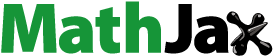
ABSTRACT
Triticale (× Triticosecale Wittmack), an edible hybrid of rye and wheat, is known for its superior agronomic traits. This study investigates the patterns of 5-n-alk(en)ylresorcinols (AR), phenolic lipids with diverse biological activities, in fresh and dried triticale across developmental stages. Total AR (TAR) content and LC-MS and GC-MS analyses were performed for profiling extracts obtained by solvent-assisted and supercritical fluid extraction methods. All triticale plant parts accumulated ARs, revealing significant distinctions in content and profiles between fresh and dry basis. CO2-extracted dry spikes at late stages and solvent-extracted fresh and dry leaves at the earliest stage exhibited the highest TAR content. Moreover, triticale kernels showcased a broader AR homolog diversity. Semi-quantitative AR levels varied based on plant parts and ontogeny, suggesting constitutive roles during growth. These insights into temporal and spatial distribution offer valuable information for determining optimal harvesting times, quality, and material condition for future breeding studies in triticale.
Introduction
5-n-alk(en)ylresorcinols (AR) represent specialized metabolites categorized within the family of non-isoprenoid phenolic lipids, characterized by a saturated or unsaturated carbon side chain attached to a resorcinol moiety at C5 (Ross, Citation2019). While these compounds occur naturally across various organisms, their prevalence is notably observed in plants (Kozubek & Tyman, Citation1999). Poaceae plants, including wheat, rye, and triticale, notably harbor high levels of AR primarily within kernel layers such as the outer and inner pericarp (husk, hyaline, and aleurone layers) (Landberg et al., Citation2008). Consequently, these plants have served as primary sources for characterizing a wide spectrum of AR homologs due to their abundant production and diversification of these compounds (Kozubek & Tyman, Citation1999; Mcgoverin et al., Citation2011). Although AR presence has been recorded in Fabaceae, Annacardiaceae, and Asteraceae plants, it occurs to a lesser extent compared to Poaceae species (Barrero et al., Citation1994; Kienzle et al., Citation2014; Melliou et al., Citation2003). Furthermore, AR are not exclusively confined to grains; varying amounts have been detected in other plant parts, such as leaves and stems of Vicia faba and roots of rice plants (Grayer & Kokubun, Citation2001; Zhao et al., Citation2019).
Recognized for their amphiphilic nature, AR possess distinctive properties – the 1,3-dihydroxylated aromatic ring contributing hydrophilic characteristics while the aliphatic chain confers lipophilicity (Sampietro et al., Citation2013a). As a result, AR display potential interactions with diverse biomolecules within biological systems (Shah et al., Citation2020). Previous studies have highlighted their role in providing antioxidant protection to cellular components against oxidative damage (Korycińska et al., Citation2009; Sapirstein, Citation2016; Zhou & Elias, Citation2013). Additionally, plants synthesize AR as a defense mechanism against both biotic and abiotic stressors (Sampietro et al., Citation2013a). The substantial accumulation of AR in Poaceae kernels contributes to pathogen resistance, exhibiting significant antimicrobial activity against pathogenic bacteria and fungi in vitro (Marentes-Culma et al., Citation2019; Miché et al., Citation2003). Moreover, these compounds can reorganize to form lipid layers on kernel surfaces, preventing rapid dehydration and safeguarding the plant against water stress (Wang et al., Citation2019).
Triticale (× Triticosecale Wittmack), a hybrid of wheat and rye, demonstrates superior agronomic traits compared to its parent species, particularly rapid adaptation to acidic soils (Cooper et al., Citation2009). Traditionally used as cattle feed due to its nutritious grains (Peña, Citation2004), triticale has gained attention for human consumption owing to its abundance in nutrients and phytochemicals that confer health benefits (Mcgoverin et al., Citation2011). Consequently, production and consumption of triticale kernels have surged, showing an over 3.7% increase since the 1980s (Arent & Zannini, Citation2013). AR are among the beneficial compounds contributing to the health properties of triticale, with AR content in grains averaging 350–650 μg/g dry matter (Ross, Citation2019).
While certain specialized metabolites in plants exhibit regulated production influenced by temporal and spatial factors (Isah, Citation2019), thereby concentrating in specific organs and developmental stages, others persist throughout the entire life cycle of the plant (Hussain et al., Citation2018). Despite this, understanding the distribution of AR-like metabolites remains challenging, as there is a scarcity of information concerning their levels in different plant parts across the life cycle of producer plants. In a previous study, we elucidated the semi-quantitative levels of AR across various developmental stages and tissues of triticale, revealing a responsive pattern associated with AR diversification depending on soil properties, particularly acidic pH (<6) and organic matter content (Marentes-Culma & Coy-Barrera, Citation2022). Moreover, the amphiphilic nature of AR can significantly influence the extraction process (Francisco et al., Citation2005; Rebolleda et al., Citation2013), particularly when dealing with removals from fresh or dried plant materials. In this context, extracting from fresh materials, which contain higher water content, leads to the partitioning of AR between the aqueous phase (due to the hydrophilic part) and the non-aqueous phase (due to the hydrophobic part), bearing similarity to the intracellular partitioning of endogenous amphiphilic substances between aqueous and lipid phases during dehydration/rehydration in desiccation-tolerant organ/organisms (Golovina et al., Citation1998). Conversely, dried plant materials, characterized by lower water content, can facilitate a more efficient partitioning of ARs into the non-aqueous phase of the extraction solvent. Additionally, they may intensify nonpolar interactions with the plant matrix, potentially impeding the removal process during extraction. This step, crucial in the preparation of plant material for extracting phytochemicals, involves several drying methods (Krakowska-Sieprawska et al., Citation2022). Consequently, the presence or absence of moisture can impact the solubility of these molecules in the extraction solvent, potentially leading to variations in extraction efficiency or interference in the extraction process. Hence, understanding such variations in the AR extraction between fresh and dried materials is crucial to discern partition-dependent extraction patterns for future studies. Additionally, there is a lack of a comprehensive comparative analysis that could shed light on the spatial and temporal patterns of occurrence and accumulation of these specialized metabolites, especially in the context of extractions from both fresh and dried triticale.
In this context, this study aims to address the above-mentioned gaps by quantifying the total AR content and analyzing the LC-MS-derived AR profiles across various triticale plant parts (including leaves, roots, spikes, and kernels) at different developmental stages from dry and fresh plant materials. This holistic analysis constitutes the main contribution and novelty of this study since it seeks to unveil, for the first time, the occurrence and dynamics of these specialized metabolites in dry and fresh triticale, thereby establishing the temporal and spatial distribution features of AR. These insights are anticipated to enhance our understanding of the chemical and biological characteristics of this hybrid plant, focusing on AR content and profiles.
Materials and methods
Plant material and developmental stage
Triticale seeds were sourced from the experimental crop of the “Obonuco Triticale 98” parental line at the Nueva Granada Military University Campus. The climate at this location is classified as subtropical highland with uniform rainfall (Cfb by Köppen and Geiger system), featuring an altitude of 2560 masl, an average temperature of 14.1 ± 6.9°C, and an annual precipitation of 1493 mm. The “Obonuco Triticale 98” line, inheriting rusticity from rye, is well-suited for cultivation in nutrient-poor soils with the occurrence of drought and frost seasons, characteristic of the Colombian Andean highlands (altitude: 2400–3000 masl). Collected triticale seeds were sown in a combining soil comprising silty loam soil with husk rice and peat in a 1:1:1 ratio, based on the soil effect of a previous study (Marentes-Culma & Coy-Barrera, Citation2022). Additionally, the plants received irrigation with a modified Hoagland solution to supplement their nutrition before the commencement of experiments. Subsequently, they were placed under greenhouse conditions, maintaining a temperature of 21 ± 9°C, 60 ± 20% relative humidity (RH), and a 12/12 light/dark photoperiod throughout the experiment. Six developmental stages were chosen based on the BBCH (biologische bundesanstalt, bundessortenamt, und chemische industrie) scale (Hess et al., Citation1997; Witzenberger et al., Citation1989). Furthermore, four plant parts – leaves, roots, spikes, and kernels – were selected for analysis based on their developmental stage-dependent biological relevance (as presented in ). Three biological replicates were collected per plant part and per developmental stage.
Table 1. Developmental stages along life cycle of triticale and test plant parts.
Preparation of raw extracts
Test triticale plants were systematically collected in triplicate and subsequently segregated into distinct plant parts based on the specified time intervals and developmental conditions, as outlined in . The collected plants were further processed under two post-harvesting conditions: fresh weight (immediately processed, denoted as FW) and dry weight (air-dried for 72 h at 60°C, denoted as DW). Both fresh and dry materials underwent overnight solvent-assisted (OSA) extraction, utilizing acetone as the extracting solvent (1:10 w/v ratio between plant material (g) and solvent (mL). The resulting mixtures were then filtered and concentrated under reduced pressure to obtain the AR-containing raw extract. On the contrary, supercritical fluid extraction was employed on DW-derived plant materials using an SFT-110 system (Supercritical Fluid Technologies, Inc, Newark, DE, USA), which comprised an SFT-10 high-pressure pump, restrictor valve, one extraction vessel, and one collector vessel. The DW material was introduced into the extraction vessel, and CO2 was pumped at a rate of 10.00 mL/min. Two extraction conditions were assessed, varying pressure/temperature parameters (4000 psi/50°C and 6000 psi/60 °C). These extraction conditions were carried out over three 15-min cycles at 100% CO2 flow.
Determination of total AR (TAR) content
All obtained extracts (n = 204) underwent analysis through a 96-well plate microcolorimetric method, employing Fast Blue RR (FBRR), as previously reported (Sampietro et al., Citation2013b) with some modifications. In brief, each AR extract was individually resuspended in methanol to prepare extract solutions (5 mg/mL). A FBRR working solution (prepared in a 1:5 ratio using methanol from a FBRR stock solution at 0.5 mg/mL) was also prepared. Each well of a 96-well microplate received 1% K2CO3 (20 µL), methanolic AR extract solution (30 µL), and FBRR working solution (180 µL). After 20 min, absorbance at 480 nm was measured using an Eliza EZ READ 800 microplate reader (Biochrom, Cambridge, UK). A control, substituting AR extract solution with methanol (30 µL), was also measured. All measurements were conducted in triplicate, and data were interpolated using a standard curve of olivetol. TAR content was expressed as µg total AR equivalent to Olivetol per g dried Material (µg OE/g DM).
DPPH· radical scavenging capacity
The antioxidant capacity of AR-extracts was evaluated using a microscale 2,2-diphenyl-1-picrylhydrazyl radical (DPPH·) assay (Buitrago et al., Citation2019). Initially, a DPPH· working solution was prepared at a 1:6 ratio with ethanol from a DPPH· stock solution at 100 mM. Increasing volumes of AR extract solutions (1–15 µL) were added to each well of the 96-well microplate. To adjust extract concentrations, 96% ethanol was added to complete 25 µL per well. Subsequently, DPPH· working solution (175 µL) was added to each well, and the plate was incubated in darkness for 1 h at room temperature. Each extract concentration was measured in triplicate. A control comprising DPPH· (175 µL) and ethanol (25 µL) was also prepared. Absorbance was measured at 515 nm for each well using an Eliza EZ READ 800 microplate reader (Biochrom, Cambridge, UK). Gallic acid served as the reference. The radical percentage of DPPH· scavenging was calculated as follows:
%DPPH· scavenging for each extract concentration was used to construct dose–response curves (% scavenging vs. decadic logarithm concentration) in triplicate using GraphPad Prism 8.0 software. The resulting dose–response curves were employed to calculate the half-maximal inhibition concentration (IC50) through non-linear regression.
Total phenolic content (TPC)
TPC was determined via a microscale Folin-Ciocalteu method (Buitrago et al., Citation2019). In brief, AR extract solution (20 µL) and 10% Folin-Ciocalteu reagent (40 µL) were added to each well of the 96-well microplate. After 3 min, 7.35% Na2CO3 (150 µL) was added, and the resulting mixture was incubated in darkness for 2 h at room temperature. Absorbance was then measured at 765 nm using an Eliza EZ READ 800 microplate reader (Biochrom, Cambridge, UK). These measurements were performed in triplicate, and data were interpolated using a standard curve of gallic acid. TPC was expressed as µg total phenolics equivalent to gallic acid per g dry material (µg GAE/g DM).
UltraHigh-performance liquid chromatography-mass spectrometry (UHPLC-MS) analysis
Extract solutions derived from fresh (FW) and dried (DW) triticale were prepared at 5 mg/mL in methanol (high-performance liquid chromatography (HPLC) grade) for chromatographic analysis. Employing a Shimadzu Prominence System (Shimadzu, Columbia, MD, USA) equipped with a Diode Array Detector (DAD) and coupled to a Shimadzu LCMS2020 mass spectrometer through electrospray ionization (ESI), all samples were subjected to ultrahigh-performance liquid chromatography (UHPLC). Separation of alkylresorcinols (AR) was achieved using a reverse-phase Kinetex® column (C18, 150 × 4.6 mm, and 2.6 µg particle size) and solvent mixtures (i.e. MeOH:H2O (8:2) (solvent A) and isopropanol:MeOH (3:7) (solvent B) with a gradient elution (0–2 min 0% B, 27–32 min 100% B, and 34–37 min 0% B) at 0.6 mL/min. The injection involved 10 µL of the extract solution. Detection was performed at 275 nm. ESI was operated in negative ion mode (scan 100–2000 m/z), with a desolvation line temperature at 250°C, nitrogen as nebulizer gas at 1.5 L/min, drying gas at 8 L/min, and detector voltage at 1.4 kV.
Gas chromatography-mass spectrometry (GC-MS) analysis
Exclusive to extracts derived from kernels using the overnight solvent-assisted (OSA) extraction method, gas chromatography-mass spectrometry (GC-MS) analysis was conducted. The working DW kernel-derived AR extract solution (200 µL, 5 mg/mL in chloroform) was dried into an insert within a chromatography vial. Following this, N-methyl-N-trimethylsilyltrifluoroacetamide (MSTFA) activated II® (Sigma, St. Louis, MO, USA) (20 µL) was added, and the reaction mixture was incubated at 60°C for 60 min. The resulting raw derivatized mixture underwent analysis using a Trace 1300 LT gas chromatograph (Thermo Scientific, Waltham, MA, USA) coupled to a Thermo ISQ Mass Spectrometer, equipped with electron impact ionization and a single quadrupole analyzer. A sample (1 µL) was injected in splitless mode, and the injection port temperature was configured at 300°C. Analytes were separated using a high-temperature capillary column (60 m × 0.25 mm ID × 0.25 µm Rxi® 5 Sil MS, Restek, State College, PA, USA). Helium (99.999%) was used as the carrier gas at 1.5 mL/min. The temperature program was initially held at 100°C for 1 min, then increased from 100°C to 250°C at 15°C/min, and held at 250°C for 5 min. Finally, the program was increased at 10°C/min until 310°C and maintained at 310°C for 30 min. ARs detected and separated in the total ion chromatogram (TIC) were identified using diagnostic fragments observed in the electron impact-derived mass spectra. All ARs exhibited the typical fragmentation patterns of silylated AR, indicating the homologous nature of all identified compounds, including the di-O-silylated 1,3-dihydroxytropylium cation ([(TMSO)2C6H4CH2]+, m/z 268), as well as the AR typical fragments at m/z 281, 310, and 341 (Marentes-Culma et al., Citation2019; Żarnowski et al., Citation2002). Additionally, the respective side-chain length and other substituents of each AR were deduced by the mass difference between the diagnostic fragment (i.e. m/z 268) and the observed molecular ion (M+).
Data analysis
The Shapiro–Wilks test was employed to examine the normal distribution of quantitative data. Subsequently, an analysis of variance (ANOVA) and multiple comparison post-hoc Tukey test were used to determine significant differences (p < 0.05) between test variables, performed using R project software version 3.0.2 (Freeware) (R Foundation, Vienna, Austria). Additionally, a feature intensity table (FIT) was constructed from a dataset comprising fresh (FW) and dried (DW)-derived plant samples (n = 102) and UHPLC-DAD-ESI-MS-detected AR features. These features were filtered from the entire dataset through the combined diagnostic analysis of UV and MS spectra (i.e. λmax = 275 nm and [M – H]− and [2 M – H]− adducts, respectively) (Knödler et al., Citation2008; Marentes-Culma et al., Citation2019). The web-based tool suite Metaboanalyst 5.0 (Chong et al., Citation2019) was then employed for a comprehensive analysis of the filtered, pre-processed FIT. Heatmaps were generated to illustrate overall AR distribution. ANOVA Simultaneous Component Analysis (ASCA) and Multivariate Empirical Bayes Analysis of Variance (MEBA) were also performed to observe AR variation-based patterns along developmental stages per plant part of triticale.
Results
Six developmental stages, namely the first leaf, two leaves, flag leaf, inflorescence, late-milky grain, and harvest, were chosen based on physiological and morphological changes in triticale’s ontogeny (Lancashire et al., Citation1991). To cover two post-harvesting plant material conditions (dry and fresh), plant material, including leaves, roots, spikes, and kernels, was harvested in triplicate at each developmental stage. The harvested materials were divided into two portions: the first portion was processed in fresh triticale (FW) samples using a conventional overnight solvent-assisted (OSA) extraction method with acetone; the second portion was processed with dried triticale (DW) samples using both OSA extraction and supercritical fluid extraction (SCF) at two independent pressure/temperature (P/T) conditions (4000 psi/50°C and 6000 psi/60 °C). Consequently, a total of 204 AR extracts were obtained, comprising 51 from FW and 153 from DW plant materials. Among the DW-derived extracts, 51 were from overnight extraction, 51 from SCF extraction at 4000 psi and 50°C, and 51 from SCF extraction at 6000 psi and 60°C.
Levels of TAR throughout life cycle of triticale
TAR content was measured for the 204 samples, considering two post-harvesting plant material conditions (i.e. fresh and dried), four plant parts, and six developmental stages of triticale in triplicate (). The resulting TAR values demonstrated varying AR content among plant parts and developmental stages. For instance, leaves at the first stage (LF1) exhibited the highest TAR content among all tested samples in both FW- and DW-derived extracts (489.0 and 375.9 µg OE/g DM, respectively). FW roots at the third stage (RF3) showed higher AR content (392.9 µg OE/g DM) than DW roots at the second stage (RD2) (291.8 µg Olivetol equivalent/g DE). Additionally, spikes at the sixth stage and kernels at the fifth stage showed elevated AR levels in both processing conditions. TAR contents from cultivated plants were notably higher than those of parent kernels measured at the beginning of the experiment (5.9 µg OE/g).
Table 2. Total AR (TAR) content, total phenolic content (TPC), and DPPH· radical-scavenging capacity of triticale samples at different developmental stages and plant parts from different processed plant materials (i.e. fresh (FW) and dry (DW) processing) and extraction methods (i.e. overnight solvent assisted (OSA) and supercritical fluid (SCF) extraction).
However, differences were observed between AR levels in FW and DW samples extracted by the OSA procedure. For instance, TAR content from leaf samples exhibited a descending trend throughout developmental stages in both processing conditions, with TAR content of FW leaves between the first and fifth stages significantly higher than that of DW leaves. The AR levels at the sixth stage did not show differences between both processing conditions (Figure S1a). Regarding root samples, AR levels for FW and DW roots exhibited a similar downward trend, but an initial increase was noted (Figure S1b). The maximum AR level of this increase was observed at the second stage in DW roots, whereas FW roots peaked at the third stage. In contrast, AR levels of both processing conditions in spikes behaved differently, with FW spikes exhibiting an initial decrease and subsequent increase, while DW spikes displayed a constant rise (Figure S1c). Finally, FW kernels showed an enhanced AR level between the fifth and sixth stages, but the TAR content of DW kernels at both stages maintained similar values (Figure S1c).
Furthermore, TAR contents for SCF-extracted DW samples considerably decreased for both P/T conditions compared to extracts obtained by OSA extraction (). For example, spike samples at the fifth stage (S5) presented the highest TAR content at both P/T conditions (1.1 µg OE/g DM extracted at 4000 psi/50°C and 1.1 µg OE/g DM extracted at 6000 psi/60 °C). Generally, the two test P/T conditions did not exhibit significant differences, except for some specific samples (L2, L3, R1, and R2). These outliers included a leaf at the second stage extracted at 6000 psi/60°C and a leaf at the third stage extracted at 4000 psi/50°C (Figure S2a). In addition, AR contents of root extracts at the first and second stages obtained at 6000 psi/60°C were higher than those obtained at 4000 psi/50°C (Figure S2b).
Total phenolic content throughout life cycle of triticale
Total Phenolic Content (TPC) was assessed for OSA-extracted FW and DW samples (n = 102) using the Folin-Ciocalteu microscaled method (Buitrago et al., Citation2019) (). Initially, the TPC of parent kernels was measured at 216.6 µg GAE/g. FW leaves exhibited the highest TPC at the first stage (7844.7 µg GAE/g DM and 2264.4 µg GAE/g in FW and DW samples, respectively), followed by a continuous decrease along developmental stages. DW leaves showed a similar trend, with a slight increase observed at the fifth stage (Figure S3a). Furthermore, the phenolic contents of FW leaves at all developmental stages were statistically higher than those of DW leaves, except for samples at the fifth stage. In the case of roots, TPC of DW roots reached the maximum value at the first stage (4600.5 µg GAE/g DM) and gradually decreased along stages. Conversely, FW roots exhibited an initial increasing trend, reaching the highest point at the second stage (135.6 µg GAE/g DM), followed by a decrease (Figure S3b). Spikes demonstrated significantly different TPC behaviors between FW and DW; FW spikes peaked at the fourth stage (1490.2 µg GAE/g DM), while DW spikes exhibited the highest content at the sixth stage (897.7 µg GAE/g DM) (Figure S3c). Finally, the TPC values of FW kernels at the fifth and sixth stages were significantly higher than those of DW kernels. However, TPC of FW kernels increased from the fifth to the sixth stage, whereas TPC of DW kernels decreased during the same stages (Figure S3c).
Radical-scavenging capacity throughout life cycle of triticale
The DPPH· assay was employed to assess the free radical-scavenging capacity of OSA-extracted FW and DW samples (n = 102). The resulting IC50 values of test samples were higher than that of trolox (IC50 = 140.3 µg/mL), with some exceeding 1000 µg/mL, while parent kernels showed an IC50 = 179.7 µg/mL (). FW leaves at the first and second stages, LF1 and LF2, exhibited the lowest IC50 values (237.8 and 229.9 µg/mL, respectively), along with FW kernels at the fifth stage (IC50 = 255.4 µg/mL).
UHPLC-MS-based AR analysis
Extracts from OSA-extracted FW and DW samples (n = 102) were analyzed using HPLC-DAD-ESI-MS with a chromatographic method developed specifically for AR analysis (Marentes-Culma et al., Citation2019). Due to the phenolic nature of ARs, negative mode was then employed. This analysis allowed the detection, separation, and annotation of different AR-type compounds (Figure S4) based on diagnostic MS data (details in section 2.7). The distribution of AR homologs regarding lateral-chain length and saturation levels was visualized through heatmap plots, comparing the normalized relative abundance of each AR homolog (). The initial heatmap illustrated the global distribution of AR homologs based on differences between the post-harvesting plant material conditions (FW and DW) (). This heatmap revealed that homologs C17 (saturated side chain), C17:1 (unsaturated side chain) and C21 (saturated side chain) were more abundant in FW samples, whereas homologs C19, C21:1, C23, and C25 were generally abundant in DW samples.
Figure 1. (a) heatmap of the relative abundance of those AR detected in all samples separating into fresh (FW) and dry (DW) triticale samples. (b) heatmaps of the relative abundance of those AR detected in FW and DW-derived extracts per plant part.
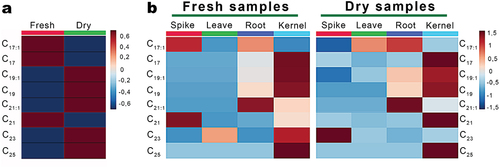
To comprehensively assess the distribution of AR homologs in triticale, two additional heatmaps were constructed to compare the abundance of AR homologs among post-harvesting conditions per plant part, irrespective of developmental stages (). The relative abundances of all AR homologs in FW samples were higher in kernels (excepting C17:1), with C17:1 and C21 emerging as the most representative in spikes. Furthermore, C21:1 exhibited a higher presence in roots, although C17:1 and C19 displayed weaker associations, while the elevated abundance of C23 was primarily linked to leaves and kernels. For DW samples, most AR homologs were notably abundant in kernels, with C23 exhibiting enhanced levels in spikes. Additionally, C21:1 and C17:1 were prevalent but with lower abundance of C19:1 and C19 in roots, while C17:1 was also abundant in leaves.
ANOVA Simultaneous Component Analysis (ASCA) models were employed to discern interaction patterns between two factors – post-harvesting condition and developmental stage per plant part (). All resulting plots exhibited distinct scores, with percentage of variation explanation (% VE) exceeding 85%. Notably, AR variations between FW and DW leaves were more pronounced at the first and fifth stages (). Roots displayed greater variations at the second, third, and fourth stages (), whereas FW and DW spikes and kernels exhibited contrasting behavior at the tested developmental stages ().
Figure 2. ASCA models relating two factors, i.e. plant material processing prior extraction (fresh weight (FW) and dry weight (DW)) and test developmental stages (i.e. first leaf, two leaves, flag leaf, inflorescence, late-milky grain, and harvest) per plant part: (a) leaves, (b) roots, (c) spikes, and (d) kernels.
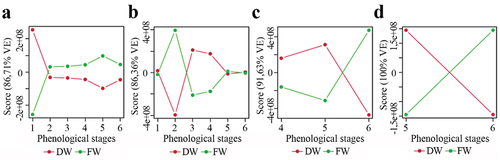
Multivariate Empirical Bayes Analysis of Variance (MEBA) was conducted to ascertain the influence of each detected AR homolog on the statistical discrimination of each post-harvesting condition throughout developmental stages per plant part. Figures S5-S8 present the resulting MEBA plots for all detected AR homologs per plant part, while consolidates MEBA plots for the most representative AR homologs. C17 from leaves and spikes, as well as C23 from spikes, exhibited significant abundance for DW samples (). Key AR homologs such as C17:1, C17, C19:1, and C23 were notably abundant in leaves, with the abundance variation of C17:1 between FW and DW samples being higher at the first stage, while C17 exhibited substantial changes at the second and fourth stages (). In roots, AR homologs C17:1, C17, C19:1, and C23 showed considerable changes between FW and DW samples, particularly at the second, third, and fourth stages (Figure S6), with C17:1 exhibiting marked variation at the second stage and C17 fluctuating predominantly at the third and fourth stages (). AR homologs C17:1, C17, C21, and C23 in spikes demonstrated several variations between FW and DW samples at all stages, especially for C23 (). Finally, AR homologs detected in kernels exhibited variable trends between the two processing conditions at the fifth and sixth developmental stages (Figure S8).
