ABSTRACT
The severe threat of microbial contamination to human life and health safety necessitates urgent research and development of antimicrobial materials capable of inhibiting or eradicating pathogens. Cyclodextrin metal-organic framework (CD-MOF) was utilized as a carrier to encapsulate gold nanoparticles (AuNPs), resulting in a composite material (Au@CD-MOF) that exhibited remarkable antibacterial properties. The antibacterial study revealed that Au@CD-MOF completely eradicated Staphylococcus aureus (S. aureus) and Listeria monocytogenes (L. monocytogenes) at a concentration of approximately 1 × 105 CFU/mL after 48 h and 36 h, respectively. Moreover, Au@CD-MOF also demonstrated the complete elimination of Escherichia coli O157:H7 (E. coli O157:H7), Pseudomonas aeruginosa PAO1 (P. aeruginosa PAO1), and Salmonella typhimurium (S. typhimurium) at a concentration of approximately 1 × 105 CFU/mL after 48 h. Moreover, the antibacterial mechanism of Au@CD-MOF was investigated using E. coli O157:H7 and S. aureus as model organisms. The findings revealed that Au@CD-MOF disrupted the integrity of cell membranes and diminished their potential, consequently impeding the normal functions of the bacteria. Additionally, the heightened permeability of cell membranes induced by Au@CD-MOF resulted in substantial leakage of nucleic acids and proteins, ultimately leading to bacterial eradication.
Highlights
Cyclodextrin metal-organic framework loaded with AuNPs has an excellent antibacterial ability.
Antibacterial mechanism of cyclodextrin metal-organic framework loaded with AuNPs was investigated.
1. Introduction
Nowadays, microbial contamination is becoming a serious issue as it can affect various stages of food processing and distribution, potentially leading to significant impacts on human health and the food industry (Paparella & Maggio, Citation2023). Foodborne illnesses caused by pathogenic bacteria continuously present challenges to food safety. Even low amounts of these bacteria in food and water can result in severe and potentially fatal diseases (A. Zhu et al., Citation2023). According to data from the World Health Organization (WHO), almost 500,000 individuals die each year due to foodborne diseases (Atay & Altan, Citation2023). Therefore, there is an urgent need to develop strategies to combat foodborne diseases. However, antibiotic overuse has led to bacterial resistance, highlighting the necessity for new approaches such as antibacterial materials to fight bacterial infections (Borreby et al., Citation2023).
Antibacterial agents such as quaternary ammonium salts (QAS), antimicrobial peptides, and graphene oxide sheets have been gradually developed and have shown excellent antibacterial effects. However, they also have certain limitations, such as the potential for bacterial resistance and other deficiencies (Fu et al., Citation2021). Among these agents, metal-organic frameworks (MOFs) have emerged as increasingly important players. By selecting suitable metastable coordination bonds, MOFs can serve as reservoirs and release antibacterial metal ions. Moreover (Han et al., Citation2022), MOFs possess distinct advantages in terms of excellent designability and porous structures. The remarkable pore structure and chemical properties of MOFs make them attractive for potential applications in various fields (X. Zhao et al., Citation2021). Nevertheless, their potential biological toxicity restricts their usage in the food sector. Consequently, researchers have shifted their focus towards developing biocompatible MOFs to address this concern. In this regard, CD-MOF has emerged as a promising alternative. CD-MOF is synthesized using naturally occurring carbohydrate cyclodextrin and alkali metal cations, and possesses renewable, non-toxic, and edible characteristics (R.-N. Zhao et al., Citation2023; Smaldone et al., Citation2010). Therefore, CD-MOF can be applied in the food industry. Furthermore, their porous structure contains numerous spherical voids that can serve as carriers for delivering antibacterial agents (Y. Wei et al., Citation2012). Metal nanoparticles are one of the most widely investigated antibacterial agents, capable of efficiently inactivating bacteria through multiple mechanisms (Doghish et al., Citation2022; Salem, Citation2022a, Citation2022b; Soliman et al., Citation2022). These nanoparticles can deposit on the cell membrane, disrupt it, penetrate it, interfere with cell metabolism, and ultimately inactivate bacteria (Han et al., Citation2022). Furthermore, nanoparticles, particularly AuNPs, have garnered significant attention from researchers due to their low toxicity and favorable biocompatibility (He et al., Citation2023), broad-spectrum antibacterial properties, and its fabrication process is environmentally friendly (Salem, Citation2023; Salem & Fouda, Citation2020).
Therefore, in this study, CD-MOF was used as a carrier to load AuNPs, resulting in the synthesis of a composite material known as Au@CD-MOF. The antibacterial efficacy of Au@CD-MOF was found to be excellent against both Gram-positive and Gram-negative bacteria. Au@CD-MOF achieved bacterial eradication by damaging the cell membrane, reducing cell membrane potential, and increasing cell membrane permeability. In summary, Au@CD-MOF exhibits significant potential for applications in the field of food antibacterial field.
2. Materials and methods
2.1. Materials
γ-CD (98%, CAS number 1746-86-0) and potassium hydroxide (KOH, 90%, CAS number 1310-58-3) were purchased from Shanghai Macklin Biochemical Co. Ltd. (Shanghai, China). Polyethylene glycol 8000 (PEG 8000, CAS number 25,322-68-3) was obtained from Shanghai Aladdin Bio-Chem Technology Co. Ltd. (Shanghai, China). Methanol (MeOH, 99.5%, CAS number 67-56-1), acetonitrile (99.5%, CAS number 75-05-8), chloroauric acid (47.8%, CAS number 16,903-35-8), and dichloromethane (99.5%, CAS number 75-09-2) were purchased from Sinopharm Chemical Reagents Co. Ltd. (Shanghai, China). LB Broth and LB Nutrient Agar, as well as strains of S. aureus ATCC 25,923, L. monocytogenes ATCC 19,114, E. coli O157:H7 ATCC 35,150, P. aeruginosa PAO1, and S. typhimurium ATCC 14,028 were obtained from Qingdao Hope Bio-technology Co., Ltd. (Qingdao, China). Phosphate-buffered saline (PBS) (0.1 M, pH = 7.0) was purchased from Beijing Solarbio Science & Technology Co., Ltd. (Beijing, China). Experimental ultra-pure water was processed by the Smart-N system (Heal Force Biotech, HK).
