ABSTRACT
The effects of lipid peroxidation on rice bran protein (RBP) structure was examined through Raman Spectroscopy. 2,2’-azobis (2-amidinopropane) dihydrochloride (AAPH), acrolein, and malondialdehyde (MDA) are chosen for lipid peroxidation. Incubation of RBP with the increasing concentration of three oxidants resulted in a gradual generation of protein carbonyl derivatives. It can be deduced from the Raman spectra that a low concentration of AAPH and acrolein both decreased the percentage of α-helix and β-sheet, which increased with MDA concentration. The Raman intensities of tryptophan residues of oxidized RBP by AAPH and acrolein, both initially increased and then decreased with oxidation concentration. After MDA treatment, the Raman intensities corresponding to Trp residues and Tyr doublet ratio of RBP were increased and then decreased, and decreased and then increased with oxidation concentration, respectively. The structural change induced by RBP oxidation with the Raman Spectroscopy can aid in refining the function of RBP characteristics and control the adjustment of the storage condition.
RESUMEN
Este estudio se propuso investigar los efectos de la peroxidación lipídica en la estructura de la proteína del salvado de arroz (RBP) empleando espectroscopia Raman. Para provocar la peroxidación lipídica se eligieron el dihidrocloruro de 2,2’-azobis (2-amidinopropano) (AAPH), la acroleína y el malondialdehído (MDA). La incubación de la RBP con una concentración creciente de los tres oxidantes dio lugar a la generación gradual de derivados carbonílicos de las proteínas. Los espectros Raman obtenidos permiten inferior que una baja concentración de AAPH y de acroleína disminuyó el porcentaje de α-hélice y de β-hoja, que, en cambio, aumentó con la concentración de MDA. Las intensidades Raman de los residuos de triptófano de la RBP oxidada por el AAPH y la acroleína aumentaron inicialmente, para luego disminuir con la concentración de oxidación. Tras el tratamiento con MDA, las intensidades Raman correspondientes a los residuos de Trp aumentaron y posteriormente se redujeron, mientras que la ratio de dobletes de Tyr de la RBP disminuyó, para después aumentar con la concentración de oxidación. El cambio estructural inducido por la oxidación de la RBP y detectado con la espectroscopia Raman puede ayudar a precisar la función de las características de la RBP y controlar el ajuste de las condiciones de almacenamiento.
1. Introduction
As one of the major products of the milling industry, rice bran is rich in protein (11.4–14.7%), carbohydrates (34.7-61-5%), and oil (15.6–19.5%). Previous studies have indicated that rice bran protein (RBP) has high threonine and lysine amounts (Fabian & Ju, Citation2011; Mawal et al., Citation1987; S. Tang et al., Citation2003). This protein has been used in preparing infant formula and has shown cancer-suppressing properties (Shoji et al., Citation2001). The amino acid composition of RBP has been a nutritious dietary source for children (Venlet et al., Citation2021).
Recenlty, researchers focused on rice bran protein stabilization (Mousavi et al., Citation2020; Rafe & Sadeghian, Citation2017; Rafe et al., Citation2017). However, the high activity of fatty lipohydrolase and lipoxidase of rice bran can hydrolyze lipids and produce free fatty acids, leading to rice bran’s rancidity. The free fatty acid can easily be oxidized and form lipid free radicals and active lipid products, which induces the oxidation of RBP and cause serious effects on the structure and function of RBP. Reactive oxygen species (ROS) and other oxidation products contribute directly and indirectly to RBP oxidation (Shacter, Citation2000). ROS can damage target proteins which lower their essential amino acid content and structural conformations (Chang et al., Citation2020). In addition, ROS significantly lowers protein digestibility and rheological properties (Yan et al., Citation2022). However, little is known of the correlation between oxidative activities and their effects on RBP structure and function.
It has been established previously that lipid peroxidation (LPO) and protein oxidation (PO) are closely related (Santillán Deiú et al., Citation2021). The LPO produces smaller-unit reactive aldehydes and ketones using lipid hydroperoxides as the starting material (Tironi et al., Citation2006). These final products can modify protein structure (Refsgaard et al., Citation2000). PO and LPO have several products: peroxyl radicals (Gieseg et al., Citation2000); therefore, we aim to assess how it influences lipoxygenase – catalyzed lipid and protein oxidations during processing and storage. As a water-soluble radical, 2,2’-azobis (2-amidinopropane) dihydrochloride (AAPH) can be thermally decomposed to peroxyl radicals under aerobic settings (Gieseg et al., Citation2000). It is unclear how reactive aldehydes and lipid hydroperoxides influence RBP oxidation, even though aldehydes are the building blocks of lipid hydroperoxides. LPO-derived reactive aldehydes are generally stable and convey oxidant function to selected target sites. They play key regulatory roles due to their longevity and high reactivity (Carbone et al., Citation2005). Our study used three similar compounds to LPO-derived aldehydes (AAPH, Acrolein, and MDA) to investigate how reactive aldehydes modify RBP. In this respect, the usefulness of the Raman spectroscopy for the study of protein structural changes in food in situ during processing and storage has been shown, together with the possibilities of using protein structural changes, to predict protein functional properties and sensory attributes (Herrero, Citation2008). Therefore, the aim of this research was to investigate RBP structural changes under different methods of oxidative modification by using Raman spectroscopy. In this study, the effects of three major oxidation processes on the protein structure and function of rice bran were systematically analyzed through the oxidative induction of AAPH, acrolein and MDA, providing theoretical guidance for the oxidative regulation of rice bran processing and storage, and new ideas for the research and development of RBP products, and promote the development of rice bran protein industry.