GC-MS-based analysis after TMSFA derivatization of kernel-derived extracts
To further elucidate the chemical composition of kernel-derived extracts and support AR identification, an additional GC-MS analysis was conducted. This analysis was exclusively performed for kernel-derived extracts, as the extraction method (i.e. SCF) and the silylation derivatization (i.e. TMSFA) conditions rendered these extracts suitable for analysis. In contrast, extracts from leaves, roots, and spikes, as well as those from OSA extraction, contained various interferents that significantly limited the derivatization procedure and a good profile recording (data not shown). The GC-MS-based chemical composition of kernel-derived extracts is summarized in . Variances were observed between extraction protocols (i.e. SCF versus OSA), as some OSA removed AR homologs remained undetected by GC-MS after SCF extraction (e.g. C17:1). Conversely, homologs like C15 and C21:1 were identified by GC-MS but solely in FW samples and at very low abundance. Notably, FW samples (KF5 and KF6) exhibited a higher number of AR homologs compared to DW samples (KD5 and KD6). Saturated homologs, including C17, C19, and C21, were more abundant and consistently present in all samples. In contrast, unsaturated AR homologs, characterized by shorter and longer side chains, exhibited low abundance in triticale kernels irrespective of the plant material condition (i.e. dry or fresh).
Table 3. Composition and total relative percentage of AR homologs in test kernel samples extracted by SCF and analyzed by GC-MS after silylation-based derivatization.
Discussion
Our findings suggest that ARs are compounds intricately involved in triticale’s physiology, as they were found across various plant parts and developmental stages. While previous studies predominantly focused on removing AR from kernels due to their high concentration of these compounds (Ross, Citation2019), our data reveal that certain plant parts, such as leaves, consistently produce AR throughout the entire life cycle of triticale. Specifically, the leaf sample at the first developmental stage (i.e. coleoptile appearance) exhibited the highest TAR content in the entire experiment. Seedlings have been identified as a plant part with elevated AR production, with demonstrated AR overproduction in this stage under the presence of Fusarium culmorum (Kozubek et al., Citation2001). In this context, AR has been linked to various ecological processes in plants, including defense against predators and/or microbes (Piasecka et al., Citation2015; Zaynab et al., Citation2018). Given that leaves are recognized as the primary source of resources for the plant due to their active photosynthetic machinery (Schultz et al., Citation2013), the biosynthesis of defensive plant metabolites is triggered to protect against phytophagous and/or pathogenic attacks attempting to seize or exploit these resources. AR are presumed to belong to the protective metabolite group and are rationalized to function as allelochemicals and phytoanticipins (Baerson et al., Citation2010). Notably, AR has been reported in wax layers on both the adaxial and abaxial sides of rye leaves, as well as within the leaf structure layers, playing protective roles as barriers (Ji & Jetter, Citation2008).
The comparison between fresh weight (FW) and dry weight (DW) triticale as the initial post-harvesting plant material condition did not reveal a discernible trend in TAR contents. In our experiment, TAR levels were significantly elevated in certain FW samples (i.e. LF1, RF3, and KF5), while others, particularly SD6, exhibited high TAR values among DW samples. This observation suggests that AR removal may depend on various physicochemical factors influenced by plant matrix characteristics (e.g. fibrous tissue, particle size, hardness, etc.) and AR properties (e.g. solubility, diffusion, etc.), which are accentuated or mitigated by the water content in the target plant material (Takeuchi et al., Citation2009). Moreover, AR are recognized as compounds with high thermal stability, demonstrated by their ability to withstand high temperatures, as evidenced by their detection in baked breads made from various Poaceae flours (Ross, Citation2019). In contrast, the TPC values were significantly higher in FW leaves and kernels, although TPC of DW roots and some spike samples surpassed those of FW-derived extracts. Some phenolic metabolites can undergo changes in plant material due to various external conditions (e.g. temperature, relative humidity, oxidative environment, etc.). Therefore, the decrease in TPC observed in DW samples can be rationalized by degradation-mediated transformation processes (Jones & Kinghorn, Citation2012). Additionally, a matrix effect justifies the observed differences in TPC. These technical considerations are crucial, as the removal of this compound type from plant sources can yield diverse information depending on the extraction process, plant material conditions, and the nature of both the plant matrix and target metabolites. Fresh material is therefore recommended for extracting AR and phenolics. On the other hand, the radical-scavenging capacity was notably low for most test samples, especially those derived from DW extracts. While AR has been documented as antioxidant compounds due to the presence of a meta-dihydroxybenzene moiety capable of accepting free radicals (Kozubek et al., Citation2001), our results suggest that compounds in triticale extracts do not exhibit a robust antioxidant capacity (IC50 >350 µg/mL). This could be attributed to the presence of non-active or low-active hydroxyl groups in AR and other phenolics, as previously reported (Ross, Citation2019).
Building on the prior information, we focused on the observed differences in TAR content values between Fresh Weight (FW) and Dry Weight (DW) samples for the subsequent metabolite profiling. Consequently, extracts obtained through OSA extraction were exclusively analyzed using ultra-high-performance liquid chromatography-mass spectrometry (UHPLC-MS). Evaluation of the AR-profiling data revealed distinctions between FW and DW samples. Heatmaps intuitively depicted the distribution of AR abundance for each post-harvesting plant material condition (i.e. fresh or dry) prior to extraction, highlighting a greater abundance of AR homologs in kernel samples. AR from Poaceae kernels has been extensively characterized, considering the inclusion of these grains in the human diet and their association with various health benefits (Landberg et al., Citation2008; Mcgoverin et al., Citation2011).
ASCA models provided insights into the relative behavior of AR composition in plant parts and developmental stages. Notably, substantial differences between FW and DW-derived extracts were observed, particularly in spikes and kernels. These variations might be attributed to a combined matrix-metabolite effect, given that FW samples were processed immediately, while DW samples underwent a 72-h drying period following established protocols for grain drying and storage (Schirmer et al., Citation2006). Based on the post-harvesting manipulation of DW material, we hypothesized that lipid layers, encompassing AR, might envelop the DW samples during the drying process to mitigate water losses in plants (Jenks et al., Citation1994). This phenomenon leads to alterations in the AR composition, primarily in spikes and kernels, resulting in distinctions between DW and FW extracts. Furthermore, this observed pattern could be substantiated by the resilience of Obonuco Triticale 98 to abiotic factors, particularly drought.
The diversity of AR is linked to the genetic and biochemical factors of producing plants, enabling the biosynthesis of different AR homologs. Specific chemotypes can be retained or maintained through various constitutive and/or induced events (Zhao et al., Citation2019). In this regard, MEBA models unveiled that the homologs C17:1, C17, and C23 were consistently the most recurrent and abundant compounds across all four tested plant parts. Saturated AR homologs, particularly C17 and C23, have been documented as the most prevalent AR in various plants, representing over 90% of the total AR in rye, wheat, and triticale (Ross, Citation2019). In the present study, AR homologs exhibited diverse behavior among and within plant parts, developmental stages, and FW/DW conditions. Some homologs displayed significant increases at early stages, such as the seedling stage, aligning with the reported defensive and protective roles of AR in plants against biotic/abiotic factors (Baerson et al., Citation2010; Ross, Citation2019). Actually, seedlings, described as an active phase of AR biosynthesis in rye, notably featured the presence of C17 (Magnucka et al., Citation2015). In contrast, reproductive plant parts exhibited a distinct AR pattern between developmental stages and even among FW and DW conditions. The substantial AR variation observed in FW kernels at stages 5–6 can be attributed to the differential accumulation of specific AR. For instance, at stage 6, C17:1, C17, C21, and C19 increased, while C21 and C23 appeared to be depleted. In spikes, C17:1 demonstrated an upregulating behavior from stage 5 to stage 6, while C23 exhibited downregulation. Concerning the FW/DW condition, the increase in AR abundance for DW-derived samples, particularly C17 and C23 in spikes, might be rationalized by a matrix effect, possibly associated with the protective role of AR against dehydration events in triticale plant materials (Jenks et al., Citation1994). In contrast, the AR upregulation for FW samples, such as C17 in leaves and C17 and C17:1 in roots, could be linked to AR biosynthesis as a constitutive mechanism for plant growth-related defense against pathogens (Sampietro et al., Citation2013a). These interpretations, elucidating the observed AR variations along stages, warrant further experiments for validation.
When comparing the GC-MS profiles of kernel-derived extracts, DW samples exhibited a higher total AR percentage than FW kernels at the fifth stage (96.02% vs. 79.61%), but this trend reversed at the sixth stage (85.11% vs. 98.40%). This influence was also evident in the number of AR compounds between both conditions, irrespective of the developmental stage. FW kernels displayed a higher number of AR (nine compounds), with variations in abundance between stages 5 and 6. In contrast, DW kernels showed a reduced number (four AR), suggesting a significant impact of the plant material condition (dry or fresh) on the extraction of AR-type compounds. This observation justifies the preference for fresh triticale kernels for extracting such compounds. A selectivity trend was noted, particularly in the extraction of saturated AR C17, C19, and C21, which were preferably extracted from DW kernels. This resulted in an abundance depletion of these saturated AR at stage 6 compared to their abundances at stage 5. These primary AR, including C23, were also observed in FW kernels, but the abundance distribution exhibited a contrary behavior between stages 5 and 6 compared to DW samples (Ross et al., Citation2003). In the case of other tested plant materials, UHPLC-MS emerged as the most favorable technique for obtaining enhanced AR profiles from a wide range of plant materials. This approach circumvents the limiting issues associated with GC-MS-based AR analysis, such as derivatization interferences, and provides an improved, broader analytical scope whether a suitable extraction and separation of AR are performed.
Finally, the comparison between the extraction methods, i.e. OSA and SCF, revealed significant differences in TAR contents and LC-MS-based AR profiles. OSA is the most frequently employed extraction method due to its ability to facilitate metabolite removal from the plant matrix (Moldoveanu & David, Citation2015). The principal feature of OSA is to minimize the degradation of thermolabile metabolites, making it a simple, cost-effective, and efficient method (Zhang et al., Citation2018). Nevertheless, SCF extraction is highly efficient, inert, non-toxic, and selective. However, SCF often requires support from a co-solvent vessel to enhance extraction yield of ARs (Athukorala et al., Citation2010). Supercritical CO2 (SC-CO2), the typical medium used in SCF extraction, generally provides lower AR yields compared to conventional organic solvent-assisted extractions (Rebolleda et al., Citation2013). In our experiment, the absence of such a vessel resulted in an inefficient AR extraction. Therefore, it is recommended for future experiments to optimize SCF-based AR extraction from triticale using co-solvents (e.g. ethanol), similar to experiments previously conducted on triticale bran, which utilized a two-step SC-CO2 extraction protocol (Athukorala et al., Citation2010).
Concluding remarks
The results of our study reveal the ubiquitous presence of AR across all plant parts and developmental stages of triticale, emphasizing their dynamic distribution throughout the plant’s life cycle. Importantly, the post-harvesting condition (fresh weight – FW, and dry weight – DW) of triticale plant materials significantly influences the levels of TAR content. It is noteworthy that these variations are distinct for specific plant parts and developmental stages, particularly in roots and leaves. This nuanced understanding of the impact of post-harvest conditions on AR levels is crucial for designing future studies, as it sheds light on the diverse trends that can emerge during AR extraction due to the interplay of extraction methods, plant matrix characteristics, and the nature of metabolites. In this context, our findings highlight triticale leaves as a significant source of AR throughout the entire life cycle, with the highest content observed at the first developmental stage. Additionally, triticale kernels emerge as the most promising reservoir for a wide variety of AR homologs at both stages (5 and 6), boasting eight main AR homologs, among which C17 and C23 stand out as the most abundant phenolic lipids. The semi-quantitative differences in AR levels further demonstrate a dependence on physiological and matrix effects, influencing both the content and profiles of the extracted AR. This performance can be attributed to constitutive mechanisms operating during plant growth, suggesting a role for AR in protective functions against biotic factors such as pathogens and abiotic stresses like drought and desiccation. The comprehensive insights provided by our study establish a valuable foundation for understanding the temporal and spatial distribution characteristics of AR in triticale. This information serves as a crucial baseline for optimizing harvesting times and conducting quality assessments. Furthermore, it contributes to the potential expansion of AR exploitation in future plant selection and breeding-based studies focused on triticale. The intricate interplay between extraction conditions and the plant’s physiology uncovered in this study adds depth to the existing knowledge, paving the way for more targeted and informed research in this field.
Supplemental Material
Download PDF (1.1 MB)Acknowledgments
Authors thank Universidad Militar Nueva Granada (UMNG) for the financial support.
Disclosure statement
No potential conflict of interest was reported by the author(s).
Data availability statement
All data generated or analyzed during this study are included in this published article. The datasets used and analyzed in this study can be found in Supplementary Material.