2.2. Methods
2.2.1. Preparation and characterization of CD-MOF and Au@CD-MOF
2.2.1.1. Preparation of CD-MOF and Au@CD-MOF
CD-MOF was synthesized using a method based on a previously reported study (J. Chen et al., Citation2022). The Au@CD-MOF was obtained following the method described by (Y. Wei et al., Citation2012). The CD-MOF particles (46.5 mg) were immersed in 5 mL of acetonitrile containing 1.25 mM chloroauric acid. The suspension was gently shaken at 60 rpm and incubated for 21 h under dark conditions at 37°C. The resulting Au@CD-MOF particles were collected from the reaction solution by centrifugation (5000 rpm, 5 min). Subsequently, the particles were washed several times with acetonitrile and dried under vacuum at 60°C for 5 h to obtain the Au@CD-MOF material. In the supporting information, the traditional method for preparing gold nanoparticles (T-AuNPs) was described.
2.2.1.2. Characterization of CD-MOF and Au@CD-MOF
A scanning electron microscope (SEM) (Gemini SEM 360, ZEISS, Germany) fitted with an Energy-Dispersive X-ray was (EDX) used to analyze CD-MOF and Au@CD-MOF, with an acceleration voltage of 3 keV. A transmission electron microscope (TEM) (JEM-1230 TEM, HITACHI, Japan) was used to observe the structure of MOF crystals. Prior to TEM imaging, the samples were sonicated in methanol or water for 10 min and then placed onto copper mesh. Powder X-ray diffraction (PXRD) (D8 Advance, Bruker, Germany) was performed on MOF crystals with an experimental condition of 40 kV tube voltage and 40 mA tube current. All the samples were analyzed over a 2θ angle range of 2°- 90° at a stepwise scan increment of 0.02° with a scanning speed of 0.1 s step−1. The PXRD of the simulated CD-MOF was calculated using Materials Studio 2017 software (Cambridge Crystallographic Data Centre). The confirmation of AuNPs released in water and generated inside CD-MOF was carried out using the Ultraviolet Spectrophotometer (UV-2600, Shimadzu, Japan). To prepare the samples, Au@CD-MOF were dissolved in pure water at a concentration of 1 mg/mL. The UV-vis absorbance spectra were recorded over a wavelength range of 300 nm to 700 nm. Additionally, the zeta potential of T-AuNPs and Au@CD-MOF (dissolved in water) was measured (Zeta Sizer Nano ZS90, Malvern, Britain) with a concentration of 0.1 mg/mL. A Fourier transform infrared spectrometer (FTIR) (Nicolet IS50, Thermo Fisher, U.S.A.) was used to collect spectra of Au@CD-MOF crystals and composite membranes. Au@CD-MOF crystals were thoroughly ground and mixed with potassium bromide before being pressed into a translucent sheet for testing. The wave number range was 500–4000 cm−1 with a resolution of 4 cm−1. A Raman spectrometer (XploRA Plus, Horiba, Japan) was utilized to obtain the Raman spectra of Au@CD-MOF. Prior to measurement, Au@CD-MOF crystals were placed on a microscope slide. The spectrometer employed an excitation wavelength of 755 nm and had a spectral acquisition range of 200–3200 cm−1 with a resolution of approximately 1 cm−1. Each spectrum was acquired for an integration time of 10 s, with 2 integrations performed and a laser power of around 10 mW. Three spectra were randomly collected from different positions on the aluminum foil for each sample.
2.2.2. Antibacterial study
The antibacterial method employed in this study followed a modified approach previously reported in the literature (Shen et al., Citation2022). The above five bacterial strains were removed from a −70°C refrigerator. They were then inoculated onto LB agar plates and cultivated for 24 h at 37°C. A bacterial colony of each strain was selected and cultured in LB medium with continuous shaking (180 rpm) at 37°C for 17 h. The bacterial concentrations were adjusted to 1 × 106 CFU/mL with sterile PBS, using the plate counting method. Sterile Au@CD-MOF crystals were added into the bacterial solution to achieve a concentration of AuNPs with 300 μg/mL, while a PBS bacterial solution without any Au@CD-MOF crystals was used as a positive control. The above solutions were stirred at 180 rpm and 37°C for 48 h. To monitor the antibacterial activity, bacterial counts were obtained at specific time intervals using the plate counting method. It is worth noting that the control group bacterial suspensions used for plate counting method were pre-diluted 1000 times, while the experimental group bacterial suspensions were not diluted. The time-inactivate curves of Au@CD-MOF against bacteria were analyzed by assessing bacterial viability at various time points (0, 1, 3, 6, 12, 24, 36, and 48 h). Each sample was tested in triplicate to ensure accuracy and reproducibility.
2.2.3. Antibacterial mechanism
S. aureus and E. coli O157:H7 were taken as model organisms to study antibacterial mechanism.
2.2.3.1. Observation of bacteria by SEM
After treatment with Au@CD-MOF, the morphology of S. aureus and E. coli O157:H7 was observed using SEM. The untreated bacterial samples and the bacterial samples treated for 2 h with Au@CD-MOF solution at a concentration of 300 μg/mL were centrifuged at 10,000 rpm for 3 min. Then, the samples were rinsed with sterile PBS solution to obtain a precipitate. A 2.5% glutaraldehyde solution was added to the precipitate, which was then fixed overnight in a refrigerator at 4°C. On the next day, the fixed solution was carefully removed and the samples were rinsed three times with PBS solution, each time lasting 15 min. The samples were then fixed with a 1% osmic acid solution for over 2 h. After carefully removing the osmic acid waste solution from the fume hood, the samples were rinsed three times with PBS solution, each time lasting 15 min. Subsequently, the samples were dehydrated with 30%, 50%, 70%, 80%, 90%, and 95% ethanol for 15 min each and then soaked in anhydrous ethanol for 20 min. Finally, the samples were dried using a critical point dryer (Hitachi HCP-2, Japan) and observed using a scanning electron microscope (Hitachi SU-8010, Japan).