2. Methodology
2.1. Samples and materials
Rice bran samples without fat were procured from Dong Fang Group (Harbin, China). Sigma – Aldrich (St. Louis, MO, USA) supplied the acrolein, 2,2’-azobis-(2-methylpropionamidine) dihydrochloride (AAPH), and 1,1,3,3-tetramethoxypropane used in this research. Moreover, all chemicals and reagents were of analytical grade and purchased from Sinopharm Chemical Reagent Co. LTD.
2.2. Preparation of RBP
The procured rice bran samples were prepared L. Zhou et al. (Citation2016) described earlier. The pH was first adjusted to 9.5 using 0.1 M of NaOH to solubilize the protein in the rice bran samples and shaking at 300 rpm for 2.0 h at 50°C. The supernatant obtained by centrifugation at 3000 × g for about 20 min was then precipitated by adjusting the pH to 3.8 with 0.1 M of HCl and centrifuged at 3000 × g for about 20 min. The precipitated protein was centrifuged and washed with distilled water two times, and the pH was brought up to 7.0 before freeze-drying, gives the RBP (87.63% protein content).
2.3. Oxidation of RBP by AAPH
The method of was followed in carrying out this step. Briefly, RBP suspensions were prepared as previously described and mixed with a serial concentration of AAPH and then incubated in a constantly stirred water bath at 37°C under dark conditions for 1 d. The final concentrations of AAPH in RBP suspension is 0, 0.04, 0.2, 1, 5, and 25 mM, respectively. After cooling to 4°C in an ice bath and the reaction halted, samples were centrifuged. The supernatant is dialyzed against deionized water at 4°C for 3 d to remove residual AAPH and salt. The obtained solutions were kept at 4°C before use.
2.4. Oxidation of RBP by acrolein
Here, the method of Wu et al. (Citation2010) was followed. Briefly, different acrolein concentrations were mixed with RBP samples and then stored at room temperature under dark conditions for 1 d. The final concentration of acrolein is 0 (control), 0.01, 0.1, 1, and 10 mM, respectively. Afterwards, the protein solution temperature was adjusted, and excess acrolein was removed following incubation by 72-h dialysis procedure as previously described, followed by sample freeze-drying and storage.
2.5. Oxidation of RBP by MDA
The method was followed in oxidizing RBP with MDA. First, a freshly-prepared MDA solution was used for this step, and this was prepared daily by hydrolyzing 1,1,3,3-tetramethoxypropane (TMP). Then, 8.4 mL (50 mM) TMP was mixed with 10.0 mL, 5.0 M of HCl, and 31.6 mL ultrapure water in the dark for 30 min to obtain MDA through acidic hydrolysis. The concentration of MDA in the stock solution is estimated by the absorbance at 267 nm when the solution is diluted to 10−5 using a molar extinction coefficient value of 31500 mol−1 cm−1.
2.6. RBP/MDA interactions
The procedure of Wu et al. (Citation2009) was followed to achieve this step. Briefly, prepared RBP solutions were mixed with different MDA concentrations, then hermetically incubated and shaken at 25°C in the dark for 1 d. The final concentration of MDA are 0 (control), 0.01, 0.1,1 and 10 mM. Following incubation, free MDA is removed from the RBP by 72-h dialysis at 4°C against deionized water. The oxidized RBP solutions are freeze-dried and stored at 4°C.
2.7. Protein carbonyl measurement
Carbonyl groups present in RBP samples were determined following Y. Huang et al., (Citation2006). Briefly, a reaction between RBP carbonyl proteins and 2,4-dinitrophenylhydrazine (DNPH) was initiated to produce protein hydrazones. The results are stated as nmol of carbonyl groups per milligram of soluble protein.
2.8. Measurement of disulfide bonds
The RBP disulfide bond contents were estimated following a modified Ellman’s method described by Sun et al., (Citation2011). The results were then expressed in nmol of disulfide bonds per milligram of protein with a molar extinction coefficient of 13600 M−1 cm−1.
2.9. Raman spectroscopic analysis
For this step, the procedure described by Tang et al. was followed. Briefly, 150 mg of RBP samples were analyzed using a Perkin Elmer Raman Station 400F Dispersive Raman Spectrometer (Waltham, MA, USA) (H. Tang et al., Citation2019). The Phe band, located near 1003 cm−1, is used as an internal normalization standard (Herrero, Citation2008). The Raman spectra of model polypeptides or monographs of Raman spectra of proteins were compared with reference values previously outlined (Li-Chan et al., Citation1994). We used the Peakfit 4.12 program to estimate RBP secondary structure under specific conditions. Triplicate Raman spectra values were determined for each sample.
2.10. Data analysis
All experiments were obtained from three independent assays and analyzed using the SAS software (SAS 8.12, SAS Institute Inc., Cary, NC). The one-way analysis of variance (ANOVA) technique assessed the relative intensity of each band as variables to determine the effect of each oxidation treatment. The Shapiro-Wilks and Kruskal-Wallis tests assessed samples’ normal and non-normal distribution. Statistical difference was measured at the P < .05 level using the Duncan’s multiple range test.
3. Results and discussion
3.1. Protein carbonyls
This study assessed how AAPH, MDA, and acrolein affect RBP carbonyl contents. In , about 3.3 nmol of carbonyl contents were detected in 1 mg of native RBP. We noticed that these parameters increased considerably following oxidation. In addition, a 2-fold carbonyl group increase was observed when oxidized RBP samples were incubated with 25 mmol/L AAPH. Previous studies reported that time and dose treatments had proportional correlations with AAPH treatment of rat and human protein cell lines (Chao et al., Citation1997; Kang et al., Citation2001). It is well established that proteins can be oxidatively modified, which gives rise to protein carbonyl groups through direct attack by ROS. Carbon-centered radicals are the first products of peroxyl radicals and protein molecule reactions which produce protein peroxyl radicals in the presence of oxygen. Further reactions can alter the conformation of the side chain groups and amino acid residue carbonylation (Headlam & Davies, Citation2004).
Table 1. Protein carbonyl of RBP incubated with increasing concentrations of AAPH, Acrolein and MDA.
Tabla 1. Carbonilo proteico de la RBP incubada con concentraciones crecientes de AAPH, acroleína y MDA.
The 10 mmol/L acrolein incubated-oxidized rice bran protein yielded a 4-fold carbonyl group increase compared to native RBP. It happens mainly because the double bonds of acrolein can react with the histidine residues imidazole group, the lysine residues ε-amino group, and the cysteine residues sulfhydryl group, therefore introducing new carbonyl groups into the protein molecule (Kawai et al., Citation2003). Acrolein is a kind of α, β-unsaturated aldehyde with the most potent oxidative activity; hence it is easy to induce carbonylation of proteins. Uchida et al. (Williams et al., Citation2006) have reported that the contents of carbonyl of bovine serum albumin increase with acrolein concentration and also found that acrolein could cross-link carbonylation with lysine and histidine residues of bovine serum albumin by Michael addition reaction, which increased the carbonyl contents of bovine serum albumin. Pocernich et al., (Citation2001) have found that a low concentration of acrolein could induce oxidation and significantly increase the carbonyl content of synaptosomes protein at room temperature. Mello et al., (Citation2007) also found that the carbonyl content of synaptosomes protein was increased linearly with different acrolein concentrations at 30°C temperature.
The carbonyl content of 10 mM MDA oxidized RBP was observed to be increased by a factor of 5 compared to the control. This observation aligns with a previous study that showed an increased production of carbonyl compounds in a pH and concentration-dependent manner upon exposure of bovine serum albumin to MDA (Burcham & Kuhan, Citation1996). Interactions involving MDA and His, Cys, and Lys side chain residues yielded carbonyl groups (Esterbauer et al., Citation1991). Furthermore, carbonyl groups become fused in the protein matrix when MDA reacts with primary amino protein groups (Busquets-Cortés et al., Citation2016).
It was found that MDA is similar to acrolein, can also react with lysine and histidine residues of bovine serum albumin to increase carbonyl content and produce Schiff’s base (Kawai et al., Citation2003; Uchida et al., Citation1997). Since two carbonyl groups are attached to an MDA molecule, when MDA oxidizes the carbonyl group of the protein, another carbonyl group can be introduced simultaneously (Slatter et al., Citation1999). Therefore, MDA-oxidized RBP has the highest carbonyl contents because it can introduce two carbonyl groups into protein structure.
3.2. Analysis with Raman spectroscopy
The different concentration levels of native and oxidized RBP were expressed as Raman spectra and selected band strata as illustrated in and , respectively. The assignments of some major bands are based on our previous works (L. Zhou et al., Citation2016). The change in frequency and intensity of the Raman band mainly represents secondary structure changes and RBP homeostasis fluctuations.
Figure 1. Raman spectrum of native and oxidized RBP. (a) AAPH induction; (b) acrolein induction; (c) MDA induction; (d) the second-derivative spectra of native RBP.
Figura 1. Espectro Raman de la RBP nativa y oxidada. (a) Inducción de AAPH; (b) Inducción de acroleína; (c) Inducción de MDA; (d) Espectros de la segunda derivada de la PBR nativa.
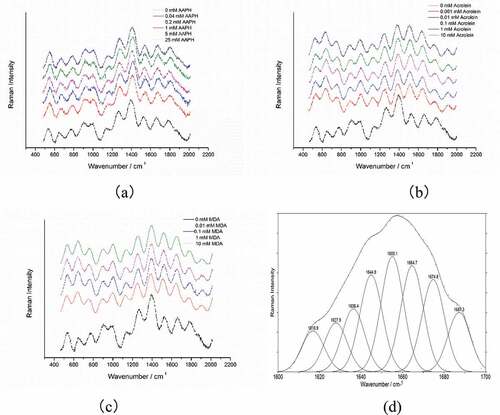
Table 2. Tentative assignment of some bands in the Raman spectrum of RBP.
Tabla 2. Asignación tentativa de algunas bandas en el espectro Raman de la RBP.
3.2.1. Determination of amide conformation
In this study, the amide I band of the Raman spectra was used to assess RBP conformations, and the band standards are shown thus: α-helix, 1645–1660 cm−1; β-sheet, 1670–1680 cm−1; β-turn, 1680–1690 cm−1; random coil, 1660–1670 cm−1 following an earlier procedure (L. Zhou et al., Citation2016). The Peakfit 4.12 software and amide I band spectral curve profile were used to estimate the RBP secondary structure within the Raman spectra as previously described (Alix et al., Citation1988).
The estimated percentage for the secondary structure of RBP is enlisted in . Native RBP has the highest Raman intensity in the range of 1665 1675 cm−1, suggesting that the β-sheet structure and unorder structures should be the dominant secondary structure of native RBP, as confirmed by the data previously reported in our study (L. Zhou et al., Citation2016). shows that native RBP contains 29.62% α-helix, 29.5% β-sheet, 13.1% β-turn, and 27.79% unordered structure.
Table 3. Percentages of protein secondary structure of rice bran protein at different oxidized condition.
Tabla 3. Porcentajes de la estructura secundaria de la proteína del salvado de arroz en diferentes condiciones de oxidación.
Table 4. Normalized intensities of the tryptophan band, tyrosyl doublet of RBP with different oxidation.
Tabla 4. Intensidades normalizadas de la banda de triptófano, doblete de tirosilo de la RBP con diferente oxidación.
In comparison to this, the contents of β-turn in oxidized RBP are higher, whereas the levels of α-helix structure of BRP oxidized by AAPH are lower than that of the native sample. The β-sheet and α-helix contents of oxidized RBP decreases at first and then increase with the concentration of AAPH. The decreased β-sheet and α-helix contents may be correlated with oxidation induced protein unfolding, AAPH oxidation may also weaken the hydrogen bonds stabilizing the β-sheet and α-helix structure. In an earlier study by Lee et al., (Citation2002), β-sheet was shown to play an essential role in protein clustering, which may account for their large reservoir of intact hydrogen bonds. It can be deduced that the increase in the β-sheet contents in oxidized RBP due to elevated AAPH concentrations may be related to the formation of aggregation. Our previous work found that oxidized RBP α-helix and β-sheet contents were first lowered and then increased as the AAPH concentration increased (L. Zhou et al., Citation2017). Choi & Ma, (Citation2005) pointed out that protein clustering could cause increased antiparallel β-sheet conformation. Wu et al., (Citation2009) reported that oxidation resulted in a gradual loss of α-helix structure of soy protein with a concomitant decrease of β-sheet structure. The β-turn and unordered structure contents of oxidized RBP initially increase and drop with elevated AAPH concentration.
When there is a 0.001 to 10 mmol/L rise in the acrolein concentration, the β-sheet and α-helix contents of oxidized RBP firstly decrease and then increase; however, the contents of the unordered structure firstly increased and then decreased. Oxidation by acrolein reduces the contents of β-turn. This phenomenon may be due to the low concentration of acrolein oxidization of RBP, which results in protein unfolding, while high acrolein levels promote more protein clustering in the oxidized RBP. According to previous research, acrolein changes can cause lowered α-helical structure and increased β-sheet structure (Wu et al., Citation2010). Tamamizu-Kato et al., (Citation2007) reported that acrolein-mediated apolipoprotein E (apoE3-NT) oxidation did not substantially change the protein secondary structure. The different results between acrolein-oxidized RBP, soy protein, and apoE3-NT could be attributed to the differences in oxidative environments and protein structure.
This study also reports that when MDA levels increase, there will be a proportional elevation in the α-helix and β-sheets contents of oxidized RBP followed by decreased contents of β-turn and unorderedindicating a more compact structure. These phenomena demonstrated that oxidation by MDA leads to the formation of RBP aggregation and accumulates with MDA concentration. F. Zhou et al., (Citation2014) also reported that the interaction between myofibrillar protein and MDA mainly leads to protein aggregation rather than a network. MDA has two free carbonyl groups, and therefore protein-bound MDA could form Schiff’s base with free amines, thus producing protein aggregates (Buttkus, Citation1967). The increasing contents of β-sheet were higher than the decreasing contents of β-turn, and it can be deduced that the increase in MDA oxidation may transform β-sheets into β-turns and also unordered structures.
The β-sheet contents of oxidized RBP by AAPH and acrolein both decrease first and then increase, which shows that RBP oxidized by AAPH and acrolein are both unfolding at low then aggregating at high concentration. However, the contents of the β-sheet of oxidized RBP by MDA have been increasing, indicating that RBP oxidized by MDA is aggregating at low concentrations. The α-helix contents usually increase accompanied by the β-sheets in RBP oxidized by three oxidants at a high concentration, which indicates that β-sheets dominate the stability of oxidized aggregates.
3.2.2. Side chain structure
Several Raman bands were detected in the Tryptophan (Trp) residue; previously, some have been used as microenvironment polarity indicators or affinity for hydrogen bonding. Li-Chan(ECY Li-Chan, Citation1996) demonstrated that tryptophan residues’ inactive hydrophobic surfaces become exposed to the polar aqueous solvent, possibly culminating in the lowered intensity of a band near the 760 cm−1 region. Some studies have also indicated that oxidation-induced protein structure unfolding can expose more tryptophan residues, which is shown in the decreased strength of stretch vibration peak of tryptophan corresponding to the protein Raman spectrum (Herrero, Citation2008; Ngarize et al., Citation2004; Xu et al., Citation2011).
As shown in , a significantly decreased Raman band intensity near 760 cm − 1 is observed in lower concentrations of AAPH and acrolein-oxidized RBP, which indicates that tryptophan residues tend to be exposed to the hydrophilic microenvironment. Some exposed hydrophobic groups interact with more oxidation, resulting in insoluble aggregates, increasing the Raman bands corresponding to Trp. It has been evidenced that the oxidation aggregates were formed via non-covalent interactions such as hydrophobic interaction, hydrogen bonding, or electrostatic interaction (Y. Huang et al., Citation2006).
Although, the intensities of the Raman bands corresponding to the stretching vibration of the tryptophan residues of oxidized RBP by MDA initially elevated, possibly because of protein aggregation formation and then lowered with partial oxidation degradation. Oxidative stress results in multiple structural changes in proteins, including oxidation of side-chain groups, backbone fragmentation, unfolding, degradation, and aggregation (X. Huang et al., Citation2017). Simat and Steinhart (Citation1998) found that the oxidation of the Trp residues may be degraded to 16 possible compounds. Ehrenshaft et al., (Citation2015) reported that tryptophan has the highest rate constant with commonly encountered biological oxidants.
