solution is largely different from the pI value). Therefore, high pH (pH 9.0) makes the thermal aggregation of myosin complex and reduces the aggregation degree of myosin aggregates. Combined with solubility, the soluble aggregates were easily generated under high electrostatic repulsion.
3.1.2 Particle size distribution of myosin in solution
High-purity myosin is soluble in high-concentration salt solution (> 0.3 M) in the form of monomers and soluble oligomers (Shimada et al., Citation2015). Nevertheless, when myosin is heated, the monomers and the oligomers are denatured and aggregated into an insoluble complex gel (Puolanne & Halonen, Citation2010). The aggregation states of myosin molecules at different pH conditions (7.0, 8.0, and 9.0) before and after water bath heating were investigated by DLS. The particle size distribution for myosin in solution is expressed as volume percentage, and the corresponding results are presented in . One major peak at approximately 260 nm and a small peak exceeding 1 μm were observed for myosin at different pH conditions in all the unheated solution. Although assigning the myosin structure based on DLS results is not feasible, the first peak was assigned as the mixture of myosin monomers and oligomers, and another one was attributed to myosin filaments (Chen et al., Citation2016; Shimada et al., Citation2015). These results suggested that the myosin solution was not homogeneous. Additionally, the mixture can aggregate into filaments through myosin head–head interactions even at high salt concentration at room temperature. Notably, pH exerted no considerable effect on the particle size of myosin before heating, which was consistent with the results of solubility and turbidity analyses. Contrary to the unheated myosin solution, the distribution at different pH conditions (7.0, 8.0, and 9.0) after heating was relatively different. Three peaks were observed at pH values of 7.0 and 8.0. The major peak at approximately 260 nm was divided into two small peaks. This phenomenon was attributed to the thermal aggregation of myosin. The head–head interactions were largely increased at the expense of thermal energy. Furthermore, the particle size distribution became uneven due to the large clusters formed in the mixture. Remarkably, the peak exceeding 1 μm of the overall proportion increased considerably, which may suggest that a certain amount of filaments with large particle size was produced. According to (Shimada et al., Citation2015), this formed particles may be the soluble filaments and insoluble oligomer–filament complex. Given the low solubility at pH 7.0 and 8.0 after heating, the peak was the insoluble oligomer–filament complex. Two peak distributions favoring the unheated myosin were observed at pH 9.0, and the peak at approximately 260 nm still accounted for a large proportion. In addition, in the region between 350 nm and 1 μm, we found that the volume ratio gradually increased when pH increased to 9.0. Analysis showed that this increase may be attributed to the particle size of soluble aggregates. Nakasawa et al., (Citation2005) demonstrated that myosin aggregates are formed through rod–rod electrostatic interaction. With the increased pH, the electrostatic repulsion between myosin monomers is strengthened. When the repulsion is sufficient, it can disrupt the existing protein aggregates and prevent further aggregate formation (Teng, Luo, & Wang, Citation2012), even under prolonged heating. Therefore, soluble aggregates are products of prevented aggregation, which is induced by the electrostatic repulsion.
Figure 2. Particle size distribution of myosin (0.5 mg/ml in 0.6 M NaCl PBS solutions) as related to pH before and after heated (75ºC water bath for 30 min).
Figura 2. Distribución de los tamaños de partículas de miosina (0.5mg/ml en soluciones de 0.6M NaCl PBS) en función del pH antes y después del calentamiento (baño de agua a 75ºC durante 30 min).
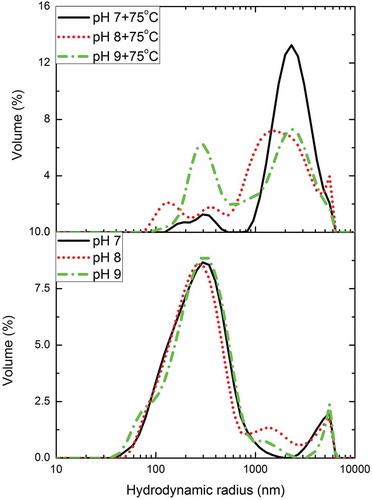
3.2 Effect of alkaline pH on the thermodynamic properties of myosin
Proteins in solution maintain a balance between its natural (folded) and denatured (unfolded) conformations; consequently, the conformation can affect the aggregation (Kristinsson & Hultin, Citation2003b; Ryan et al., Citation2013). This balance is closely related to the intramolecular energy of the protein. DSC can help determine the change in energy of protein molecules when heated. This technique is largely suitable for thermal aggregation study. The endotherm profile of myosin at different pH values (7.0, 8.0, and 9.0) in the NaCl PBS (0.6 M) is presented in . Evidently, two transitions peaks (Tm) were observed between 40°C and 60°C, which suggested that the myosin structure underwent two important transformations. The onset temperatures (Tonset) of denaturation of the three samples from pH 7.0 to 9.0 were 38.7°C, 38.4°C, and 38.4°C, respectively. A similar phenomenon was reported by Howell et al., (Citation1991); they indicated that increasing pH can create a condition favorable to myosin disaggregation in vitro. Moreover, the aggregated myosin (oligomers or filaments) showed higher stability in the initial time than that of monomers because additional calories were provided. Coincidentally, Zhao et al., (Citation2017) reported that protein aggregation may offset the endothermic effect of the heat-induced denaturation. In our experiments, heat-induced myosin denaturation was considerably affected with the aggregate state at the beginning of denaturation. With the pH increased from 7.0 to 9.0, the first major Tm displayed an increasing trend and occurred at 48.6°C, 49.1°C, and 49.3°C, respectively. The enthalpy change (∆H) of myosin at different pH values also showed the same trend as that of Tm (data not shown). This result indicated that a large amount of heat is required to denature myosin with increased pH. According to to Davies, Bardsley, Ledward, and Poulter (Citation1988), the different aggregation characteristics of myosin could partly explain the endothermic properties at different pH values. This trend is also observed in sarcoplasmic proteins (Tadpitchayangkoon, Park, & Yongsawatdigul, Citation2010); protein aggregation is assumed as highly dependent on pH and electrostatic repulsion, which reduces the protein–protein interaction and requires high temperature. Similar to the DLS analysis, given the prevented aggregation, many myosin monomers and oligomers cannot form the normal filaments and the oligomer–filament complex, which both exhibit high degree of aggregation. Consequently, many soluble myosin aggregates were produced at pH 9.0. Soluble aggregates in whey protein are precisely produced by heating under the condition that do not yield precipitates and large aggregates (Ryan & Foegeding, Citation2015). High-concentration salt in solution may weaken the electrostatic repulsion between proteins, especially with heating. Without discussion of the electrostatic repulsion, the difference in the DSC endotherms is probably related to the heads of myosin. In detail, many researchers revealed that the first peak corresponds to the denaturation of the myosin heads, and the second peak is the tail (Cortes-Ruiz et al., Citation2016). Kristinsson and Hultin (Citation2003a) demonstrated that the heads are sensitive to alkaline pH treatment. Alkaline pH may influence the structure of myosin heads. Thus, thermal aggregation through the interaction between the heads and the tail will be altered. According to the optical information, the soluble aggregate formation under pH 9.0 is probably due to the change in heads. Additionally, many oligomers and monomers cannot easily transform to the normal filaments and the oligomer–filament complex.
3.3 Effect of alkaline pH on the structure of myosin before and after thermal treatment
3.3.1 Changes in tertiary and quaternary structures
pH and heat can largely affect the tertiary and quaternary structures of protein molecules. The changes in surface hydrophobicity and R–SH content are used to characterize the tertiary and quaternary structure changes (Zhang et al., Citation2017). The changes in surface hydrophobicity and R–SH content of myosin are presented in . With the increased pH, the fluorescence intensity of myosin in the unheated group also increased (), whereas that of the heated group decreased. However, the fluorescence intensity of heated myosin was still considerably higher than that of the unheated one. Results indicated that increased pH can increase the surface hydrophobicity, and heating can increase such hydrophobicity more than that of increased pH. ANS is an extrinsic molecular probe that can noncovalently bind to the nonpolar region of the protein. Myosin heads are responsible for almost all of the ANS-binding sites (Kato & Konno, Citation1993). In addition, the myosin tail is not sensitive to alkaline treatment (Kristinsson & Hultin, Citation2003a). The positive relationship between ANS and pH suggested the unfolding of protein. Specifically, the myosin heads became remarkably open, and many hydrophobic groups from the interior of the protein were exposed. Evidently, prolonged heating substantially increased the surface hydrophobicity of myosin, but this phenomenon decreased with the increased pH after heating. The decreased surface hydrophobicity was in agreement with the results of Dong et al. (Citation2014). Nakai and Li-Chan (Citation1988) indicated that hydrophobic cluster exposure is a prerequisite for the heat-induced aggregation of myosin. Although a completely objective ANS can be affected with the pH, most researchers proved that values are higher at acidic pH than that in alkaline pH (Alizadeh-Pasdar & Li-Chan, Citation2000). Previous results also showed that high exposure of hydrophobic clusters may result in a distinct structure of myosin aggregates. With the aid of electrostatic repulsion and other acting forces, soluble aggregates largely existed at pH 9.0. In addition to this hypothesis, thiol oxidation can influence the aggregation of proteins and subsequent decline of surface hydrophobicity (Tadpitchayangkoon et al., Citation2010).
Figure 4. Changes in surface hydrophobicity (A) and reactive sulfhydryl content (B) of myosin (1 mg/ml in 0.6 M NaCl PBS solutions) with or without thermal treatment (75ºC water bath for 30 min) at different values of pH. Values are means ± SD (n >= 3), a-e indicates that the different letters are significantly different (P < 0.05).
Figura 4. Cambios en la hidrofobicidad superficial (A) y el contenido de sulfhidrilo reactivo (B) de miosina (1mg/ml en soluciones de 0.6M NaCl PBS) con o sin tratamiento térmico (baño de agua a 75ºC durante 30 min) con distintos valores de pH. Los valores son medias ± DE (n ≥ 3), a-e indican que las distintas letras son significativamente diferentes (P < 0.05).
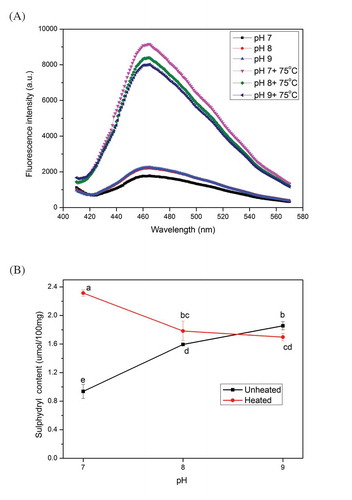
SH is an important chemical bond in proteins that plays an important role in the maintenance of the tertiary and quaternary structures of protein. Notably, SH can be easily oxidized to disulfide bonds, and most of SH is located in the heads of myosin (Xiong, Citation1997). The R–SH contents of myosin extracts from chicken breasts at different pH conditions are shown in . The R–SH content of myosin was the lowest at pH 7.0, and it increased with increased pH. Nonetheless, the R–SH content of the heated group presented a downward trend, and the content at pH 9.0 was lower than the unheated myosin at the same pH. Similar to the analysis of surface hydrophobicity, proteins unfolded at alkaline pH. Many interior groups, including hydrophobic and R–SH groups, were also exposed. Proteins at alkaline pH could aid the transformation between SH and inter- and intramolecular disulfide bonds (Tadpitchayangkoon et al., Citation2010). The increase or decrease of R–SH groups may be in the equilibrium of exposure and transformation. Therefore, heating can promote this process and aggravate the oxidation of SH. Heating denatured the proteins and modified the conformation structure with the following unmasking and activation of SH. Thus, the low R–SH content at pH 9.0 after heating may suggest the remarkable oxidation of R–SH. Frederiksen, Lund, Andersen, and Skibsted (Citation2008) demonstrated that the SH oxidation is related to the formation of myosin aggregates. Our DLS results showed that a large number of the oligomer–filament complex were formed. The heated myosin at pH 9.0 with the highest degree of oxidation displayed the lowest particle size. This result may be attributed to the intramolecular disulfide bonds of myosin that were formed (or at least more than that of the two other groups). Consequently, the subsequent thermal aggregation might be disturbed. As reported in whey proteins (Monahan, German, & Kinsella, Citation1995), when many intramolecular disulfide bonds are formed, the protein unfolding may be suppressed with the consequent decrease in SH content and surface hydrophobicity. Therefore, the intramolecular disulfide bonds may be an important factor contributing to the maximum amount of soluble myosin aggregates at pH 9.0. Specifically, alkaline pH affected the tertiary and quaternary structures of myosin heads and the following important head–head interaction, which may affect the formation of myosin aggregates.
3.3.2 Changes in secondary structure
The secondary structure of myosin was evaluated using far-UV CD spectroscopy. The effects of different pH conditions on myosin with or without thermal treatment are shown in . All the samples were similar to those reported previously (Chen et al., Citation2016; Deng, Luo, Wang, & Zhao, Citation2015), with two negative peaks at 208 and 222 nm. These two negative peaks are often regarded as the predominance of the α-helical structure of myosin (Chen et al., Citation2016; Guo et al., Citation2015). Evidently, the increase of pH in the unheated myosin exerted no apparent changes on the α-helical structure content; the contents were 73.1%, 72.8%, and 71.8%. However, when this myosin was subject to heating, the content decreased as follows: 59.0%, 63.5%, and 65.0%. The α-helical structure content in myosin is vital for observing the changes in secondary structure. According to Kristinsson and Hultin (Citation2003a), the α-helical structure of myosin is mostly distributed in the rod rather than in the heads. Our previous analysis also showed that the myosin rod is insensitive to the alkaline pH, which is possibly a major cause of the slight variation in the α-helical structure of the proteins before heating. Hence, heating is a common method to denature and unfold proteins. The evident decline in the α-helical structure of myosin after thermal treatment may be due to this heating and unfolding, thereby indicating the considerable thermal aggregation of myosin. Similar to the data on surface hydrophobicity and R–SH, the α-helical structure content before and after heating was diametrically opposed. On the view of molecular dynamics, such trend is unreasonable, and protein unfolding under high pH may be hindered. According to the analysis of the heat-induced soy protein gels (Puppo & Anon, Citation1998), disulfide bonds contribute largely to the stability of structure at alkaline pH. Similarly, Monahan et al. (Citation1995) reported that when many intramolecular disulfide bonds are formed in whey proteins, protein unfolding may be suppressed. On the basis of our results, we speculated that myosin at pH 9.0 may form intramolecular disulfide bonds in the head region, which can consequently inhibit the protein unfolding and change the subsequent thermal aggregation processes. Soluble myosin aggregates were formed in this environment.
Figure 5. Far-UV circular dichroism (CD) spectroscopy of myosin with or without thermal treatment (75ºC water bath for 30 min) as a function of pH. The concentration of myosin solutions was 0.2 mg/ml.
Figura 5. Espectroscopía de dicroísmo circular (CD) de UV lejano de miosina con o sin tratamiento termal (baño de agua a 75ºC durante 30 min) en función del pH. La concentración de las soluciones de miosina fue de 0.2mg/ml.
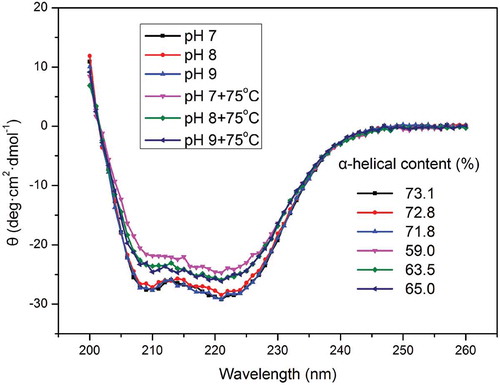
3.4 Initial stability testing of myosin after pH cycling
Chicken breast myosin treated at different pH conditions (7–9) after prolonged heating (75°C, 30 min) were acidized to pH 7.0, and their solubilities were investigated. The changes in solubility after pH cycling are shown in . The solubility of the sample treated with pH 9.0 after pH cycling (pH 9→7) was 90.9%. The solubility values of the control and pH 8→7 samples were only 6.7% and 12.4%, respectively. Evidently, these results were similar to those of solubility before the pH was adjusted to pH 7.0. The turbidity and particle size of myosin after pH cycling presented the same trend as the solubility (data not shown). This result indicated that the final pH adjustment to 7.0 exerted no considerable effect on the solubility and particle size of proteins. With prolonged heating and pH cycling, myosin still exhibited good stability, which proved the feasibility of soluble aggregate formation. Photographs from the 1st day were consistent with the results after pH cycling. Different solutions presented diverse color, and the control with deep white cloudy color indicated poor solubility. After 5 days, a large number of proteins settled at the bottom of the bottle. However, the solution at pH 9→7 still remained homogeneous, and only a little precipitation can be found. By contrast, the other solutions, including the untreated myosin solution, all displayed many deposits. These results indicated the excellent storage stability of the solution at pH 9→7. The proteins, which possessed high solubility and remarkable stability after thermal aggregation, were obtained. With this simple method, meat protein-based beverages can easily avoid thermal aggregation-induced insolubility, even under conventional thermal treatments. Considering the effect of heat treatment on the growth inhibition of microorganisms, the shelf life can also be guaranteed.
Figure 6. Solubility of myosin solutions with thermal treatment (75ºC water bath for 30 min) as related to pH-cycling. The photographs of the solutions at 1st day and 7th day were embedded in the figure. The bottle in the box is the untreated myosin solution, while the bottom three bottles are the solutions (from left, the control, 8→7 and 9→7) with thermal treatment after pH-cycling. Values are means ± SD (n >= 3), a-c indicates that the different letters are significantly different (P < 0.05).
Figura 6. Solubilidad de las soluciones de miosina con tratamiento térmico (baño de agua a 75°C durante 30 min) en función del ciclo de pH. Las fotografías de las soluciones tomadas en los días primero y séptimo fueron insertadas en la figura. La botella en la caja es la solución de miosina sin tratamiento, mientras que las tres botellas inferiores son las soluciones (desde la izquierda, el control, 8→7 y 9→7) con el tratamiento térmico posterior al ciclo de pH. Los valores son las medias ± DE (n ≥ 3), a-c indican que las distintas letras son significativamente diferentes (P < 0.05).
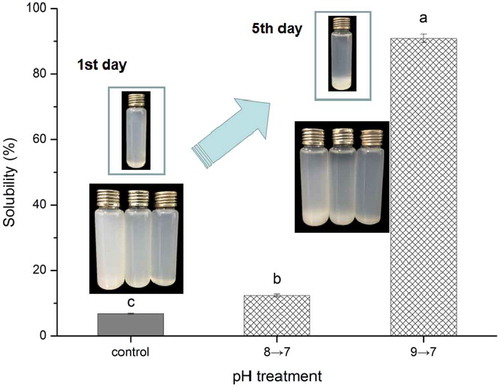
4. Conclusions
The thermal aggregation of myosin showed diverse response to different pH conditions (7.0, 8.0, and 9.0). In addition to particle size, the solubility and turbidity of myosin after thermal treatment were improved by adjusting the pH-dependent thermal aggregation. When pH increased to 9.0, myosin displayed remarkable thermal stability. We speculated that this phenomenon may be possibly caused by the formation of soluble aggregates. Moreover, myosin became considerably open, and many internal groups (hydrophobic and R–SH groups) were exposed. Nonetheless, although protein unfolding and group exposure were reinforced after prolonged heating, pH 9.0 showed the least reinforcement among the three groups. Soluble aggregates may be produced under such conditions, and electrostatic repulsion and intramolecular disulfide bonds may play important roles in this process. Our results strongly indicated that electrostatic repulsion reduced the aggregation degree of aggregates. Furthermore, the intramolecular disulfide bonds in the myosin head region essentially inhibited the formation of normal thermally aggregated complexes. The proteins after pH cycling also displayed high solubility and stability, which may be applicable for various applications.
Ethical Statement
This study was carried out in an accordance to animal welfare standards and were approved by the Ethical Committe for Animal Experiments of NanJing Agricultural University, China. All animal experiments accorded with the guidelines of the Animal Welfare Council of China
Disclosure statement
No potential conflict of interest was reported by the authors.
Additional information
Funding
References
- Alizadeh-Pasdar, N., & Li-Chan, E. C. Y. (2000). Comparison of protein surface hydrophobicity measured at various pH values using three different fluorescent probes. Journal Of Agricultural And Food Chemistry, 48(2), 328–334. Retrieved from <Go to ISI>://WOS:000085510000034.
- Anema, S. G., & Li, Y. M. (2003). Effect of pH on the association of denatured whey proteins with casein micelles in heated reconstituted skim milk. Journal Of Agricultural And Food Chemistry, 51(6), 1640–1646. Retrieved from <Go to ISI>://WOS:000181401400024.
- Chen, X., Tume, R. K., Xu, X. L., & Zhou, G. H. (2017). Solubilization of myofibrillar proteins in water or low ionic strength media: Classical techniques, basic principles, and novel functionalities. Critical Reviews in Food Science and Nutrition, 57(15), 3260–3280. Retrieved from <Go to ISI>://WOS:000401989100009.
- Chen, X., Zou, Y. F., Han, M. Y., Pan, L. H., Xing, T., Xu, X. L., & Zhou, G. H. (2016). Solubilisation of myosin in a solution of low ionic strength L-histidine: Significance of the imidazole ring. Food Chemistry, 196, 42–49. Retrieved from <Go to ISI>://WOS:000366223800006.
- Cortes-Ruiz, J. A., Pacheco-Aguilar, R., Ramirez-Suarez, J. C., Lugo-Sanchez, M. E., Garcia-Orozco, K. D., Sotelo-Mundo, R. R., & Pena-Ramos, A. (2016). . Conformational Changes in Proteins Recovered from Jumbo Squid (Dosidicus Gigas) Muscle through pH Shift Washing Treatments. Food Chemistry,196, 769–775. 10.1016/j.foodchem.2015.09.054 . Retrieved from <Go to ISI>://WOS:000366223800096.
- Creamer, L., Zoerb, H., Olson, N., & Richardson, T. (1982). Surface hydrophobicity of αs1-I, αs1-casein A and B and its implications in cheese structure. Journal of Dairy Science, 65(6), 902–906.
- Davies, J. R., Bardsley, R. G., Ledward, D. A., & Poulter, R. G. (1988). Myosin Thermal-Stability In Fish Muscle. Journal of the Science of Food and Agriculture, 45(1), 61–68. Retrieved from <Go to ISI>://WOS:A1988P665400007.
- Deng, Y., Luo, Y. L., Wang, Y. G., & Zhao, Y. Y. (2015). Effect of different drying methods on the myosin structure, amino acid composition, protein digestibility and volatile profile of squid fillets. Food Chemistry, 171, 168–176. Retrieved from <Go to ISI>://WOS:000343952300024.
- Dong, X. P., Ma, L. L., Zheng, J., Wang, J. T., Wu, Q., Song, S., & Zhou, D. Y. (2014). Effect of pH on the physicochemical and heat-induced gel properties of scallop Patinopecten yessoensis actomyosin. Fisheries Science, 80(5), 1073–1082. Retrieved from <Go to ISI>://WOS:000342220700024.
- Ferrone, F. (1999). Analysis of protein aggregation kinetics. Methods Enzymol, 309(1), 256–274. Retrieved from <Go to ISI>://WOS:000084721400017.
- Frederiksen, A. M., Lund, M. N., Andersen, M. L., & Skibsted, L. H. (2008). Oxidation of porcine myosin by hypervalent myoglobin: The role of thiol groups. Journal Of Agricultural And Food Chemistry, 56(9), 3297–3304. Retrieved from <Go to ISI>://WOS:000255655600060.
- Giroux, H. J., Houde, J., & Britten, M. (2010). Preparation of nanoparticles from denatured whey protein by pH-cycling treatment. Food Hydrocolloids, 24(4), 341–346. Retrieved from <Go to ISI>://WOS:000274082200012.
- Gornall, A. G., Bardawill, C. J., & David, M. M. (1949). Determination of serum proteins by means of the biuret reaction. Journal Biol Chemical, 177(2), 751–766.
- Guo, X., Peng, Z., Zhang, Y., Liu, B., & Cui, Y. (2015). The solubility and conformational characteristics of porcine myosin as affected by the presence of L-lysine and L-histidine. Food Chemistry, 170, 212–217.
- Hayakawa, T., Ito, T., Wakamatsu, J., Nishimura, T., & Hattori, A. (2009). Myosin is solubilized in a neutral and low ionic strength solution containing L-histidine. Meat Science, 82(2), 151–154. Retrieved from <Go to ISI>://WOS:000265398700002.
- Howell, B. K., Matthews, A. D., & Donnelly, A. P. (1991). Thermal-Stability Of Fish Myofibrils - a Differential Scanning Calorimetric Study. International Journal Of Food Science And Technology, 26(3), 283–295. Retrieved from <Go to ISI>://WOS:A1991FW11300005.
- Kato, S., & Konno, K. (1993). Isolation Of Carp Myosin Rod And Its Structural Stability. Nippon Suisan Gakkaishi, 59(3), 539–544. Retrieved from <Go to ISI>://WOS:A1993KX82400021.
- Kristinsson, H. G., & Hultin, H. O. (2003a). Changes in conformation and subunit assembly of cod myosin at low and high pH and after subsequent refolding. Journal Of Agricultural And Food Chemistry, 51(24), 7187–7196. Retrieved from <Go to ISI>://WOS:000186580100046.
- Kristinsson, H. G., & Hultin, H. O. (2003b). Effect of low and high pH treatment on the functional properties of cod muscle proteins. Journal Of Agricultural And Food Chemistry, 51(17), 5103–5110. Retrieved from <Go to ISI>://WOS:000184635500043.
- Mcswiney, M., Singh, H., & Campanella, O. H. (1994). Thermal Aggregation And Gelation Of Bovine Beta-Lactoglobulin. Food Hydrocolloids, 8(5), 441–453. Retrieved from <Go to ISI>://WOS:A1994PP73400004.
- Militello, V., Casarino, C., Emanuele, A., Giostra, A., Pullara, F., & Leone, M. (2004). Aggregation kinetics of bovine serum albumin studied by FTIR spectroscopy and light scattering. Biophysical Chemistry, 107(2), 175–187. Retrieved from <Go to ISI>://WOS:000189048000007.
- Monahan, F. J., German, J. B., & Kinsella, J. E. (1995). Effect of Ph and temperature on protein unfolding and thiol-disulfide interchange reactions during heat-induced gelation of whey proteins. Journal Of Agricultural And Food Chemistry, 43(1), 46–52. Retrieved from <Go to ISI>://WOS:A1995QD19900010.
- Nakai, S., & Li-Chan, E. (1988). Hydrophobicity-functionality relationship of food proteins. Hydrophobic interactions in food systems(pp. 43–61). Boca Raton: CRC Press.
- Nakasawa, T., Takahashi, M., Matsuzawa, F., Aikawa, S., Togashi, Y., Saitoh, T., & Yazawa, M. (2005). Critical regions for assembly of vertebrate nonmuscle myosin II. Biochemistry, 44(1), 174–183. Retrieved from <Go to ISI>://WOS:000226214600020.
- Offer, G., & Trinick, J. (1983). On the mechanism of water holding in meat: The swelling and shrinking of myofibrils. Meat Science, 8(4), 245–281.
- Pan, K., & Zhong, Q. X. (2016). Organic Nanoparticles in Foods: Fabrication, characterization, and utilization. Annual Review Of Food Science And Technology, 7(7), 245–266. Retrieved from <Go to ISI>://WOS:000371430700011.