2.2.3.2. Assessment of bacterial cell membrane integrity
The propyl iodide (PI) staining method was used to assess the integrity of bacterial cell membranes (Tang et al., Citation2017). Bacterial solutions (1 mL) treated with 300 μg/mL Au@CD-MOF for 24 h were centrifuged at 8,000 rpm for 10 min at 25°C. The samples were then rinsed three times with PBS buffer solution and resuspended in 1 mL PI solution (20 μM) for 30 min in a dark condition at room temperature. Subsequently, a Multimode microplate reader (INFINITE E PLEX, Tecan Austria Gmbh, Austria) was used to measure the fluorescence spectra of the above solutions. The excitation wavelength was set at 550 nm, and the emission wavelength ranged from 620 nm to 700 nm. The Gain value was set to 80.
2.2.3.3. Assessment of bacterial cell membrane potential
The Rhodamine 123 (Rh 123) fluorescence staining method was used to assess the bacterial cell membrane potential (Y. Zhang et al., Citation2016). Bacterial solutions (1 mL) treated with 300 μg/mL Au@CD-MOF for 24 h were centrifuged at 8,000 rpm for 10 minutes at 25°C. The samples were then rinsed three times with PBS buffer solution and resuspended in 1 mL Rh 123 solution (2 μg/mL) for 30 min in a dark condition at room temperature. Additionally, the samples were rinsed twice with PBS buffer solution to remove residual Rhodamine 123. Finally, a Multimode microplate reader was used to measure the fluorescence spectra of the above solutions. The excitation wavelength was set at 480 nm, and the emission wavelength was set at 530 nm. The Gain value was set to 80.
2.2.3.4. Assessment of bacterial cell membrane permeability
When bacterial membranes are broken or perforated, the cytoplasmic contents are released into the surrounding environment. Therefore, the integrity of the bacterial cell membrane was examined using an Ultraviolet-visible spectrophotometer (NanoDrop One/One C, Thermo Scientific, U.S.A.) at 260 nm and 280 nm. The amounts of nucleic acids and protein released were calculated based on the optical density (OD) values at 260 nm and 280 nm, as described previously (Kim et al., Citation2022; B. Zhang et al., Citation2023). The bacterial solutions (1 mL) treated with 300 μg/mL Au@CD-MOF for 24 h were centrifuged at 8,000 rpm for 10 min at 25°C. Then, the supernatant was collected to measure the absorbance at 260 nm and 280 nm. Prior to the measurement, baseline correction was performed using an Au@CD-MOF solution with a concentration of 300 μg/mL.
2.2.4. Data analysis
All data were subjected to analysis of variance (ANOVA) using the IBM SPSS Statistics 25.0, and a Tukey test was used to compare the means when the overall P yalue for an experimentwas below the threshold of statistical significance (P < 0.05). The data were plotted using Origin 2018 and GraphPad Prism 9, with each data obtained from three independent replicate experiments.
3. Results and discussions
3.1. Characterization of CD-MOF and Au@CD-MOF
SEM and TEM were used to observe the morphology and fine structure of MOF crystals, respectively. The EDX image of Au@CD-MOF was presented in Figure S3, revealing highly consistent distribution patterns between the Au and K signals, indicating uniform distributions of AuNPs within the Au@CD-MOF structure. The CD-MOF exhibited a typical cubic shape with excellent uniformity (Figure S1 [A(a)] and [A(b)]). After loading with AuNPs (Figure S1 [B(a)] and [B(b)]), the Au@CD-MOF crystals underwent slight deformation due to solvent effects; however, their appearance and size remained unchanged. TEM results (Figure S1 [A(c), B(c)]) displayed that the cubic structures of both MOF crystals were maintained. Furthermore, small nanoparticles were observed inside Au@CD-MOF while there was nothing in CD-MOF structure (Figure S1 [A(d), B(d)]). These small nanoparticles inside the Au@CD-MOF may be gold nanoparticles.
PXRD results (Figure S2) revealed the crystal structures for both CD-MOF and Au@CD-MOF. Characteristic peaks corresponding to the simulated and reported results were observed at 2θ values of 4.0°, 5.64°, 6.94°, 13.35°, and 16.64° for CD-MOF (B. Zhang et al., Citation2021). In addition to possessing characteristic peaks similar to those of CD-MOF, the pattern obtained from the PXRD of Au@CD-MOF also showed distinct peaks at different angles (38.2°, 44.4°, 64.5°, and 77.5°) for gold (111), (200), (220), and (311) diffraction planes, respectively. These findings are aligned with previous studies (Shah et al., Citation2021). PXRD results showed that the generation of AuNPs inside CD-MOF did not significantly alter the characteristic peaks of the CD-MOF. This suggested that the CD-MOF retained its crystal structure and successfully generated AuNPs.
The TEM image () displayed Au@CD-MOF dissolved in water. The figure showed that there numberous AuNPs dispersed in water. However, the absence of a cubic shape in the TEM image of Au@CD-MOF can be attributed to the structure of CD-MOF completely decomposed due to its high hydrophilicity. The UV absorbance curve () of Au@CD-MOF dissolved in water demonstrated the presence of AuNPs, as evidenced by the absorbance peak at approximately 520 nm. Moreover, the absorbance curves showed that Au@CD-MOF released AuNPs rapidly and completely, as indicated by the similarity between the 0 h and 48 h curves. Additionally, zeta potential is commonly used to assess the repulsion forces between nanoparticles in colloidal suspensions, providing insight into their stability against particle aggregation (Shan et al., Citation2023). The zeta potential of Au@CD-MOF was measured to be −36.1 mV, similar to the −33.1 mV observed for T-AuNPs (). This result suggested that the AuNPs encapsulated within CD-MOF exhibited good colloidal stability. The FTIR and Raman spectra of Au@CD-MOF were supplied in Figure S4 and Figure S5, respectively.
