ABSTRACT
The genus, Cicer, accomodate chickpeas which offer a highly nutritious profile for human wellness. In this study, we hydrolyzed different species of Cicer seed proteins using various proteolytic enzymes to determine DH, antioxidant, ACE-I inhibitory and anti-inflammatory activity. The alcalase hydrolysis experimented for 120 minutes achieved maximum peptide content. And subsequent size fractionation of peptide by G-50 gel filtration column chromatography showed an enhancement in bioactivity. The alcalase hydrolysate demonstrated highest ACE inhibition (66.18 ± 2.65%, 64.22 ± 1.32%, and 60.19 ± 1.46%) in Cicer arietinum, Cicer reticulatum, and Cicer echinospermum, respectively. Furthermore, alcalase hydrolysate showed promising antioxidant efficacy measured by various techniques. Hyaluronidase inhibition was moderate, while lipoxygenase inhibition of alcalase hydrolysate showed an elevated response (IC50 = 32.6 ± 1.4 μg/ml for C. arietinum). Among all proteases, alcalase generated maximum degree of hydrolysis, resulting in peptides that exhibited significantly improved bioactivity.
1. Introduction
Plant peptides have received increased attention due to their health benefits, which include antioxidant potential as inhibitors of lipid peroxidation and free radical scavenging (Ghribi et al., Citation2015; Gupta et al., Citation2022; Li et al., Citation2008; Ying et al., Citation2021). Enzymatic hydrolysates from a variety of legume seeds, can be obtained using single or multi-enzyme hydrolysis, generating bioactive peptides of health interest (Erdmann et al., Citation2008; Garcia et al., Citation2013; Gupta et al., Citation2022; Udenigwe & Aluko, Citation2012). Literature has described the antioxidant activities of plant food proteins produced by enzymatic hydrolysis, such as bovine caseins and whey proteins (Pihlanto, Citation2006), soy proteins (Moure et al., Citation2006), and wheat protein (Kim, Citation2017).
Plant-based peptides are produced in an environmentally friendly manner and have to put-out health benefits. Ingesting plant-based peptides is in line with the Sustainable Development Goals (SDGs), which include “Good health and well-being”. It is challenging to link peptides’ real health-promoting benefits to their bioavailability, that has been studied on small scale (Rivero-Pino, Citation2023). The stability of such peptides is reliant on the sequence, is closely linked to their functionality. Certain peptides produced by enzymatic hydrolysis are bioactive, whereas others are not. The manufacturer can claim that the product may have health-promoting characteristics because of the bioavailable peptides that may be involved in preventing illness (Cruz-Casas et al., Citation2021; Manzoor et al., Citation2022).
Chickpea seeds belonging to the family Leguminosae come in two distinct types: desi and kabuli. Desi seeds are typically dark brown in color with a rough exterior, while kabuli seeds are mostly white, large, and have a smooth texture. The Cicer species are divided into three gene pools, with the primary gene pool including C. arietinum, the most cultivated species, and two wild annual species, C. echinospermum and C. reticulatum. The secondary gene pool includes C. judaicum and C. Pinnatifidium, while the tertiary gene pool includes three annual species, including C. cuneatum. