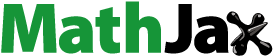
ABSTRACT
Catechin-loaded folate-conjugated chitosan nanoparticles (CFC-NPs) were synthesized through ionic gelation reactions between folate-modified chitosan and sodium tripolyphosphate. Their chemical structures, size distribution and morphology, drug loading capacity and entrapment efficiency, in vitro release behavior, and anti-proliferative effects were investigated. Results showed that CFC-NPs dispersed as a near-spherical shape with an average diameter of 255 nm. Drug loading capacity and entrapment efficiency reached 8.66–11.53% and 19.02–43.28%, respectively. Meanwhile, the loaded catechin exhibited a sustainable release from the nanoparticles, with the cumulative release ratio being 75.20% over 36 h. Blank folate-conjugated chitosan nanoparticles (FC-NPs) at 0.5 mg/mL had little or no cytotoxicity toward MCF-7 and HepG2 cells, while CFC-NPs had significant anti-proliferative activity in a dose-dependent manner. After incubation for 36 h, cell viability reached 35.22 ± 3.42% and 58.44 ± 3.85% in MCF-7 and HepG2 cells, respectively. These results suggest that CFC-NPs might be effective carrier of catechins with antitumor activity.
RESUMEN
En el presente estudio se sintetizaron nanopartículas de quitosano cargadas de catequina y conjugadas con folato (CFC-NPs) a través de reacciones de gelificación iónica entre el quitosano y el tripolifosfato de sodio modificados con folato. Se investigaron sus estructuras químicas, su distribución según el tamaño y la morfología, su capacidad de carga de droga y su eficiencia de atrapamiento, así como su comportamiento en la fase de liberación in vitro y sus efectos antiproliferativos. Los resultados mostraron que las CFC-NP se dispersan como una forma casi esférica cuyo diámetro promedio es de 255 mm. La capacidad de carga de droga y la eficiencia de atrapamiento alcanzaron 8.66-11.53% y 19.02-43.28%, respectivamente. Asimismo, la catequina cargada se liberó de las nanopartículas de forma sostenible, registrando una ratio de liberación cumulativa de 75.20% durante 36 h. Las nanopartículas de quitosano vacías, conjugadas con folato (FC-NPs) a 0.5 mg/mL, produjeron poca o ninguna citotoxicidad en las células MCF-7 y HepG2, mientras las CFC-NP exhibieron actividad antiproliferativa significativa dependiente de la dosis. Tras su incubación durante 36 h, la viabilidad celular alcanzó 35.22 ± 3.42% y 58.44 ± 3.85% en las células MCF-7 y HepG2, respectivamente. Los resultados apuntan a que las CFC-NP pueden ser efectivas portadoras de quitosano con actividad antitumoral.
PALABRAS CLAVE:
Introduction
Catechins are a group of polyphenolic compounds that are widely utilized as nutraceuticals for improving antioxidant, anti-inflammatory, and antibacterial activities (Hsieh et al., Citation2016; Quesille-villalobos, Torrico, & Ranilla, Citation2013). Studies on in vitro and animal experiments have supported the roles of catechins in inhibiting tumorigenesis in a variety of animal models of carcinogenesis, as well as in various human cell lines (Lambert & Yang, Citation2003). However, oral bioavailability of catechins is very low, and less than 5% of the molecules remain available following oral administration, due to low permeability and/or solubility within the gut, insufficient gastric residence time, and digestive instability (Zhang, Jung, & Zhao, Citation2016). These disadvantages greatly restricted the bioactive performance of the catechins and their applications as nutraceuticals and/or pharmaceuticals.
Nowadays, nanotechnology has manifested special advantages in various fields, including food processing and packaging, biomedicine, and pharmacy for improving product functionality (He & Hwang, Citation2016). Nanoparticles are believed to be promising candidates for the encapsulation of chemically labile bioactive substances to enhance their stability during food processing, and to improve the bioavailability of poorly soluble nutrients and drugs. It is reported that encapsulation of catechins in carbohydrates nanoparticles, liposomes significantly improved their antioxidant efficacy (Kosaraju, D’Ath, & Lawrence, Citation2006).
Chitosan, a polysaccharide derived by partial deacetylation of chitin, is widely used due to its versatile functionalities, including biodegradation and biocompatibility, non-toxicity, simple preparation, bacteriostatic, and anti-cholesteremic properties (Dutta, Tripathi, Mehrotra, & Dutta, Citation2009; Hernández-Ochoa, Macías-Castañeda, Nevárez-Moorillón, Salas-Muñoz, & Sandoval-Salas, Citation2012; Yuan, Zhang, Tang, & Sun, Citation2016). Moreover, chitosan is known to be mucoadhesive and transiently open the tight junctions between epithelial cells to enhance intestinal permeation of food nutrients and drugs (Thanou, Verhoef, & Junginger, Citation2001). Active hydroxyl and amine groups in the backbone of chitosan make it amenable to simple chemical modifications to form a large variety of chitosan-based nanoparticles, microspheres, hydrogels, and films. Recent studies demonstrated that the incorporation of targeted ligands with chitosan not only keep the original physicochemical properties of chitosan, but also endow tumor-targeted activity (Li et al., Citation2011). Of various ligands, folic acid is appealing for targeting cell membrane and allowing the nanoparticles endocytosis to deliver the attached therapeutic agents into the tumor sites. Folate receptor is significantly up-regulated on the surface of many cancer cells, such as ovarian, colorectal, colon, kidney, and breast cancers, whereas in most normal tissues, it is expressed in very low levels (Hilgenbrink & Low, Citation2005). The high affinity of folate to bind its receptor has facilitated the development of folate or folate conjugates-based targeted delivery systems (Ding et al., Citation2011). However, to the best of our knowledge, there appear to be limited investigations focusing on the properties of catechin-loaded folate-conjugated chitosan nanoparticles (CFC-NPs) and their cytotoxicity.
In this study, CFC-NPs were synthesized by ionic-gelation reactions. Their physicochemical properties including particle size, surface morphology, zeta potential, drug loading capacity, and in vitro release behavior were characterized. Moreover, their anti-proliferative effect was also evaluated by MTT assay using human breast adenocarcinoma MCF-7 cells and hepatocellular HepG2 cells.
Materials and methods
Materials
Chitosan (Mw = 178.19 kDa, deacetylation degree ≥95%, CS) and sodium tripolyphosphate (TPP) were purchased from Aladdin Reagent Company (Shanghai, China). Catechin (≥99%), folate acid, 1-(3-dimethylaminopropyl)-3-ethylcarbodiimide hydrochloride (EDC), and 3-(4,5-dimethylthiazol-2-yl)-2,5-diphenyl tetrazolium bromide (MTT) were obtained from Sigma-Aldrich Chemical Co. (St. Louis, MO, U.S.A.). Fetal bovine serum (FBS), folate-free Dulbecco’s modified Eagle’s medium (DMEM), trypsin-EDTA (0.25% trypsin with EDTA-4Na), and penicillin streptomycin were obtained from Thermo Fisher Scientific Inc. (Grand Island, NY, U.S.A.). All other chemicals were of analytical grade and purchased from Sinopharm Chemical Reagent Co., Ltd. (Shanghai, China).
Synthesis of folate-conjugated chitosan
Folate-conjugated chitosan was synthesized based on the mechanism of carbodiimide chemistry (Dubé, Francis, Leroux, & Winnik, Citation2002). Briefly, 2 g of CS was dissolved in 400 mL aqacetate buffer (pH 4.8) to form a 0.5% (m/v) CS solution. A mixture solution of EDC and folic acid was prepared by dissolving folic acid simultaneously in anhydrous dimethyl sulfoxide, and stirring at room temperature until folic acid was well dissolved. It was then added to the CS solution and stirred at room temperature in the dark for 16 h. The pH of the mixture was adjusted to 9.0 by dropwise addition of 1 M NaOH solution. The resulting coagulation was purified by dialysis against phosphate buffer (pH 7.4) for 3 days and then against for distilled water for another 3 days. Finally, the yellow colored folate-conjugated chitosan was collected and lyophilized at −50°C.
Preparation of CFC-NPs
CFC-NPs were prepared based on ionic gelation reactions as described previously (Calvo, Remuñán-López, Vila-Jato, & Alonso, Citation1997). Briefly, 40 mg of folate-conjugated chitosan was dissolved in 20 mL of 2% acetic acid solution (v/v), and TPP was dissolved in distilled water at a concentration of 2 mg/mL. A certain amount of catechin was added to the CS solution under magnetic stirring until it was well dissolved. Then, the mixture solution was brought to pH 5.0 by addition of aqueous NaOH. After that, 5 mL of TPP solution was added dropwise into 20 mL of CS and catechin mixture solution under magnetic stirring at room temperature, and was continuously stirred for 30 min. The formation of nanoparticles started spontaneously via the ionic gelation mechanism. The CFC-NPs were collected by centrifuged at 16,000 × g for 30 min and freeze-dried at −50°C for 24 h. Folate-conjugated chitosan nanoparticles without loading catechin (FC-NPs) were also prepared as a control.
Characterization of CFC-NPs
Fourier transform-infrared spectra (FT-IR) of catechin, folate-conjugated chitosan, and CFC-NPs were recorded by a FT-IR spectrometer (Vertex 70V, Bruker Company, Ettlingen, Germany) in the range of 600–4000 cm−1. The samples were diluted in KBr powder and pellets were made to perform the measurement.
The size distribution of nanoparticles was characterized by a 3000HS Particle Size Analyzer (Malvern Instruments Ltd., Malvern, U.K.) on the basis of dynamic light scattering (DLS) technique. Zeta potential was measured with a Nano ZS zeta potential analyzer (Malvern Instruments Ltd.).
The morphology analysis was performed by a transmission electron microscopy (TEM) (JEOL H-7650, Hitachi High-Technologies Corporation, Tokyo, Japan). A drop of nanoparticles dispersed in aqueous solutions was placed on a copper grid coated with carbon film and evaporated in air at room temperature.
Determination of the entrapment efficiency and loading capacity
The entrapment efficiency and loading capacity of CFC-NPs were determined as follows: CFC-NPs suspensions were centrifuged at 16,000 × g for 30 min. The free catechin in the clear supernatant was measured in triplicates as described previously (Mitsunaga, Doi, Kondo, & Abe, Citation1998; Sun, Ricardo-da-Silva, & Spranger, Citation1998) with some modifications. The total volume of reaction medium was fixed at 10 mL, comprising of 1 mL of the supernatant, 4.5 mL of 1% (w/v) vanillin (prepared freshly in methanol), and 4.5 mL of 9 M HCl solution. The mixture was vortexed for 1 min, and then allowed to react for 30 min at room temperature. Afterwards, its absorbance was recorded at 500 nm with a UV-2000 spectrophotometer (UNICO Shanghai Instruments Co., Ltd. Shanghai, China), and the amount of free catechin in the supernatant was calculated by comparison with a standard curve of catechin ranging from 2 to 12 μg/mL (, r2 = 0.9991). The entrapment efficiency and loading capacity were determined according to the following formula:
Where T is the total amount of catechin in the solution, F is the amount of free catechin in the supernatant after ultrafiltration, and W is the mass of CFC-NPs measured after freeze-drying.
In vitro release study
In vitro release behavior of catechin from CFC-NPs was assayed as per the method from Liang et al. (Citation2011). Ten milligrams of CFC-NPs were firstly suspended in 10 mL of phosphate buffer solution (pH 7.4), and incubated under shaking at 120 × g and 37°C. At predetermined time intervals, 2 mL medium was removed and replaced by 2 mL fresh PBS to maintain sink condition. The amount of catechin released into the solution was calculated. All measurements were performed in triplicate.
Assay of anti-proliferative effect
Human breast adenocarcinoma MCF-7 cells and hepatocellular carcinoma HepG2 cells were obtained from American Type Culture Collection (ATCC, Rockville, MD, U.S.A.). They were cultured in folate-free DMEM supplemented with 10% FBS, 100 U/mL penicillin, and 100 µg/mL of streptomycin in a humidified incubator at 37°C and 5% CO2.
Cytotoxicity of the nanoparticles was investigated by 3-(4,5-dimethylthiazol-2-yl)-2, 5-diphenyl tetrazolium bromide (MTT) assay as described previously (Mosmann, Citation1983). Briefly, cells were seeded in 96-well plates at a density of 1×105 cells/mL. After 24 h of preconditioning, the medium was replaced with fresh medium containing various concentrations of CFC-NPs (0.5 mg/mL, 0.25 mg/mL, and 0.125 mg/mL, respectively), and cells were incubated for a further 24 h or 36 h. At the end of the incubation period, the medium was decanted, and 100 μL of MTT dye solution (0.5 mg/mL in PBS buffer) were added to each well and the plate was incubated for 4 h at 37°C. Then, 200 μL of dimethyl sulfoxide were added and the whole was incubated for 15 min under gentle shaking at 37°C to dissolve/extract tetrazolium dye. Relative cell viability was calculated by determining the absorbance at 550 nm using a SPECTRAMAX M5e spectrophotometer (Molecular Devices Inc., Sunnyvale, CA, U.S.A.) and untreated control cells were assigned a relative viability of 100%.
Statistical analysis
All experiments were carried out in triplicate and data were expressed as means ± standard deviation. A one-way analysis of variance (ANOVA) was performed to calculate significant differences in treatment means, and multiple comparisons of means were done by the least significance difference test using the statistical software SAS 9.2 (SAS Institute Inc., Cary, NC, U.S.A.). A probability value of <0.05 was considered significant.
Results and discussion
FT-IR spectra analysis
The FT-IR spectra of catechin, folate-conjugated chitosan, and CFC-NPs are shown in . In previous studies, we had confirmed the successful synthesis of folate-conjugated chitosan by chemical linking of carboxylic group of folate with amino group of chitosan (Liu, Wang et al., Citation2014). Herein, we compared the changes of FT-IR peaks of CFC-NPs with those of folate-conjugated chitosan.
Figure 1. FT-IR spectra of (A) catechin, (B) folate-conjugated chitosan, and (C) CFC-NPs.
Figura 1. Espectro FT-IR de (A) catequina, (B) quitosano conjugado de folato y (C) CFC-NPs.s
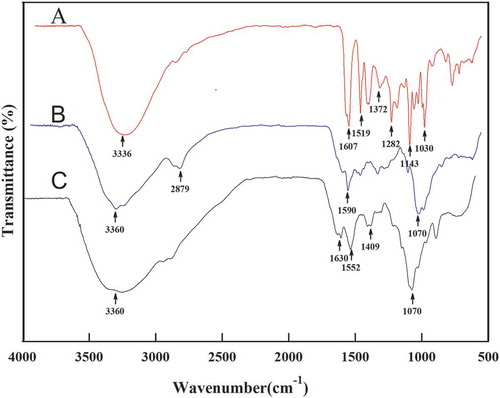
As shown in , catechin exhibited the typical characteristics of FT-IR spectrum of phenolic compounds. The broad absorption bands at 3336 cm−1 and 1372 cm−1 can be attributed to –OH stretching and plane blending vibrations, respectively. The bands within the 1400 cm−1 and 1600 cm−1 belong to C=C stretching vibrations of aromatic ring (Chen, Tsao, Liu, Huang, & Wang, Citation2010). The absorption bands within 1200 cm−1 and 1600 cm−1 are ascribed to C–O/C–C stretching vibrations (Liu, Lu, Kan, Wen, & Jin, Citation2014). The FT-IR spectra of folate-conjugated chitosan are illustrated in . The absorption peaks at 3360 cm−1 and the bands within 3065 cm−1 and 2480 cm−1 can be attributed to stretching vibrations of –NH and carboxyl association, respectively. The appearance of the absorption bands within 1604 cm−1 and 1484 cm−1 is attributed to the typical absorption of benzene ring. The absorption peak at 1590 cm−1, the specific peak of carbonyl groups, suggested the interaction between the carboxyl groups of the folic acid and the amine functionality of the chitosan molecule (Dudhani & Kosaraju, Citation2010). indicates that the spectrum of CFC-NPs is different from those of folate-conjugated chitosan. The peak of 3360 cm−1 became wider in CFC-NPs, indicating that hydrogen bonding is enhanced. The absorption peaks at 1590 cm−1 became stronger and shifted to 1552 cm−1, and a new sharp peak appeared at 1630 cm−1, suggesting the cross-linkage between ammonium ion of the folate-conjugated chitosan and phosphoric anions of sodium tripolyphosphate (Wu, Yang, Wang, Hu, & Fu, Citation2005). Moreover, as compared to catechin, the absorption at 1607 cm−1 disappeared, and the absorption peaks at 1630 cm−1 was strengthened due to the encapsulating of the catechin into the CFC-NPs. These results confirmed the successful formation of CFC-NPs by ionic gelation reactions between folate-modified chitosan and TPP.
Particle size, morphology, and zeta potential analysis
Particle size and zeta potentials are two important characteristics of the nanoparticles for allowing cell membrane interaction with nanoparticles and their endocytosis (Mansouri et al., Citation2006). Therefore, size distribution, zeta potentials, and surface morphology of the blank FC-NPs and CFC-NPs were measured by DLS and TEM, respectively. As shown in , the average diameters for FC-NPs and CFC-NPs were 105.7 nm ( and 255.0 nm (), respectively. Our results are in agreement with those reported by other authors, who found that the particle sizes of chitosan-tripolyphosphate nanoparticles ranged from 152 nm to 309.7 nm (Hu et al., Citation2008). The average diameter of the FC-NPs was obviously increased after loading of catechins in nanoparticles. Similar results are reported for the chitosan-lysozyme nanoparticles and for the kaempferol-loaded chitosan nanoparticles (Ilk, Sağlam, Özgen, & Korkusuz, Citation2017; Wu et al., Citation2017). The larger particle size of CFC-NPs might be attributed to the entrapment of catechin in the inner phase of the polysaccharide matrix. When drugs are present in the inner phase of chitosan-based nanoparticles, the particle size of these nanoparticles is usually larger than those drug-free nanoparticles (Du et al., Citation2005; Raj, Wairkar, Sridhar, & Gaud, Citation2018). Surface properties and morphology of the nanoparticles play a crucial role in drug release kinetics. TEM images demonstrate that both nanoparticles were dispersed as a near-spherical shape (), which was attributed to the expected electrostatic interaction based on oppositely charges of solutions (Souza et al., Citation2014). The mean particle size of CFC-NPs was in the range of 300–400 nm, which was obviously larger than that of the blank FC-NPs. In addition, it appeared that the sizes of the nanoparticles obtained by TEM were a little larger than those by particle size analyzer. These differences might be attributed to the different sample states of the nanoparticles as particle size analyzer measures the hydrodynamic radius of nanoparticles, while TEM visualizes the core of nanoparticles (Li et al., Citation2011). Moreover, for the TEM, the evaporation of water during the sample preparation might influence the dimensions of the nanoparticles (Zhu et al., Citation2011).
Figure 2. Particle size distribution of (A) FC-NPs and (B) CFC-NPs.
Figura 2. Distribución granulométrica de (A) FC-NPs y (B) CFC-NPs.
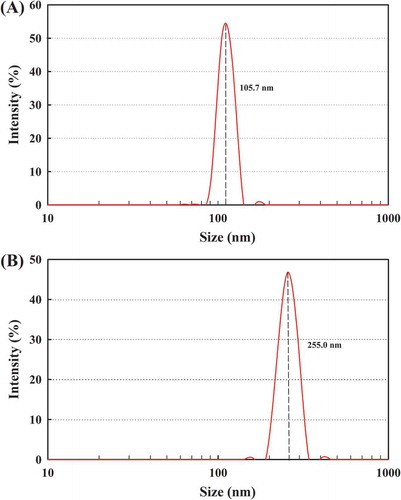
Figure 3. Transmission electron micrograph of (A) FC-NPs and (B) CFC-NPs.
Figura 3. Micrografía electrónica de transmisión de (A) FC-NPs y (B) CFC-NPs.
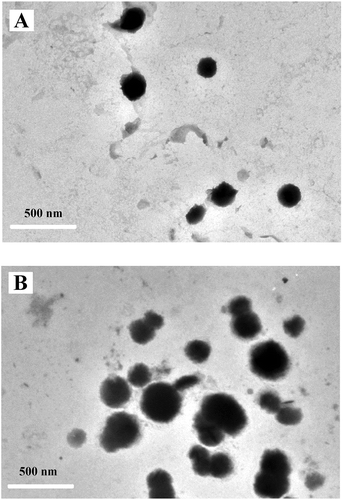
The zeta potential of FC-NPs and CFC-NPs was narrowly distributed with an average zeta potential of 17 mV and 24 mV, respectively. The formation of chitosan- tripolyphosphate nanoparticles is based on the interaction between the protonized–NH3+ in chitosan and the polyanionic phosphate groups in tripolyphosphate. Therefore, the surface charge of the nanoparticles depends on the degree of neutralization of –NH3+ by the polyanionic phosphate groups (Hu et al., Citation2008). The positive value of zeta potential is attributed to the cationic nature of chitosan. Besides, the positive surface charge might facilitate the nanoparticles mucoadhesive potential and absorption enhancement properties. In addition, the zeta potential of both nanoparticles was obviously lower when compared to that of the chitosan nanoparticles (approximately 30–50 mV), suggesting that the decoration of chitosan by folate molecule caused a decline in zeta potential. This change might be ascribed to the partial substitution of amino group in chitosan by folic acid in the coupling process, as well as the effects of side chain of folate on crosslinking between chitosan and TPP (Li et al., Citation2011). The zeta potential of the FC-NPs was obviously increased after loading of catechins in nanoparticles. Previous studies have shown that covalent bonding and hydrogen bonding between tea catechins and chitosan occurred during the formation of nanoparticles loaded with tea catechins (Hu et al., Citation2008). This interaction between catechins and chitosan might make catechin not only entrapped within the nanoparticle matrix but also embedded on the exterior, which might be responsible for the variation of zeta potentials of catechin-loaded chitosan nanoparticles.
Entrapment efficiency and loading capacity
The entrapment efficiency and loading capacity of CFC-NPs with different mass ratios of catechin, folate-conjugated chitosan, and TPP are shown in . When the mass ratio was 3:15:5 (Formulation I), the loading capacity of CFC-NPs was 8.66 ± 1.02%, which was slightly lower than those comprised of a mass ratio of 9:15:5 (formulation II, 11.53 ± 0.62%) (p < 0.05). By contrast, the entrapment efficiency was reduced with the increase of catechin in CFC-NPs. When the mass ratio was varied from 3:15:5 to 9:15:5, the entrapment efficiency of the CFC-NPs was significantly decreased from 43.28 ± 1.02% to 19.02 ± 0.62% (p < 0.01), suggesting that the excess of catechin could not be totally entrapped into the nanoparticles. Similar result was observed in the tea polyphenols-loaded chitosan nanoparticles (Liang et al., Citation2011). The decreased entrapment efficiency for Formulation II might be explained that the additive amount of catechin might exceed the capacity of the folate-conjugated chitosan nanoparticles to encapsulate the compound. In addition, some researchers have confirmed that there are interactions of polyphenolic with the chitosan matrix (Kosaraju et al., Citation2006). Similar interactive actions were also found between hydroxyl groups in catechin and amide group in chitosan (Zhang & Kosaraju, Citation2007). It is possible that the interaction between excessive catechin and chitosan matrix might be unfavorable for the formation of chitosan-tripolyphosphate nanoparticles, leading to a decrease in entrapment efficiency of catechin.
Table 1. The entrapment efficiency and loading capacity of CFC-NPs with different mass ratios of catechin, folate-conjugated chitosan, and TPP.
Tabla 1. Eficiencia de atrapamiento y capacidad de carga de CFC-NPs con ratios de masa diferentes de catequina, quitosano conjugado de folato y TPP.
In vitro release of catechins from CFC-NPs
In vitro release behavior of catechins from CFC-NPs was investigated in PBS (pH 7.4) at 37°C. The cumulative release profile of CFC-NPs with different mass ratios (3:15:5 and 9:15:5) is shown in . The loaded catechins exhibited a sustainable release from the CFC-NPs, and the cumulative release ratio reached 75.2% (9:15:5) and 59.4% (3:15:5) over 36 h, respectively. The release profile was divided into two different phases. An apparent initial burst was observed within the first 4 h, in which about 52.8% (9:15:5) and 43.3% (3:15:5) of the catechins was released from CFC-NPs. Thereafter, the drug release profiles switched to a slow release phase, where the loaded catechins were released from the CFC-NPs in a continuous way for up to 36 h. The initial burst effect might result from the catechin absorbed on the shell of the nanoparticles. The slow release might be explained that the chitosan nanoparticles had a rigid and hydrophobic core, restricting the migration of the catechin from the core (Tan & Liu, Citation2009). It is noteworthy that CFC-NPs (9:15:5) had a greater cumulative release ratio than those (3:15:5) throughout the experiment. It could be explained considering that the nanoparticles (3:15:5) had a relative higher polymer concentration, leading to form the thicker polymer wall, and thus prevented the catechin from releasing. Regardless of formulation, the CFC-NPs exhibited the potential to release the loaded catechin sustainably.
Anti-proliferative effect of CFC-NPs
Anti-proliferative effect of the nanoparticles was evaluated by MTT assay, which is a colorimetric test based on the selective ability of viable cells to reduce the tetrazolium component of MTT into purple formazan crystals (Berridge & Tan, Citation1993). Human breast adenocarcinoma MCF-7 cells (folate receptor-positive) and hepatocellular carcinoma HepG2 cells (folate receptor-negative) were used (Duan et al., Citation2012).
After incubation with the different concentrations of CFC-NPs or FC-NPs for 24 and 36 h, the cell viability is shown in . As for both cells, nanoparticles exhibited varied cell cytotoxicity depending on the incubated concentration and time. There were no significant decreases in cell viability in both cells when incubated with FC-NPs for 24 or 36 h even at a concentration of 0.5 mg/mL, suggesting that the FC-NPs had little or no cytotoxicity. The proof of weak or no cytotoxicity of chitosan-based micelles or nanoparticles were also reported elsewhere (Cao et al., Citation2007). For MCF-7 cells, the CFC-NPs showed significant anti-proliferative effects in a concentration-dependent fashion (p < 0.05). After incubation with CFC-NPs (0.5 mg/mL) for 24 and 36 h, the cell viability was decreased to 41.36 ± 1.58% and 35.22 ± 3.42%, respectively. On the contrary, on the HepG2 cells which were folate receptor-negative, CFC-NPs exhibited the weaker cell cytotoxic activities compared with that on MCF-7 cells. After incubation for 24 and 36 h, the lowest cell viabilities were 66.11 ± 3.29% and 58.44 ± 3.85%, respectively. The cell viability of MCF-7 cells was approximately two times lower than HepG2 cells, suggesting that the CFC-NPs have stronger cytotoxic effects on folate receptor-positive tumor cells. These results indicated that the folate moieties in CFC-NPs played an important role in enhancing cytotoxic effect by binding of CFC-NPs with folate receptors on MCF-7 cells, and subsequently improving their intracellular uptake through the receptor-mediated endocytosis. Our results are in agreement with Duan et al. (Citation2012), who found that incorporation of folate with chitosan/doxorubicin poly(butyl cyanoacrylate) nanoparticles resulted in enhanced cellular uptake and cytotoxicity against MCF-7 cells. Folate-tethered emulsions of all-trans retinoic acid were capable of improving growth inhibitory effects against human mouth epithelial carcinoma KB cells than those without folate conjugation (Kim et al., Citation2008). In addition, we also observed that the anti-proliferative effects of the nanoparticles were evaluated in both cells when incubation time prolonged, which might be explained considering that more catechin was released from CFC-NPs with incubation time. Similar dose- and time-dependent cytotoxicity were reported for N-succinyl-chitosan nanoparticles in human chronic myeloid leukemia K562 cells (Luo, Su, Wang, Wang, & Li, Citation2012), as well as for chitosan-loaded tea polyphenols nanoparticles in human HepG2 cells (Liang et al., Citation2011).
Conclusion
Taken together, CFC-NPs were synthesized in this study through ionic gelation reactions between folate-modified chitosan and TPP. Characterization using FT-IR confirmed the cross-linkage between ammonium ion of the chitosan and phosphoric anions of TPP, as well as the encapsulation of the catechin into the CFC-NPs. The morphology of CFC-NPs was a near-spherical shape with an average diameter of 255 nm. Drug loading capacity and release results showed that the catechin had a high loading content in nanoparticles, and a sustainable release profile. In addition, the FC-NPs had little or no cytotoxicity even at the tested concentration of 1 mg/mL toward MCF-7 and HepG2 cells, while CFC-NPs exhibited significant anti-proliferative effects in a dose-dependent manner. These results suggest that CFC-NPs can become a promising targeted delivery system of catechin. More efforts should be made in the future to assess the anti-proliferative effect of CFC-NPs using different cancer cell lines as well as their performance in vivo.
Abbreviations
CS: chitosan; TPP: sodium tripolyphosphate; EDC: 1-(3-dimethylaminopropyl)-3-ethylcarbodiimide hydrochloride; MTT: 3-(4,5-dimethylthiazol-2-yl)-2,5-diphenyl tetrazolium bromide; CFC-NPs: catechin-loaded folate-conjugated chitosan nanoparticles; FC-NPs: folate-conjugated chitosan nanoparticles; FT-IR: Fourier transform-infrared spectra; DLS dynamic light scattering; TEM: transmission electron microscopy.
Disclosure statement
No potential conflict of interest was reported by the authors.
Additional information
Funding
References
- Berridge, M. V., & Tan, A. S. (1993). Characterization of the cellular reduction of 3-(4,5-dimethylthiazol-2-yl)-2,5-diphenyltetrazolium bromide (MTT): Subcellular localization, substrate dependence, and involvement of mitochondrial electron transport in MTT reduction. Archives of Biochemistry and Biophysics, 303, 474–482.
- Calvo, P., Remuñán-López, C., Vila-Jato, J. L., & Alonso, M. J. (1997). Novel hydrophilic chitosan-polyethylene oxide nanoparticles as protein carriers. Journal of Applied Polymer Science, 63, 125–132.
- Cao, Y., Zhang, C., Shen, W., Cheng, Z., Yu, L., & Ping, Q. (2007). Poly(N-isopropylacrylamide)–Chitosan as thermosensitive in situ gel-forming system for ocular drug delivery. Journal of Controlled Release, 120, 186–194.
- Chen, Y. M., Tsao, T. M., Liu, C. C., Huang, P. M., & Wang, M. K. (2010). Polymerization of catechin catalyzed by Mn-, Fe- and Al-oxides. Colloids and Surfaces B: Biointerfaces, 81, 217–223.
- Ding, N., Lu, Y., Lee, R. J., Yang, C., Huang, L., Liu, J., & Xiang, G. (2011). Folate receptor-targeted fluorescent paramagnetic bimodal liposomes for tumor imaging. International Journal of Nanomedicine, 6, 2513–2520.
- Du, J., Zhang, S., Sun, R., Zhang, L., Xiong, C., & Peng, Y. (2005). Novel polyelectrolyte carboxymethyl konjac glucomannan-chitosan for drug delivery. II. Release of albumin in vitro. Journal of Biomedical Materials Research Part B: Applied Biomaterials, 72, 299–304.
- Duan, J., Liu, M., Zhang, Y., Zhao, J., Pan, Y., & Yang, X. (2012). Folate-decorated chitosan/doxorubicin poly(butyl)cyanoacrylate nanoparticles for tumor-targeted drug delivery. Journal of Nanoparticle Research, 14, 1–9.
- Dubé, D., Francis, M., Leroux, J. C., & Winnik, F. M. (2002). Preparation and tumor cell uptake of poly(N-isopropylacrylamide) folate conjugates. Bioconjugate Chemistry, 13, 685–692.
- Dudhani, A. R., & Kosaraju, S. L. (2010). Bioadhesive chitosan nanoparticles: Preparation and characterization. Carbohydrate Polymers, 81, 243–251.
- Dutta, P. K., Tripathi, S., Mehrotra, G. K., & Dutta, J. (2009). Perspectives for chitosan based antimicrobial films in food applications. Food Chemistry, 114, 1173–1182.
- He, X., & Hwang, H. M. (2016). Nanotechnology in food science: Functionality, applicability, and safety assessment. Journal of Food and Drug Analysis, 24, 671–681.
- Hernández-Ochoa, L., Macías-Castañeda, C. A., Nevárez-Moorillón, G. V., Salas-Muñoz, E., & Sandoval-Salas, F. (2012). Antimicrobial activity of chitosan-based films including spices’ essential oils and functional extracts. CyTA - Journal of Food, 10, 85–91.
- Hilgenbrink, A. R., & Low, P. S. (2005). Folate receptor-mediated drug targeting: From therapeutics to diagnostics. Journal of Pharmaceutical Sciences, 94, 2135–2146.
- Hsieh, S. K., Xu, J. R., Lin, N. H., Li, Y. C., Chen, G. H., Kuo, P. C., … Tzen, J. T. C. (2016). Antibacterial and laxative activities of strictinin isolated from Pu’er tea (Camellia sinensis). Journal of Food and Drug Analysis, 24, 722–729.
- Hu, B., Pan, C., Sun, Y., Hou, Z., Ye, H., Hu, B., … Zeng, X. (2008). Optimization of fabrication parameters to produce chitosan-tripolyphosphate nanoparticles for delivery of tea catechins. Journal of Agricultural & Food Chemistry, 56, 7451–7458.
- Ilk, S., Sağlam, N., Özgen, M., & Korkusuz, F. (2017). Chitosan nanoparticles enhance the anti-quorum sensing activity of kaempferol. International Journal of Biological Macromolecules, 94, 653–662.
- Kim, S. H., Kim, J. K., Lim, S. J., Park, J. S., Lee, M. K., & Kim, C. K. (2008). Folate-tethered emulsion for the target delivery of retinoids to cancer cells. European Journal of Pharmaceutics and Biopharmaceutics, 68, 618–625.
- Kosaraju, S. L., D’Ath, L., & Lawrence, A. (2006). Preparation and characterisation of chitosan microspheres for antioxidant delivery. Carbohydrate Polymers, 64, 163–167.
- Lambert, J. D., & Yang, C. S. (2003). Cancer chemopreventive activity and bioavailability of tea and tea polyphenols. Mutation Research/Fundamental and Molecular Mechanisms of Mutagenesis, 523-524, 201–208.
- Li, P., Wang, Y., Zeng, F., Chen, L., Peng, Z., & Kong, L. X. (2011). Synthesis and characterization of folate conjugated chitosan and cellular uptake of its nanoparticles in HT-29 cells. Carbohydrate Research, 346, 801–806.
- Liang, J., Li, F., Fang, Y., Yang, W., An, X., Zhao, L., … Hu, Q. (2011). Synthesis, characterization and cytotoxicity studies of chitosan-coated tea polyphenols nanoparticles. Colloids and Surfaces B: Biointerfaces, 82, 297–301.
- Liu, B., Wang, Y., Yu, Q., Liu, F., Zang, J., Jin, L., … Li, F. (2014). Optimization of preparation of catechin-loaded folate-conjugated chitosan nanoparticles by response surface analysis. Food Science, 35, 46–52.
- Liu, J., Lu, J. F., Kan, J., Wen, X. Y., & Jin, C. H. (2014). Synthesis, characterization and in vitro anti-diabetic activity of catechin grafted inulin. International Journal of Biological Macromolecules, 64, 76–83.
- Luo, H., Su, H., Wang, X., Wang, L., & Li, J. (2012). N-Succinyl-chitosan nanoparticles induced mitochondria-dependent apoptosis in K562. Molecular and Cellular Probes, 26, 164–169.
- Mansouri, S., Cuie, Y., Winnik, F., Shi, Q., Lavigne, P., Benderdour, M., … Fernandes, J. C. (2006). Characterization of folate-chitosan-DNA nanoparticles for gene therapy. Biomaterials, 27, 2060–2065.
- Mitsunaga, T., Doi, T., Kondo, Y., & Abe, I. (1998). Color development of proanthocyanidins in vanillin-hydrochloric acid reaction. Journal of Wood Science, 44, 125–130.
- Mosmann, T. (1983). Rapid colorimetric assay for cellular growth and survival: Application to proliferation and cytotoxicity assays. Journal of Immunological Methods, 65, 55–63.
- Quesille-villalobos, A. M., Torrico, J. S., & Ranilla, L. G. (2013). Phenolic compounds, antioxidant capacity, and in vitro α-amylase inhibitory potential of tea infusions (Camellia sinensis) commercialized in Chile. CyTA - Journal of Food, 11, 60–67.
- Raj, R., Wairkar, S., Sridhar, V., & Gaud, R. (2018). Pramipexole dihydrochloride loaded chitosan nanoparticles for nose to brain delivery: Development, characterization and in vivo anti-Parkinson activity. International Journal of Biological Macromolecules, 109, 27–35.
- Souza, M. P., Vaz, A. F. M., Correia, M. T. S., Cerqueira, M. A., Vicente, A. A., & Carneiro-da-Cunha, M. G. (2014). Quercetin-loaded lecithin/chitosan nanoparticles for functional food applications. Food and Bioprocess Technology, 7, 1149–1159.
- Sun, B., Ricardo-da-Silva, J. M., & Spranger, I. (1998). Critical factors of vanillin assay for catechins and proanthocyanidins. Journal of Agricultural and Food Chemistry, 46, 4267–4274.
- Tan, Y. L., & Liu, C. G. (2009). Self-aggregated nanoparticles from linoleic acid modified carboxymethyl chitosan: Synthesis, characterization and application in vitro. Colloids and Surfaces B: Biointerfaces, 69, 178–182.
- Thanou, M., Verhoef, J. C., & Junginger, H. E. (2001). Oral drug absorption enhancement by chitosan and its derivatives. Advanced Drug Delivery Reviews, 52, 117–126.
- Wu, T., Wu, C., Fu, S., Wang, L., Yuan, C., Chen, S., … Hu, Y. (2017). Integration of lysozyme into chitosan nanoparticles for improving antibacterial activity. Carbohydrate Polymers, 155, 192–200.
- Wu, Y., Yang, W., Wang, C., Hu, J., & Fu, S. (2005). Chitosan nanoparticles as a novel delivery system for ammonium glycyrrhizinate. International Journal of Pharmaceutics, 295, 235–245.
- Yuan, G., Zhang, X., Tang, W., & Sun, H. (2016). Effect of chitosan coating combined with green tea extract on the melanosis and quality of Pacific white shrimp during storage in ice. CyTA - Journal of Food, 14, 35–40.
- Zhang, H., Jung, J., & Zhao, Y. (2016). Preparation, characterization and evaluation of antibacterial activity of catechins and catechins-Zn complex loaded β-chitosan nanoparticles of different particle sizes. Carbohydrate Polymers, 137, 82–91.
- Zhang, L., & Kosaraju, S. L. (2007). Biopolymeric delivery system for controlled release of polyphenolic antioxidants. European Polymer Journal, 43, 2956–2966.
- Zhu, H., Liu, F., Guo, J., Xue, J., Qian, Z., & Gu, Y. (2011). Folate-modified chitosan micelles with enhanced tumor targeting evaluated by near infrared imaging system. Carbohydrate Polymers, 86, 1118–1129.