3.2. Antibacterial study
3.2.1. The result of Au@CD-MOF against S. aureus
S. aureus still viable after the treatment of Au@CD-MOF for 12 h (). However, when comparing the 12 h treatment to 24 h treatment, it clearly showed that the bacterial count decreased as the treatment time extended. illustrated the bacterial time-inactivate profile of S. aureus and it showed the bacterial count of experimental group significantly decreased from 6.1 log to 0.3 log compared to the control group just decreased from 6.1 log to 5.3 log. showed the inactivated bacterial count increased as treatment time prolonged. At a treatment time of 1 h, the inactivated bacterial count was 0.78 log. When treatment time extended to 3 h, the inactivated bacterial count increased to 1.4 log. Continuously extending the treatment time to 6 h, 12 h, 24 h, 36 h and 48 h, resulted in an increase of 3 log, 3.3 log, 4 log, 5.8 log, and 5.3 log in the inactivated bacterial count, respectively. Above results indicated that Au@CD-MOF had a good antibacterial property against S. aureus. These findings differed from a study that reported strong hydrophilic-dependent antimicrobial activity of AuNPs which with increased hydrophobicity showed less antimicrobial efficiency toward gram-positive S. aureus (Linklater et al., Citation2020). Additionally, Wei et al., reported that 16 ug/ml of silver nanoparticles composite inactivated 100% S. aureus with a concentration at 1.0 × 105 CFU/mL (H. Wei et al., Citation2024).
Figure 2. Optical images of agar plates characteristic of antibacterial activities of Au@CD-MOF against S. aureus under 12 h and 24 h and bacterial solution of the control group was prediluted 103 times (a); Bacterial time-inactivate profiles of Au@CD-MOF against S. aureus (b); inactivated bacterial count of Au@CD-MOF against S. aureus (c).
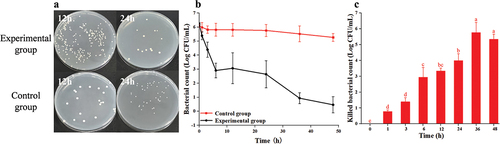
3.2.2. The result of Au@CD-MOF against L. monocytogenes
Very few L. monocytogenes survived after a 12 h treatment with Au@CD-MOF (). When the treatment time was extended to 24 h, there were no bacteria left on the plate. Furthermore, the bacterial time-inactivate profile of L. monocytogenes clearly demonstrated a gradual reduction in bacterial count over time (). At a treatment time of 36 h, all the bacteria were inactivated by Au@CD-MOF. showed the specific inactivated bacterial count in the time intervals. At treatment times of 1 h and 3 h, the inactivated bacterial count were 0.3 log and 1.1 log, respectively. With a treatment time of 6 h, 12 h, 24 h and 36 h, the inactivated bacterial count reached 4.7 log, 3.5 log, 4.4 log and 5.5 log, respectively. Above results indicated Au@CD-MOF had a notable capability in inhibiting the growth of L. monocytogenes. Its antibacterial ability against L. monocytogenes was superior to caffeine-loaded AuNPs (Caff-AuNPs). The growth of cells from the 10th stable passage of L. monocytogenes cultures was inhibited at 1024 μg/mL of Caff-AuNPs. And L. monocytogenes exhibited slight resistance even with 2048 μg/mL of Caff-AuNPs (Khan et al., Citation2021) while Au@CD-MOF inactivated 5.46 log bacterial count with 300 μg/mL. Furthermore, Osaili et al., found that the minimum bactericidal concentration of ZnO-nanoparticles against L. monocytogenes was 350 μg/mL which also higher than 300 μg/mL of Au@CD-MOF (Osaili et al., Citation2019).
Figure 3. Optical images of agar plates characteristic of antibacterial activities of Au@CD-MOF against L. monocytogenes under 12 h and 24 h and bacterial solution of the control group was prediluted 103 times (a); Bacterial time-inactivate profiles of Au@CD-MOF against L. monocytogenes (b); inactivated bacterial count of Au@CD-MOF against L. monocytogenes (c).
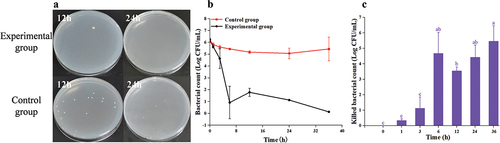
3.2.3. The result of Au@CD-MOF against E. coli O157:H7
There were numerous bacteria on the plate after a 12 h treatment (), but most of them were inactivated when the treatment time was prolonged to 24 h. The bacterial time-inactivate profile of E. coli O157:H7 clearly demonstrated a continuous decrease in bacterial count as the treatment time increased (). When the treatment time was 24 h, almost all bacteria were inactivated by Au@CD-MOF. showed that at a treatment time of 3 h, the inactivated bacterial count was 0.6 log. Furthermore, when the treatment time increased to 6 h, the inactivated bacterial count reached 1.57 log. When the treatment time was extended to 12 h, the inactivated bacterial count reached 3.3 log, which was twice as much as at 6 h. Additionally, almost all bacteria were inactivated when treatment time extended to 24 h. These results indicated that Au@CD-MOF exhibited excellent antibacterial properties against E. coli O157:H7, similar to AuNPs capped with a novel titanium (IV)-containing polyoxomolybdate cluster in the previous study. The AuNPs composite reported exhibited outstanding bactericidal efficiency, with a minimum inhibitory concentration of just 3.12 µm for the E. coli and no growth (minimum bactericidal concentration) was found when E. coli was treated with a concentration of 6.25 µm (Paesa et al., Citation2023).
Figure 4. Optical images of agar plates characteristic of antibacterial activities of Au@CD-MOF against E. coli O157:H7 under 12 h and 24 h and bacterial solution of the control group was prediluted 103 times (a); Bacterial time-inactivate profiles of Au@CD-MOF against E. coli O157:H7 (b); inactivated bacterial count of Au@CD-MOF against E. coli O157:H7 (c).
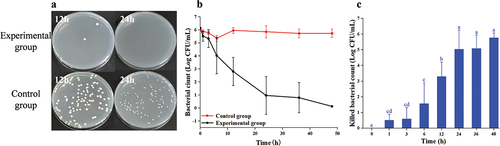
3.2.4. The result of Au@CD-MOF against P. aeruginosa PAO1
When the treatment time was 12 h, there were still numerous bacteria alive on the plate (). However, when the treatment time was extended to 24 h, there were hardly any living bacteria left on the plate. depicted the time-inactivate profile of P. aeruginosa PAO1, illustrating a consistent decline in bacterial count with increasing treatment duration. And when the treatment time was 36 h, almost all bacteria were inactivated by Au@CD-MOF. At a treatment time of 3 h, 1.15 log P. aeruginosa PAO1 were inactivated by Au@CD-MOF (). Furthermore, at treatment times of 6 h and 12 h, 1.63 log and 3.12 log bacteria were inactivated, respectively. These results indicated that Au@CD-MOF exhibited excellent antibacterial properties against P. aeruginosa PAO1 which was superior to a previous study. Hashem et al. reported that colloidal gold nanoparticles were prepared by the chemical reduction of gold ions in the presence of chitosan which showed a maximum inhibitory impact against P. aeruginosa PAO1 at 500 µg/mL (Hashem et al., Citation2022). This concentration was much higher than 300 µg/mL of Au@CD-MOF.
Figure 5. Optical images of agar plates characteristic of antibacterial activities of Au@CD-MOF against P. aeruginosa PAO1 under 12 h and 24 h and bacterial solution of the control group was prediluted 103 times (a); Bacterial time-inactivate profiles of Au@CD-MOF against P. aeruginosa PAO1 (b); inactivated bacterial count of Au@CD-MOF against P. aeruginosa PAO1 (c).
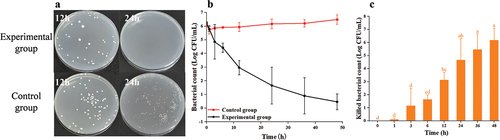
3.2.5. The result of Au@CD-MOF against S. typhimurium
The bacterial counts of the experimental groups were lower than those of the control groups (). Furthermore, compared to a treatment time of 12 h, the bacterial count significantly decreased when the treatment time was extended to 24 h. The time-inactivate profile of S. typhimurium () demonstrated a rapid decrease in bacterial counts for treatment duration of up to 36 h. However, when the treatment time exceeded 36 h, the bacterial counts showed a slower change. At a treatment time of 3 h resulted in a 0.43 log reduction in bacterial count (). Additionally, at treatment times of 12 h and 36 h, 1.91 log and 4.75 log reductions of S. typhimurium were observed with Au@CD-MOF, respectively. These results indicated that Au@CD-MOF exhibited excellent antibacterial properties against S. typhimurium.
Figure 6. Optical images of agar plates characteristic of antibacterial activities of Au@CD-MOF against S. typhimuriumunder 12 h and 24 h and bacterial solution of the control group was prediluted 103 times (a); Bacterial time-inactivate profiles of Au@CD-MOF against S. typhimuriumunder (b); inactivated bacterial count of Au@CD-MOF against S. typhimuriumunder (c).
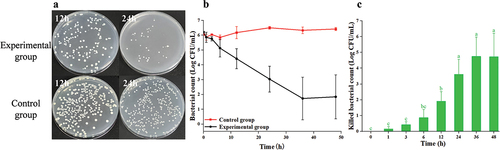
3.3. Antibacterial mechanism
3.3.1. SEM observation
The SEM image () of the control group of E. coli O157:H7 demonstrated intact cell membranes and normal morphology (F. Wei et al., Citation2021). However, the SEM image () of E. coli O157:H7 after treatment with Au@CD-MOF clearly showed pores and breakage on the surface of the bacteria (Gao et al., Citation2023). The result indicated the cell membrane of E. coli O157:H7 was damaged by Au@CD-MOF. Similarly, the S. aureus in the control group had intact and smooth cell membranes and a round and uniform morphology (). However, the bacteria in the experimental group suffered significant morphological deformations (), such as breakage. Additionally, the cell surfaces in the Au@CD-MOF treatment were covered with a large number of AuNPs, many of which adhered closely to the cell walls of S. aureus. Furthermore, obvious cracks could be seen in the cell walls (Ge et al., Citation2024). Therefore, the SEM observation results indicated that Au@CD-MOF damaged the cell membrane of S. aureus.
3.3.2. Cell membrane integrity
The cell membrane is crucial for bacteria as it acts as a barrier, preventing harmful substances from entering the cells. When the cell membrane is severely damaged by antibacterial substances, the DNA, RNA, and other macromolecules inside the cell can leak out (Hu et al., Citation2019). The fluorescence intensity () of the Au@CD-MOF treatment groups was significantly higher than that of the control group, indicating damage to the treated bacterial cells (Tang et al., Citation2017). Thus, the figure demonstrated that Au@CD-MOF severely damaged the cell membrane of E. coli O157:H7 while having only a minimal effect on S. aureus. Some people also reported that the control group barely damaged the bacterial membrane. In contrast, the groups with bacitracin directed gold nanoclusters treatment exhibited 26.6% of the bacteria with the damaged membrane (Wang et al., Citation2019).
Figure 8. PI fluorescence spectrum of E. coli O157:H7 and S. aureus which were treated with Au@CD-MOF for 24 h (a); Rh 123 fluorescence spectrum of E. coli O157:H7 and S. aureus which were treated with Au@CD-MOF for 24 h (b); optical density at 260 nm (c) and 280 nm (d) of E. coli O157:H7 and S. aureus which were treated with Au@CD-MOF for 24 h.