The tyrosyl (Tyr) double ratio(I850/830) functions in assessing the tyrosyl residue region. This parameter has its lowest value (0.3) when these inactive residues, with carboxyl oxygen receive hydrogen bonds from the phenolic OH group. An earlier study showed that Tyr protein surface exposure increases the I850/830 value to about 1.25, ranging from 0.95 to 1.01 (Lord & Yu, Citation1970). This observation implied that native and oxidized RBP Tyr could have a possible dual function. Results in indicate that the tyrosine ratios of oxidized RBP generally increased with raised AAPH levels from 0.04 to 1 mmol/L, indicating exposure to the Tyr residues to a hydrophilic environment. As a result of oxidation and protein denaturation, there was an increase in structural flexibility, and some of the initially protected backbone amide groups were exposed (Saeed et al., Citation2006). The tyrosine doublet I850/830 ratios of oxidized RBP generally decrease as the AAPH concentration increases from 1 to 25 mmol/L, confirming the formation of oxidization aggregation and rebury tyrosine residues. The Tyr doublet I850/830 ratios of acrolein oxidized RBP increased at low concentrations, while the decrease at high oxidation agent concentration due to the formation of oxidation aggregation and tyrosine indicated that Tyr oxidation by acrolein could induce protein polymerization (Morzel et al., Citation2006). The tyrosine doublet I850/830 ratios of MDA oxidized RBP firstly decreased, which may be due to the formation of protein aggregation, and then increased with phenyl-epoxidation on Tyr.
A previous study had identified the CH2 and CH3 bending vibrations at about 1450 cm−1. The decrease in the intensity of these bands indicates the exposure of aliphatic residues, while an increase indicates buried residues (Herrero, Citation2008). By monitoring the changes in the spectra around 1450 cm−1 (C – H2 bending), the microenvironment of the aliphatic amino acid residues was investigated. It has been reported that the increased intensity of Raman bands causes the CH2 and CH3 bending vibrations to indicate an increased exposure of hydrophobic groups (Bouraoui et al., Citation1997). However, Herrero (Citation2008) and Li-Chan(ECY Li-Chan, Citation1996) found that the higher intensity of this band represents an increase in hydrophobic interaction of the aliphatic residues. There was no noticeable momentous change in the 1450 cm−1 band of RBP oxidized by three oxidants, probably because the aliphatic amino acids are not sensitive to RBP oxidation.
As shown in and , Disulfide bonds are the main force protein structure stability. The 500 550 cm−1 band range is created by joining the thiol groups of two cysteine molecules. A previous study showed that cysteine-containing protein and peptides are usually displayed in the 500 to 510 cm−1, which has been assigned to S-S stretching vibrations of disulfide bonds with the lowest potential energy is in the gauche-gauche-gauche (g-g-g) conformation, identified at the 515 and 540 cm−1 band range (Mohamed et al., Citation2005). Low concentration of AAPH and acrolein decrease the contents of g-g-g conformation, accompanied by the loss of disulfide bonds, but increases the content of g-g-t and t-g-t conformation, which indicates that oxidation of RBP mainly reduces the percentage of intramolecular g-g-g conformation, which may be helpful for the development of RBP structure. The content of g-g-g and g-g-t conformation increases following the increasing disulfide bonds by a high concentration of AAPH, confirming that the increasing disulfide bonds are mainly g-g-g and g-g-t restructuring. However, oxidation mediated by a high concentration of acrolein generally decreases the content of disulfide bonds, especially the intermolecular t-g-t conformation. In contrast, Trp and Tyr of oxidized RBP by a high concentration of acrolein both tended to be buried in oxidation aggregation, which suggested that oxidation aggregation is unstable through disulfide bonds but hydrophobic interactions. The loss of disulfide bonds induced by a low concentration of MDA oxidation is mainly related to the decreased content of g-g-g and t-g-t conformation, which indicates that oxidation induced by MDA attracts both intra-molecular and intermolecular disulfide bonds. However, the decreased contents of disulfide bonds induced by high MDA oxidation concentration correspond to lower g-g-t conformation levels.
Figure 2. The decomposition of the components of RBP in regions of 500 550.
Figura 2. Descomposición de los componentes de la RBP en las regiones de 500 550.
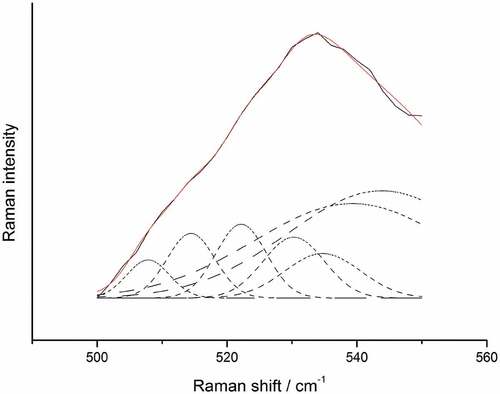
Table 5. Vibration mode assignments of the disulfide bonds in RBP with different oxidation.
Tabla 5. Asignaciones del modo de vibración de los enlaces disulfuro en la RBP con diferente oxidación.
However, the Raman can be used as a meansto characterize the structure of rice bran protein as affected by oxidation, but is not sufficient for structure study and needs further experiments to show the relation between your findings. For instance, FTIR and SDS pages, as well as SEM, are essential to get better results. These works will be further studied in our next work.
4. Conclusions
We used Raman spectroscopy to figure out the structural changes in RBP treated by oxidation. Raman spectroscopy is a powerful tool for verifying the structural characterization of oxidized protein. The structural change investigated through Raman spectroscopy can be used to reveal the mechanisms of protein oxidation. These findings can aid the refining of the function of RBP characteristics and controlling the adjustment of the storage time.