Supplementary material
Supplemental data for this article can be accessed online at https://doi.org/10.1080/19476337.2024.2354520
Additional information
Funding
References
- Arent, E., & Zannini, E. (2013). Cereal grains for the food and beverage industries (1st ed.). Woodhead publishing. https://doi.org/10.1533/9780857098924.201
- Athukorala, Y., Hosseinian, F. S., & Mazza, G. (2010). Extraction and fractionation of alkylresorcinols from triticale bran by two-step supercritical carbon dioxide. LWT - Food Science and Technology, 43(4), 660–11. https://doi.org/10.1016/j.lwt.2009.11.008
- Baerson, S. R., Schröder, J., Cook, D., Rimando, A. M., Pan, Z., Dayan, F. E., Noonan, B. P., & Duke, S. O. (2010). Alkylresorcinol biosynthesis in plants. Plant Signaling & Behavior, 5(10), 1286–1289. https://doi.org/10.4161/psb.5.10.13062
- Barrero, A., Cabrera, E., Rodriguez, I., & Planelles, F. (1994). Alkylresorcinols and isocoumarins from ononis pubescens. Phytochemistry, 35(2), 493–498. https://doi.org/10.1016/S0031-9422(00)94789-7
- Buitrago, D., Buitrago-Villanueva, I., Barbosa-Cornelio, R., & Coy-Barrera, E. (2019). Comparative examination of antioxidant capacity and fingerprinting of unfractionated extracts from different plant parts of quinoa (chenopodium quinoa) grown under greenhouse conditions. Antioxidants, 8(8), 238. https://doi.org/10.3390/antiox8080238
- Chong, J., Wishart, D. S., & Xia, J. (2019). Using MetaboAnalyst 4.0 for comprehensive and integrative metabolomics data analysis. Current Protocols in Bioinformatics, 68(1), e86. https://doi.org/10.1002/cpbi.86
- Cooper, K. V., Mergoum, M., Singh, P. K., & Pen, R. J. (2009). Triticale: A ‘“new”’ crop with old challenges. In M. J. Carena (Ed.), Cereals. Springer US. https://doi.org/10.1007/978-0-387-72297-9
- Francisco, J. D. C., Danielsson, B., Kozubek, A., & Dey, E. S. (2005). Application of supercritical carbon dioxide for the extraction of alkylresorcinols from rye bran. The Journal of Supercritical Fluids, 35(3), 220–226. https://doi.org/10.1016/j.supflu.2005.01.010
- Golovina, E. A., Hoekstra, F. A., & Hemminga, M. A. (1998). Drying increases intracellular partitioning of amphiphilic substances into the lipid Phase1: Impact on membrane permeability and significance for desiccation tolerance. Plant Physiology, 118(3), 975–986. https://doi.org/10.1104/pp.118.3.975
- Grayer, R. J., & Kokubun, T. (2001). Plant-fungal interactions: The search for phytoalexins and other antifungal compounds from higher plants. Phytochemistry, 56(3), 253–263. https://doi.org/10.1016/S0031-9422(00)00450-7
- Hess, M., Barralis, G., Bleiholder, H., Buhr, L., Eggers, T., Hack, H., & Stauss, R. (1997). Use of the extended BBCH scale—general for the descriptions of the growth stages of mono; and dicotyledonous weed species. Weed Research, 37(6), 433–441. https://doi.org/10.1046/j.1365-3180.1997.d01-70.x
- Hussain, H. A., Hussain, S., Khaliq, A., Ashraf, U., Anjum, S. A., Men, S., & Wang, L. (2018). Chilling and drought stresses in crop plants: Implications, cross talk, and potential management opportunities. Frontiers in Plant Science, 9(April), 1–21. https://doi.org/10.3389/fpls.2018.00393
- Isah, T. (2019). Stress and defense responses in plant secondary metabolites production. Biological Research, 52(1), 39. https://doi.org/10.1186/s40659-019-0246-3
- Jenks, M. A., Joly, R. J., Peters, P. J., Rich, P. J., Axtell, J. D., & Ashworth, E. N. (1994). Chemically induced cuticle mutation affecting epidermal conductance to water vapor and disease susceptibility in sorghum bicolor (L.) Moench. Plant Physiology, 105(4), 1239–1245. https://doi.org/10.1104/pp.105.4.1239
- Ji, X., & Jetter, R. (2008). Very long chain alkylresorcinols accumulate in the intracuticular wax of rye (secale cereale L.) leaves near the tissue surface. Phytochemistry, 69(5), 1197–1207. https://doi.org/10.1016/j.phytochem.2007.12.008
- Jones, W. P., & Kinghorn, A. D. (2012). Extraction of plant secondary metabolites. Natural Products Isolation, 3(12), 341–366. https://doi.org/10.1007/978-1-61779-624-1_13
- Kienzle, S., Carle, R., Sruamsiri, P., Tosta, C., & Neidhart, S. (2014). Occurrence of alk(en)ylresorcinols in the fruits of two mango (mangifera indica L.) cultivars during on-tree maturation and postharvest storage. Journal of Agricultural and Food Chemistry, 62(1), 28–40. https://doi.org/10.1021/jf4028552
- Knödler, M., Kaiser, A., Carle, R., & Schieber, A. (2008). Profiling of Alk(en)ylresorcinols in cereals by HPLC-DAD-APcI-MS n. Analytical and Bioanalytical Chemistry, 391(1), 221–228. https://doi.org/10.1007/s00216-008-1937-8
- Korycińska, M., Czelna, K., Jaromin, A., & Kozubek, A. (2009). Antioxidant activity of rye bran alkylresorcinols and extracts from whole-grain cereal products. Food Chemistry, 116(4), 1013–1018. https://doi.org/10.1016/j.foodchem.2009.03.056
- Kozubek, A., & Tyman, J. H. P. (1999). Resorcinolic lipids, the natural non-isoprenoid phenolic amphiphiles and their biological activity. Chemical Reviews, 99(1), 1–31. https://doi.org/10.1021/cr970464o
- Kozubek, A., Zarnowski, R., Stasiuk, M., & Gubernator, J. (2001). Natural amphiphilic phenols as bioactive compounds. Cellular and Molecular Biology Letters, 6(2A), 351–355.
- Krakowska-Sieprawska, A., Kiełbasa, A., Rafińska, K., Ligor, M., & Buszewski, B. (2022). Modern methods of pre-treatment of plant material for the extraction of bioactive compounds. Molecules, 27(3), 730. https://doi.org/10.3390/molecules27030730
- Lancashire, P. D., Bleiholder, H., Boom, T. V. D., Langelüddeke, P., Stauss, R., Weber, E., & Witzenberger, A. (1991). A uniform decimal code for growth stages of crops and weeds. Annals of Applied Biology, 119(3), 561–601. https://doi.org/10.1111/j.1744-7348.1991.tb04895.x
- Landberg, R., Kamal-Eldin, A., Salmenkallio-Marttila, M., Rouau, X., & Åman, P. (2008). Localization of alkylresorcinols in wheat, rye and barley kernels. Journal of Cereal Science, 48(2), 401–406. https://doi.org/10.1016/j.jcs.2007.09.013
- Magnucka, E. G., Pietr, S. J., & Zarnowski, R. (2015). Dynamics of alkylresorcinols during rye caryopsis germination and early seedling growth. Zeitschrift Fur Naturforschung - Section C Journal of Biosciences, 70(3–4), 71–73. https://doi.org/10.1515/znc-2014-4194
- Marentes-Culma, R., & Coy-Barrera, E. (2022). Effect of soil type on the temporal and spatial 5-n-alk(en)ylresorcinol variation in triticale grown under greenhouse conditions. Journal of Soil Science and Plant Nutrition, 22(4), 4428–4437. https://doi.org/10.1007/s42729-022-01043-z
- Marentes-Culma, R., Orduz-Díaz, L. L., & Coy-Barrera, E. (2019). Targeted metabolite profiling-based identification of antifungal 5-n-alkylresorcinols occurring in different cereals against Fusarium oxysporum. Molecules, 24(4), 770. https://doi.org/10.3390/molecules24040770
- Mcgoverin, C. M., Snyders, F., Muller, N., Botes, W., Fox, G., & Manley, M. (2011). A review of triticale uses and the effect of growth environment on grain quality. Jornal Science Food Agriculture, 91(January), 1155–1165. https://doi.org/10.1002/jsfa.4338
- Melliou, E., Magiatis, P., & Skaltsounis, A. L. (2003). Alkylresorcinol derivatives and sesquiterpene lactones from cichorium spinosum. Journal of Agricultural and Food Chemistry, 51(5), 1289–1292. https://doi.org/10.1021/jf025848g
- Miché, L., Belkin, S., Rozen, R., & Balandreau, J. (2003). Rice seedling whole exudates and extracted alkylresorcinols induce stress-response in Escherichia coli biosensors. Environmental Microbiology, 5(5), 403–411. https://doi.org/10.1046/j.1462-2920.2003.00432.x
- Moldoveanu, S., & David, V. (2015). Solvent extraction. In Modern sample preparation for chromatography (pp. 131–189). Elsevier. https://doi.org/10.1016/B978-0-444-54319-6.00006-2
- Peña, R. J. (2004). Food uses of triticale. In M. Mergoum and H. Gómez-Macpherson (Eds.), Triticale improvement and production (pp. 119–122). Rome, Italy: Food and Agriculture Organization of the United Nations (FAO).