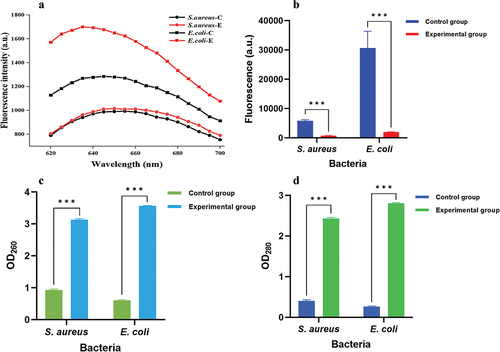
3.3.3. Cell membrane potential
The fluorescence value () of the control group of S. aureus was much higher than that of the experimental group, indicating that the cell membrane potential of the control group’s bacteria was high. This result demonstrated that the bacteria in the control group had intact cell membranes, while those in the experimental group had significantly damaged cell membranes (Y. Zhu et al., Citation2023). Additionally, E. coli O157:H7 showed similar results; the fluorescence value of the experimental group was much lower than that of the control group, indicating that the cell membrane potential of the experimental group’s bacteria was low. These results demonstrate that Au@CD-MOF can change the cell membrane potential and influence the bacteria’s normal activity.
3.3.4. Cell membrane permeability
The optical density (OD) value () at 260 nm represents the leakage of nucleic acid. Compared to the control group of S. aureus, the experimental group of S. aureus had a much higher OD260 value, indicating severe nucleic acid leakage caused by Au@CD-MOF. Similarly, the OD260 value of the experimental group of E. coli O157:H7 was much larger than that of the control group, indicating severe nucleic acid leakage (Azi et al., Citation2022; Qiao et al., Citation2020). Furthermore, the value of the OD at 280 nm represented the leakage of protein. Both the experimental groups of S. aureus and E. coli O157:H7 had much higher OD280 values compared to their respective control groups (), indicating severe protein leakage (P. Chen et al., Citation2023; X. Zhao et al., Citation2020). These results demonstrate that Au@CD-MOF can kill bacteria by causing severe leakage of nucleic acid and protein.
4. Conclusions
Au@CD-MOF was synthesized successfully and it exhibited excellent antibacterial ability against both Gram-positive such as S. aureus and L. monocytogenes and Gram-negative bacteria including E. coli O157:H7, P. aeruginosa PAO1, and S. typhimurium. Furthermore, Au@CD-MOF exerted its antibacterial effect by damaging the cell membrane, reducing cell membrane potential, and increasing cell membrane permeability. The disruption of bacterial cell membranes leads to the leakage of a large amount of nucleic acids and proteins, ultimately resulting in bacterial death. In conclusion, Au@CD-MOF holds promising prospects in the fields of antibacterial materials and food safety.
Author contributions
Meimei Guo: Software, Investigation, Formal analysis, Methodology and data curation, Writing-review & editing original draft;
Tahirou Sogore: Formal analysis and Writing-review & editing original draft;
Tian Ding: Formal analysis and Writing-review & editing original draft and Funding acquisition;
Mofei Shen: Conceptualization, Supervision, Writing-review & editing, Funding acquisition, Validation.
All authors discussed the results and commented on the manuscript.
Supplemental Material
Download MS Word (660.8 KB)Acknowledgments
This research is supported by the Starry Night Science Fund of Zhejiang University Shanghai Institute for Advanced Study, Grant No.SN-ZJU-SIAS-004 and China Postdoctoral Science Foundation (Grant No.2023M733112).
Disclosure statement
No potential conflict of interest was reported by the author(s).
Supplementary material
Supplemental data for this article can be accessed online at https://doi.org/10.1080/19476337.2024.2330670.
Additional information
Funding
References
- Atay, E., & Altan, A. (2023). Nanomaterial interfaces designed with different biorecognition elements for biosensing of key foodborne pathogens. Comprehensive Reviews in Food Science and Food Safety, 22(4), 3151–9. https://doi.org/10.1111/1541-4337.13179
- Azi, F., Li, Z., Xu, P., & Dong, M. (2022). Transcriptomic analysis reveals the antibacterial mechanism of phenolic compounds from kefir fermented soy whey against Escherichia coli 0157: H7 and Listeria monocytogenes. International Journal of Food Microbiology, 383, 109953. https://doi.org/10.1016/j.ijfoodmicro.2022.109953
- Borreby, C., Lillebæk, E. M. S., & Kallipolitis, B. H. (2023). Anti-infective activities of long-chain fatty acids against foodborne pathogens. FEMS Microbiology Reviews, 47(4). https://doi.org/10.1093/femsre/fuad037
- Chen, J., Guo, T., Ren, X., Yang, T., Zhang, K., Guo, Y., Chen, X., Gui, S., Wang, S., Li, Q., Peng, C., Zhang, J., & Wu, L. (2022). Efficient capture and stabilization of iodine via gas-solid reaction using cyclodextrin metal-organic frameworks. Carbohydrate Polymers, 291, 119507. https://doi.org/10.1016/j.carbpol.2022.119507
- Chen, P., Liu, Y., Li, C., Hua, S., Sun, C., & Huang, L. (2023). Antibacterial mechanism of vanillin against Escherichia coli O157: H7. Heliyon, 9(9), e19280. https://doi.org/10.1016/j.heliyon.2023.e19280
- Doghish, A. S., Hashem, A. H., Shehabeldine, A. M., Sallam, A.-A. M., El-Sayyad, G. S., & Salem, S. S. (2022). Nanocomposite based on gold nanoparticles and carboxymethyl cellulose: Synthesis, characterization, antimicrobial, and anticancer activities. Journal of Drug Delivery Science and Technology, 77, 103874. https://doi.org/10.1016/j.jddst.2022.103874
- Fu, Y., Yang, L., Zhang, J., Hu, J., Duan, G., Liu, X., Li, Y., & Gu, Z. (2021). Polydopamine antibacterial materials. Materials Horizons, 8(6), 1618–1633. https://doi.org/10.1039/d0mh01985b
- Gao, H., Sun, M., Duan, Y., Cai, Y., Dai, H., & Xu, T. (2023). Controllable synthesis of lignin nanoparticles with antibacterial activity and analysis of its antibacterial mechanism. International Journal of Biological Macromolecules, 246, 125596. https://doi.org/10.1016/j.ijbiomac.2023.125596
- Ge, Y.-M., Xue, Y., Zhao, X.-F., Liu, J.-Z., Xing, W.-C., Hu, S.-W., & Gao, H.-M. (2024). Antibacterial and antioxidant activities of a novel biosynthesized selenium nanoparticles using Rosa roxburghii extract and chitosan: Preparation, characterization, properties, and mechanisms. International Journal of Biological Macromolecules, 254, 127971. https://doi.org/10.1016/j.ijbiomac.2023.127971
- Han, D., Liu, X., & Wu, S. (2022). Metal organic framework-based antibacterial agents and their underlying mechanisms. Chemical Society Reviews, 51(16), 7138–7169. https://doi.org/10.1039/d2cs00460g
- Hashem, A. H., Shehabeldine, A. M., Ali, O. M., & Salem, S. S. (2022). Synthesis of chitosan-based gold nanoparticles: Antimicrobial and wound-healing activities. Polymers, 14(11), 2293. https://doi.org/10.3390/polym14112293
- He, K., Ding, Y. F., Zhao, Z., Liu, B., Nie, W., Luo, X., Yu, H. Z., Liu, J., & Wang, R. (2023). Cucurbit[7]uril‐mediated organ‐specific delivery of ultrasmall NIR‐II luminescent gold nanocarriers for therapy of acute kidney injury. Advanced Functional Materials, 34(6). https://doi.org/10.1002/adfm.202309949
- Hu, W., Li, C., Dai, J., Cui, H., & Lin, L. (2019). Antibacterial activity and mechanism of Litsea cubeba essential oil against methicillin-resistant Staphylococcus aureus (MRSA). Industrial Crops and Products, 130, 34–41. https://doi.org/10.1016/j.indcrop.2018.12.078
- Khan, F., Park, S.-K., Bamunuarachchi, N. I., Oh, D., & Kim, Y.-M. (2021). Caffeine-loaded gold nanoparticles: Antibiofilm and anti-persister activities against pathogenic bacteria. Applied Microbiology and Biotechnology, 105(9), 3717–3731. https://doi.org/10.1007/s00253-021-11300-3
- Kim, G., Xu, Y., Zhang, J., Sui, Z., & Corke, H. (2022). Antibacterial activity and multi-targeting mechanism of dehydrocorydaline from corydalis turtschaninovii Bess. Against Listeria monocytogenes. Frontiers in Microbiology, 12. https://doi.org/10.3389/fmicb.2021.799094
- Linklater, D. P., Baulin, V. A., Le Guével, X., Fleury, J. B., Hanssen, E., Nguyen, T. H. P., Juodkazis, S., Bryant, G., Crawford, R. J., Stoodley, P., & Ivanova, E. P. (2020). Antibacterial action of nanoparticles by lethal stretching of bacterial cell membranes. Advanced Materials, 32(52). https://doi.org/10.1002/adma.202005679
- Osaili, T. M., Albiss, B. A., Alromi, R. F., Olaimat, A., Al-Holy, M., Savvaidis, I., & Holley, R. (2019). Effects of metal oxide nanoparticles with plant extract on viability of foodborne pathogens. Journal of Food Safety, 39(5), e12681. https://doi.org/10.1111/jfs.12681
- Paesa, M., Almazán, F., Yus, C., Sebastián, V., Arruebo, M., Gandía, L. M., Reinoso, S., Pellejero, I., & Mendoza, G. (2023). Gold nanoparticles capped with a novel Titanium(IV)‐containing polyoxomolybdate cluster: Selective and enhanced bactericidal effect against Escherichia coli. Small, 20(6). https://doi.org/10.1002/smll.202305169
- Paparella, A., & Maggio, F. (2023). Detection and control of foodborne pathogens. Foods, 12(19), 3521. https://doi.org/10.3390/foods12193521
- Qiao, Z., Sun, H., Zhou, Q., Yi, L., Wang, X., Shan, Y., Yi, Y., Liu, B., Zhou, Y., & Lü, X. (2020). Characterization and antibacterial action mode of bacteriocin BMP32r and its application as antimicrobial agent for the therapy of multidrug-resistant bacterial infection. International Journal of Biological Macromolecules, 164, 845–854. https://doi.org/10.1016/j.ijbiomac.2020.07.192
- Salem, S. S. (2022a). Baker’s yeast-mediated silver nanoparticles: Characterisation and antimicrobial biogenic tool for suppressing pathogenic microbes. BioNanoScience, 12(4), 1220–1229. https://doi.org/10.1007/s12668-022-01026-5
- Salem, S. S. (2022b). Bio-fabrication of selenium nanoparticles using Baker’s yeast extract and its antimicrobial efficacy on food borne pathogens. Applied Biochemistry and Biotechnology, 194(5), 1898–1910. https://doi.org/10.1007/s12010-022-03809-8
- Salem, S. S. (2023). A mini review on green nanotechnology and its development in biological effects. Archives of Microbiology, 205(4). https://doi.org/10.1007/s00203-023-03467-2
- Salem, S. S., & Fouda, A. (2020). Green synthesis of metallic nanoparticles and their prospective biotechnological applications: An overview. Biological Trace Element Research, 199(1), 344–370. https://doi.org/10.1007/s12011-020-02138-3