Disclosure statement
No potential conflict of interest was reported by the author(s).
Additional information
Funding
References
- Alix, A. J. P., Pedanou, G., & Berjot, M. (1988). Fast determination of the quantitative secondary structure of proteins by using some parameters of the Raman Amide I band. Journal of Molecular Structure, 174(1988), 159–164. https://doi.org/10.1016/0022-2860(88)80151-0
- Bouraoui, M., Nakai, S., & Li-Chan, E. (1997). In situ investigation of protein structure in Pacific whiting surimi and gels using Raman spectroscopy. Food Research International, 30(1), 65–72. https://doi.org/10.1016/S0963-9969(97)00020-3
- Burcham, P. C., & Kuhan, Y. T. (1996). Introduction of carbonyl groups into proteins by the lipid peroxidation product, malondialdehyde. Biochemical and Biophysical Research Communications, 220(3), 996–1001. https://doi.org/10.1006/bbrc.1996.0521
- Busquets-Cortés, C., Capó, X., Martorell, M., Tur, J. A., Sureda, A., & Pons, A. (2016). Training enhances immune cells mitochondrial biosynthesis, fission, fusion, and their antioxidant capabilities synergistically with dietary docosahexaenoic supplementation. Oxidative Medicine and Cellular Longevity, 2016, 1–10. https://doi.org/10.1155/2016/8950384
- Buttkus, H. (1967). The reaction of myosin with malonaldehyde. Journal of Food Science, 32(4), 432–434. https://doi.org/10.1111/j.1365-2621.1967.tb09703.x
- Carbone, D. L., Doorn, J. A., Kiebler, Z., & Petersen, D. R. (2005). Cysteine modification by lipid peroxidation products inhibits protein disulfide isomerase. Chemical Research in Toxicology, 18(8), 1324–1331. https://doi.org/10.1021/tx050078z
- Chang, R. L., Stanley, J. A., Robinson, M. C., Sher, J. W., Li, Z., Chan, Y. A., Omdahl, A. R., Wattiez, R., Godzik, A., & Matallana‐surget, S. (2020). Protein structure, amino acid composition and sequence determine proteome vulnerability to oxidation‐induced damage. The EMBO Journal, 39(23), e104523. https://doi.org/10.15252/embj.2020104523
- Chao, C.-C., Ma, Y.-S., & Stadtman, E. R. (1997). Modification of protein surface hydrophobicity and methionine oxidation by oxidative systems. Proceedings of the National Academy of Sciences, 94(7), 2969. https://doi.org/10.1073/pnas.94.7.2969
- Choi, S.-M., & Ma, C.-Y. (2005). Conformational study of globulin from common buckwheat (Fagopyrum esculentum Moench) by Fourier transform infrared spectroscopy and differential scanning calorimetry. Journal of Agricultural and Food Chemistry, 53(20), 8046–8053. https://doi.org/10.1021/jf051040v
- Ehrenshaft, M., Deterding, L. J., & Mason, R. P. (2015). Tripping up Trp: Modification of protein tryptophan residues by reactive oxygen species, modes of detection, and biological consequences. Free Radical Biology & Medicine, 89(2015), 220–228. https://doi.org/10.1016/j.freeradbiomed.2015.08.003
- Esterbauer, H., Schaur, R. J., & Zollner, H. (1991). Chemistry and biochemistry of 4-hydroxynonenal, malonaldehyde and related aldehydes. Free Radical Biology & Medicine, 11(1), 81–128. https://doi.org/10.1016/0891-5849(91)90192-6
- Fabian, C., & Ju, Y.-H. (2011). A review on rice bran protein: Its properties and extraction methods. Critical Reviews in Food Science and Nutrition, 51(9), 816–827. https://doi.org/10.1080/10408398.2010.482678
- Gieseg, S., Duggan, S., & Gebicki, J. M. (2000). Peroxidation of proteins before lipids in U937 cells exposed to peroxyl radicals. The Biochemical Journal, 350(1), 215. https://doi.org/10.1042/bj3500215
- Headlam, H. A., & Davies, M. J. (2004). Markers of protein oxidation: Different oxidants give rise to variable yields of bound and released carbonyl products. Free Radical Biology & Medicine, 36(9), 1175–1184. https://doi.org/10.1016/j.freeradbiomed.2004.02.017
- Herrero, A. M. (2008). Raman spectroscopy for monitoring protein structure in muscle food systems. Critical Reviews in Food Science and Nutrition, 48(6), 512–523. https://doi.org/10.1080/10408390701537385
- Huang, Y., Hua, Y., & Qiu, A. (2006). Soybean protein aggregation induced by lipoxygenase catalyzed linoleic acid oxidation. Food Research International, 39(2), 240–249. https://doi.org/10.1016/j.foodres.2005.07.012
- Huang, X., Sontag-Strohm, T., Stoddard, F. L., & Kato, Y. (2017). Oxidation of proline decreases immunoreactivity and alters structure of barley prolamin. Food Chemistry, 214(2017), 597–605. https://doi.org/10.1016/j.foodchem.2016.07.108
- Kang, J. H., Kim, K. S., Choi, S. Y., Kwon, H. Y., & Won, M. H. (2001). Oxidative modification of human ceruloplasmin by peroxyl radicals. Biochimica et Biophysica Acta (BBA) - General Subjects, 1568(1), 30–36. https://doi.org/10.1016/S0304-4165(01)00198-2