- Piasecka, A., Jedrzejczak-Rey, N., & Bednarek, P. (2015). Secondary metabolites in plant innate immunity: Conserved function of divergent chemicals. New Phytologist, 206(3), 948–964. https://doi.org/10.1111/nph.13325
- Rebolleda, S., Beltrán, S., Sanz, M. T., González-Sanjosé, M. L., & Solaesa, Á. G. (2013). Extraction of alkylresorcinols from wheat bran with supercritical CO 2. Journal of Food Engineering, 119(4), 814–821. https://doi.org/10.1016/j.jfoodeng.2013.07.008
- Ross, A. B. (2019). Alkylresorcinols. In J. Johnson and T. Wallace (Eds.), Whole grains and their bioactives: Composition and health (pp. 393–406). John Wiley & Sons Ltd. https://doi.org/10.1002/9781119129486
- Ross, A., Shepherd, M., Schüpphaus, M., Sinclair, V., Alfaro, B., Kamal-Eldin, A., & Åman, P. (2003). Alkylresorcinols in cereals and cereal products. Journal of Agricultural and Food Chemistry, 51(14), 4111–4118. https://doi.org/10.1021/jf0340456
- Sampietro, D. A., Belizán, M. M. E., Apud, G. R., Juarez, J. H., Vattuone, M. A., & Catalán, C. A. N. (2013a). Alkylresorcinols: Chemical properties, methods of analysis and potential uses in food, industry and plant protection. Natural Antioxidants and Biocides from Wild Medicinal Plants June 2016, pp. 148–166. https://doi.org/10.1079/9781780642338.0148
- Sampietro, D. A., Jimenez, C. M., Belizán, M. M., Vattuone, M. A., & Catalán, C. A. N. (2013b). Development and validation of a micromethod for fast quantification of 5-n-alkylresorcinols in grains and whole grain products. Food Chemistry, 141(4), 3546–3551. https://doi.org/10.1016/j.foodchem.2013.06.069
- Sapirstein, H. D. (2016). Bioactives in wheat bran. Encyclopedia of Food Grains, 1–9. https://doi.org/10.1016/B978-0-12-394437-5.00109-1
- Schirmer, M. A., Freo, J. D., Müller, M. M., Bueno, P. D. F., Prestes, D. H., & Elias, M. C. (2006). Effects of drying methods and storage period in the industrial quality of wheat. In I. Lorini, B. Bacaltchuk, H. Beckel, D. Deckers, E. Sundfeld. J. P. dos Santos, J. D. Biagi, J. C. Celaro, L. R. D’A. Faroni, L.de O. F. Bortolini, M. R. Sartori, M. C. Elias, R. N. C. Guedes, R. G. da Fonseca, & V. M. Scussel (Eds.), 9th International Working Conference on Stored-Product Protection, Campinas, São Paulo, Brazil, 15–18 October 2006 (pp. 1019–1025). Passo Fundo, RS, Brazil: Brazilian Post-harvest Association–ABRAPOS.
- Schultz, J. C., Appel, H. M., Ferrieri, A. P., & Arnold, T. M. (2013). Flexible resource allocation during plant defense responses. Frontiers in Plant Science, 4(AUG), 1–11. https://doi.org/10.3389/fpls.2013.00324
- Shah, I., Shah, M. A., Nawaz, M. A., Pervez, S., Noreen, N., Vargas de la Cruz, C., Khan, F., Blundell, R., Briffa, J., Azzopardi, J., & Niaz, K. (2020). Analysis of other phenolics (capsaicin, gingerol, and alkylresorcinols). In A. S. Silva; S. F. Nabavi; M. Saeedi, and S. M. Nabavi (Eds.), Recent advances in natural products analysis (1st ed. pp. 255–271). Elsevier Inc. https://doi.org/10.1016/b978-0-12-816455-6.00006-8
- Takeuchi, T., Pereira, G., Braga, M., Maróstica, M., Leal, P., & Meireles, A. (2009). Low-pressure solvent extraction (solid–liquid extraction, microwave assisted, and ultrasound assisted) from condimentary plants. In M. A. Meireles (Ed.), Extracting bioactive compounds for food products: Theory and applications (contemporary food engineering) (1st ed., pp. 137–218). Boca Raton, Fl, USA: CRC Press.
- Wang, J., Gao, X., & Wang, Z. (2019). Non-destructive determination of alkylresorcinol (ARs) content on wheat seed surfaces and prediction of ARs content in whole-grain flour. Molecules, 24(7), 21–23. https://doi.org/10.3390/molecules24071329
- Witzenberger, A., Hack, H., & van den Boom, T. (1989). Erlâuterungen zum BBCH-Dezimal-Code fûr die Entwicklungsstadien des Getreides - mit Abbildungen. Gesunde Pflanzen, 41(11), 384–388.
- Żarnowski, R., Suzuki, Y., Yamaguchi, I., & Pietr, S. J. (2002). Alkylresorcinols in barley (hordeum vulgare L. distichon) grains. Zeitschrift Für Naturforschung C, 57(1–2), 57–62. https://doi.org/10.1515/znc-2002-1-210
- Zaynab, M., Fatima, M., Abbas, S., Sharif, Y., Umair, M., Zafar, M. H., & Bahadar, K. (2018). Role of secondary metabolites in plant defense against pathogens. Microbial Pathogenesis, 124, 198–202. https://doi.org/10.1016/j.micpath.2018.08.034
- Zhang, Q. W., Lin, L. G., & Ye, W. C. (2018). Techniques for extraction and isolation of natural products: A comprehensive review. Chinese Medicine (United Kingdom), 13(1), 1–26. https://doi.org/10.1186/s13020-018-0177-x
- Zhao, X., Huang, L., Kang, L., Jetter, R., Yao, L., Li, Y., Xiao, Y., Wang, D., Xiao, Q., Ni, Y., & Guo, Y. (2019). Comparative analyses of cuticular waxes on various organs of faba bean (vicia faba L.). Plant Physiology and Biochemistry, 139(March), 102–112. https://doi.org/10.1016/j.plaphy.2019.03.015
- Zhou, L., & Elias, R. J. (2013). Understanding antioxidant and prooxidant mechanisms of phenolics in food lipids. In Lipid oxidation: Challenges in food systems (pp. 297–321). AOCS Press. https://doi.org/10.1016/B978-0-9830791-6-3.50012-6