- Shah, S., Shah, S. A., Faisal, S., Khan, A., Ullah, R., Ali, N., & Bilal, M. (2021). Engineering novel gold nanoparticles using Sageretia thea leaf extract and evaluation of their biological activities. Journal of Nanostructure in Chemistry, 12(1), 129–140. https://doi.org/10.1007/s40097-021-00407-8
- Shan, L., Qiao, Y., Ma, L., Zhang, X., Chen, C., Xu, X., Li, D., Qiu, S., Xue, X., Yu, Y., Guo, Y., Qian, K., & Wang, J. (2023). AuNPs/CNC nanocomposite with a “Dual dispersion” effect for LDI-TOF MS analysis of intact proteins in NSCLC serum exosomes. Advanced Science, 2307360. https://doi.org/10.1002/advs.202307360
- Shen, M., Liao, X., Xianyu, Y., Liu, D., & Ding, T. (2022). Polydimethylsiloxane membranes incorporating metal–organic frameworks for the sustained release of antibacterial agents. Acs Applied Materials & Interfaces, 14(10), 12662–12673. https://doi.org/10.1021/acsami.1c24921
- Smaldone, R. A., Forgan, R. S., Furukawa, H., Gassensmith, J. J., Slawin, A. M. Z., Yaghi, O. M., & Stoddart, J. F. (2010). Metal-organic frameworks from edible natural products. Angewandte Chemie International Edition, 49(46), 8630–8634. https://doi.org/10.1002/anie.201002343
- Soliman, M. K. Y., Salem, S. S., Abu-Elghait, M., & Azab, M. S. (2022). Biosynthesis of silver and gold nanoparticles and their efficacy towards antibacterial, antibiofilm, cytotoxicity, and antioxidant activities. Applied Biochemistry and Biotechnology, 195(2), 1158–1183. https://doi.org/10.1007/s12010-022-04199-7
- Tang, H., Chen, W., Dou, Z.-M., Chen, R., Hu, Y., Chen, W., & Chen, H. (2017). Antimicrobial effect of black pepper petroleum ether extract for the morphology of Listeria monocytogenes and salmonella typhimurium. Journal of Food Science and Technology, 54(7), 2067–2076. https://doi.org/10.1007/s13197-017-2644-2
- Wang, S., Wang, Y., Peng, Y., & Yang, X. (2019). Exploring the antibacteria performance of multicolor Ag, Au, and Cu nanoclusters. ACS Applied Materials & Interfaces, 11(8), 8461–8469. https://doi.org/10.1021/acsami.8b22143
- Wei, F., Cui, X., Wang, Z., Dong, C., Li, J., & Han, X. (2021). Recoverable peroxidase-like Fe3O4@MoS2-Ag nanozyme with enhanced antibacterial ability. Chemical Engineering Journal, 408, 408. https://doi.org/10.1016/j.cej.2020.127240
- Wei, H., Yang, C., Bi, F., Li, B., Xie, R., Yu, D., Fang, S., Hua, Z., Wang, Q., & Yang, G. (2024). Structure-controllable and mass-produced glycopolymersomes as a template of the carbohydrate@Ag nanobiohybrid with inherent antibacteria and biofilm eradication. Biomacromolecules, 25(1), 315–327. https://doi.org/10.1021/acs.biomac.3c01003
- Wei, Y., Han, S., Walker, D. A., Fuller, P. E., & Grzybowski, B. A. (2012). Nanoparticle core/shell architectures within MOF crystals synthesized by reaction diffusion. Angewandte Chemie International Edition, 51(30), 7435–7439. https://doi.org/10.1002/anie.201202549
- Zhang, B., Chen, H., Hu, Q., Jiang, L., Shen, Y., Zhao, D., & Zhou, Z. (2021). CelluMOFs: Green, facile, and flexible metal‐organic frameworks for versatile applications. Advanced Functional Materials, 31(43). https://doi.org/10.1002/adfm.202105395
- Zhang, B., Zang, Y., Mo, Q., Sun, L., Tu, M., Shu, D., Li, Y., Xue, F., Wu, G., & Zhao, X. (2023). Antibacterial activity and mechanism of slightly acidic electrolyzed water (SAEW) combined with ultraviolet light against Staphylococcus aureus. Lwt, 182, 114746. https://doi.org/10.1016/j.lwt.2023.114746
- Zhang, Y., Liu, X., Wang, Y., Jiang, P., & Quek, S. (2016). Antibacterial activity and mechanism of cinnamon essential oil against Escherichia coli and Staphylococcus aureus. Food Control, 59, 282–289. https://doi.org/10.1016/j.foodcont.2015.05.032
- Zhao, R.-N., Zhu, B.-W., Xu, Y., Yu, S.-F., Wang, W.-J., Liu, D.-H., & Hu, J.-N. (2023). Cyclodextrin-based metal-organic framework materials: Classifications, synthesis strategies and applications in variegated delivery systems. Carbohydrate Polymers, 319, 319. https://doi.org/10.1016/j.carbpol.2023.121198
- Zhao, X., Chen, L., Wu, J. E., He, Y., & Yang, H. (2020). Elucidating antimicrobial mechanism of nisin and grape seed extract against Listeria monocytogenes in broth and on shrimp through NMR-based metabolomics approach. International Journal of Food Microbiology, 319, 108494. https://doi.org/10.1016/j.ijfoodmicro.2019.108494
- Zhao, X., Zheng, M., Gao, X., Zhang, J., Wang, E., & Gao, Z. (2021). The application of MOFs-based materials for antibacterials adsorption. Coordination Chemistry Reviews, 440, 440. https://doi.org/10.1016/j.ccr.2021.213970
- Zhu, A., Ali, S., Jiao, T., Wang, Z., Ouyang, Q., & Chen, Q. (2023). Advances in surface‐enhanced raman spectroscopy technology for detection of foodborne pathogens. Comprehensive Reviews in Food Science and Food Safety, 22(3), 1466–1494. https://doi.org/10.1111/1541-4337.13118
- Zhu, Y., Xu, H., Cui, D., Zhou, R., Wang, Y., Soni, A., Brightwell, G., Zhuang, J., Ma, R., & Jiao, Z. (2023). A novel antibacterial mechanism of atmospheric cold plasma against staphylococcus aureus through degradation of cellular staphyloxanthin. Innovative Food Science & Emerging Technologies, 90, 103496. https://doi.org/10.1016/j.ifset.2023.103496