- Kawai, Y., Kato, Y., Fujii, H., Makino, Y., Mori, Y., Naito, M., & Osawa, T. (2003). Immunochemical detection of a novel lysine adduct using an antibody to linoleic acid hydroperoxide-modified protein. Journal of Lipid Research, 44(6), 1124–1131. https://doi.org/10.1194/jlr.M200442-JLR200
- Lee, H.-J., Choi, C., & Lee, S.-J. (2002). Membrane-Bound α-synuclein has a high aggregation propensity and the ability to seed the aggregation of the cytosolic form. The Journal of Biological Chemistry, 277(1), 671–678. https://doi.org/10.1074/jbc.M107045200
- Li-Chan, E. (1996). The applications of Raman spectroscopy in food science. Trends in Food Science & Technology, 11(7), 361–370. https://doi.org/10.1016/S0924-2244(96)10037-6
- Li-Chan, E., Nakai, S., & Hirotsuka, M. (1994). Raman spectroscopy as a probe of protein structure in food systems. In R. Y. Yada, R. L. Jackman, & J. L. Smith (Eds.), Protein structure-function relationships in foods (pp. 163–197). Springer US. https://doi.org/10.1007/978-1-4615-2670-4_8
- Lord, R. C., & Yu, N.-T. (1970). Laser-Excited Raman spectroscopy of biomolecules: I. Native lysozyme and its constituent amino acids. Journal of Molecular Biology, 50(2), 509–524. https://doi.org/10.1016/0022-2836(70)90208-1
- Mawal, Y. R., Mawal, M. R., & Ranjekar, P. K. (1987). Biochemical and immunological characterization of rice albumin. Bioscience Reports, 7(1), 1–9. https://doi.org/10.1007/BF01122721
- Mello, C. F., Sultana, R., Piroddi, M., Cai, J., Pierce, W. M., Klein, J. B., & Butterfield, D. A. (2007). Acrolein induces selective protein carbonylation in synaptosomes. Neuroscience, 147(3), 674–679. https://doi.org/10.1016/j.neuroscience.2007.04.003
- Mohamed, A., Peterson, S. C., Hojilla-Evangelista, M. P., Sessa, D. J., Rayas Duarte, P., & Biresaw, G. (2005). Effect of heat treatment and pH on the thermal, surface, and rheological properties of Lupinus albus protein. Journal of the American Oil Chemists’ Society, 82(2), 135–140. https://doi.org/10.1007/s11746-005-1055-8
- Morzel, M., Gatellier, P., Sayd, T., Renerre, M., & Laville, E. (2006). Chemical oxidation decreases proteolytic susceptibility of skeletal muscle myofibrillar proteins. Meat Science, 73(3), 536–543. https://doi.org/10.1016/j.meatsci.2006.02.005
- Mousavi, S. M. R., Rafe, A., & Yeganehzad, S. (2020). Structure-Rheology relationships of composite gels: Alginate and Basil seed gum/guar gum. Carbohydrate Polymers, 232(2020), 115809. https://doi.org/10.1016/j.carbpol.2019.115809
- Ngarize, S., Adams, A., & Howell, N. K. (2004). Studies on egg albumen and whey protein interactions by FT-Raman spectroscopy and rheology. Food Hydrocolloids, 18(1), 49–59. https://doi.org/10.1016/S0268-005X(03)00041-9
- Pocernich, C. B., Cardin, A. L., Racine, C. L., Lauderback, C. M., & Allan Butterfield, D. (2001). Glutathione elevation and its protective role in acrolein-induced protein damage in synaptosomal membranes: Relevance to brain lipid peroxidation in neurodegenerative disease. Neurochemistry International, 39(2), 141–149. https://doi.org/10.1016/S0197-0186(01)00012-2
- Rafe, A., & Sadeghian, A. (2017). Stabilization of Tarom and Domesiah cultivars rice bran: Physicochemical, functional and nutritional properties. Journal of Cereal Science, 74(2017), 64–71. https://doi.org/10.1016/j.jcs.2017.01.019
- Rafe, A., Sadeghian, A., & Hoseini‐yazdi, S. Z. (2017). Physicochemical, functional, and nutritional characteristics of stabilized rice bran form tarom cultivar. Food Science & Nutrition, 5(3), 407–414. https://doi.org/10.1002/fsn3.407
- Refsgaard, H. H. F., Tsai, L., & Stadtman, E. R. (2000). Modifications of proteins by polyunsaturated fatty acid peroxidation products. Proceedings of the National Academy of Sciences, 97(2), 611. https://doi.org/10.1073/pnas.97.2.611
- Saeed, S., Gillies, D., Wagner, G., & Howell, N. K. (2006). ESR and NMR spectroscopy studies on protein oxidation and formation of dityrosine in emulsions containing oxidised methyl linoleate. Food and Chemical Toxicology, 44(8), 1385–1392. https://doi.org/10.1016/j.fct.2006.03.005
- Santillán Deiú, A., Miglioranza, K. S., Ondarza, P. M., & de la Torre, F. R. (2021). Exposure to environmental concentrations of fipronil induces biochemical changes on a neotropical freshwater fish. Environmental Science and Pollution Research, 28(32), 43872–43884. https://doi.org/10.1007/s11356-021-13786-w
- Shacter, E. (2000). Quantification and significance of protein oxidation in biological samples. Drug Metabolism Reviews, 32(3–4), 307–326. https://doi.org/10.1081/DMR-100102336
- Shoji, Y., Mita, T., Isemura, M., Mega, T., Hase, S., Isemura, S., & Aoyagi, Y. (2001). A fibronectin-binding protein from rice bran with cell adhesion activity for animal tumor cells. Bioscience, Biotechnology, and Biochemistry, 65(5), 1181–1186. https://doi.org/10.1271/bbb.65.1181