- Pereira, P. M. D. C., & Vicente, A. F. D. B. (2013). Meat nutritional composition and nutritive role in the human diet. Meat Science, 93(3), 586–592. Retrieved from <Go to ISI>://WOS:000314735100032.
- Puolanne, E., & Halonen, M. (2010). Theoretical aspects of water-holding in meat. Meat Science, 86(1), 151–165.
- Puppo, M. C., & Anon, M. C. (1998). Structural properties of heat-induced soy protein gels as affected by ionic strength and pH. Journal Of Agricultural And Food Chemistry, 46(9), 3583–3589.
- Ryan, K. N., & Foegeding, E. A. (2015). Formation of soluble whey protein aggregates and their stability in beverages. Food Hydrocolloids, 43, 265–274. Retrieved from <Go to ISI>://WOS:000345683500033.
- Ryan, K. N., Zhong, Q., & Foegeding, E. A. (2013). Use of whey protein soluble aggregates for thermal stability—A hypothesis paper. Journal Of Food Science, 78(8). 10.1111/1750-3841.12027
- Shen, X., Fang, T. Q., Gao, F., & Guo, M. R. (2017). Effects of ultrasound treatment on physicochemical and emulsifying properties of whey proteins pre- and post-thermal aggregation. Food Hydrocolloids, 63, 668–676. Retrieved from <Go to ISI>://WOS:000389091600072.
- Shimada, M., Takai, E., Ejima, D., Arakawa, T., & Shiraki, K. (2015). Heat-induced formation of myosin oligomer-soluble filament complex in high-salt solution. International Journal Of Biological Macromolecules, 73, 17–22. Retrieved from <Go to ISI>://WOS:000349194300003.
- Tadpitchayangkoon, P., Park, J. W., & Yongsawatdigul, J. (2010). Conformational changes and dynamic rheological properties of fish sarcoplasmic proteins treated at various pHs. Food Chemistry, 121(4), 1046–1052. Retrieved from <Go to ISI>://WOS:000277107100019.
- Tazawa, T., Kato, S., Katoh, T., & Konno, K. (2002). Role of neck region in the thermal aggregation of myosin. Journal Of Agricultural And Food Chemistry, 50(1), 196–202. Retrieved from <Go to ISI>://WOS:000173078600035.
- Teng, Z., Luo, Y. C., & Wang, Q. (2012). Nanoparticles synthesized from soy protein: Preparation, characterization, and application for nutraceutical encapsulation. Journal Of Agricultural And Food Chemistry, 60(10), 2712–2720. Retrieved from <Go to ISI>://WOS:000301407000038.
- Vetri, V., Librizzi, F., Leone, M., & Militello, V. (2007). Thermal aggregation of bovine serum albumin at different pH: Comparison with human serum albumin. European Biophysics Journal with Biophysics Letters, 36(7), 717–725. Retrieved from <Go to ISI>://WOS:000248833500006.
- Xiong, Y. (1997). Structure-function relationships of muscle protein. In S. Damodaran & A. Paraf (Eds.), Food protein and their application (pp. 341–391). New York: Marcel Dekker, Inc.
- Yamamoto, K. Retrieved from<Go to ISI>://WOS:A1990EL63800002. (1990). Electron-microscopy of thermal aggregation of Myosin. Journal Of Biochemistry, 108(6), 896–898.
- Zhang, Z. Y., Yang, Y. L., Zhou, P., Zhang, X., & Wang, J. Y. (2017). Effects of high pressure modification on conformation and gelation properties of myofibrillar protein. Food Chemistry, 217, 678–686. Retrieved from <Go to ISI>://WOS:000384851800086.
- Zhao, X., Xing, T., Chen, X., Han, M.-Y., Xu, X.-L., & Zhou, G.-H. (2017). Yield, thermal denaturation, and microstructure of proteins isolated from pale, soft, exudative chicken breast meat by using isoelectric solubilization/precipitation. Process Biochemistry.