- Simat, T. J., & Steinhart, H. (1998). Oxidation of free tryptophan and tryptophan residues in peptides and proteins. Journal of Agricultural and Food Chemistry, 46(2), 490–498. https://doi.org/10.1021/jf970818c
- Slatter, D. A., Paul, R. G., Murray, M., & Bailey, A. J. (1999). Reactions of lipid-derived malondialdehyde with collagen. The Journal of Biological Chemistry, 274(28), 19661–19669. https://doi.org/10.1074/jbc.274.28.19661
- Sun, W., Cui, C., Zhao, M., Zhao, Q., & Yang, B. (2011). Effects of composition and oxidation of proteins on their solubility, aggregation and proteolytic susceptibility during processing of Cantonese sausage. Food Chemistry, 124(1), 336–341. https://doi.org/10.1016/j.foodchem.2010.06.042
- Tamamizu-Kato, S., Wong, J. Y., Jairam, V., Uchida, K., Raussens, V., Kato, H., Ruysschaert, J.-M., & Narayanaswami, V. (2007). Modification by acrolein, a component of tobacco smoke and age-related oxidative stress, mediates functional impairment of human apolipoprotein E. Biochemistry, 46(28), 8392–8400. https://doi.org/10.1021/bi700289k
- Tang, H., Fu, T., Feng, Y., Zhang, S., Wang, C., & Zhang, D. (2019). Effect of heat treatment on solubility, surface hydrophobicity and structure of rice bran albumin and globulin. Quality Assurance and Safety of Crops & Foods, 11(6), 499–509. https://doi.org/10.3920/QAS2018.1402
- Tang, S., Hettiarachchy, N. S., Horax, R., & Eswaranandam, S. (2003). Physicochemical properties and functionality of rice bran protein hydrolyzate prepared from heat-stabilized defatted rice bran with the aid of enzymes. Journal of Food Science, 68(1), 152–157. https://doi.org/10.1111/j.1365-2621.2003.tb14132.x
- Tironi, V. A., Tomás, M. C., & Añón, M. C. (2006). Structural and functional changes in myofibrillar proteins of Sea salmon (Pseudopercis semifasciata) by interaction with malonaldehyde (RI). Journal of Food Science, 67(3), 929–935. https://doi.org/10.1111/j.1365-2621.2002.tb09430.x
- Uchida, K., Sakai, K., Itakura, K., Osawa, T., & Toyokuni, S. (1997). Protein modification by lipid peroxidation products: Formation of malondialdehyde-DerivedNϵ-(2-Propenal)lysine in proteins. Archives of Biochemistry and Biophysics, 346(1), 45–52. https://doi.org/10.1006/abbi.1997.0266
- Venlet, N. V., Hettinga, K. A., Schebesta, H., & Bernaz, N. (2021). Perspective: A legal and nutritional perspective on the introduction of Quinoa-Based infant and follow-on formula in the EU. Advances in Nutrition, 12(4), 1100–1107. https://doi.org/10.1093/advances/nmab041
- Williams, T. I., Lynn, B. C., Markesbery, W. R., & Lovell, M. A. (2006). Increased levels of 4-hydroxynonenal and acrolein, neurotoxic markers of lipid peroxidation, in the brain in mild cognitive impairment and early Alzheimer’s disease. Neurobiology of Aging, 27(8), 1094–1099. https://doi.org/10.1016/j.neurobiolaging.2005.06.004
- Wu, W., Wu, X., & Hua, Y. (2010). Structural modification of soy protein by the lipid peroxidation product acrolein. LWT - Food Science and Technology, 43(1), 133–140. https://doi.org/10.1016/j.lwt.2009.05.006
- Wu, W., Zhang, C., Kong, X., & Hua, Y. (2009). Oxidative modification of soy protein by peroxyl radicals. Food Chemistry, 116(1), 295–301. https://doi.org/10.1016/j.foodchem.2009.02.049
- Xu, X.-L., Han, M.-Y., Fei, Y., & Zhou, G.-H. (2011). Raman spectroscopic study of heat-induced gelation of pork myofibrillar proteins and its relationship with textural characteristic. Meat Science, 87(3), 159–164. https://doi.org/10.1016/j.meatsci.2010.10.001
- Yan, S., Xu, J., Liu, G., Du, X., Hu, M., Zhang, S., Jiang, L., Zhu, H., Qi, B., & Li, Y. (2022). Emulsions co-stabilized by soy protein nanoparticles and tea saponin: Physical stability, rheological properties, oxidative stability, and lipid digestion. Food Chemistry, 387(2022), 132891. https://doi.org/10.1016/j.foodchem.2022.132891
- Zhou, L., Yang, Y., Ren, H., Zhao, Y., Wang, Z., Wu, F., & Xiao, Z. (2016). Structural changes in rice bran protein upon different extrusion temperatures: A Raman spectroscopy study. Journal of Chemistry, 2016, 1–8. https://doi.org/10.1155/2016/6898715
- Zhou, L., Zhang, Y., Zhao, C., Lin, H., Wang, Z., & Wu, F. (2017). Structural and functional properties of rice bran protein oxidized by peroxyl radicals. International Journal of Food Properties, 20(2),1456-1467. Doi: 10.1080/10942912.2017.1352596.
- Zhou, F., Zhao, M., Su, G., Cui, C., & Sun, W. (2014). Gelation of salted myofibrillar protein under malondialdehyde-induced oxidative stress. Food Hydrocolloids, 40(2014), 153–162. https://doi.org/10.1016/j.foodhyd.2014.03.001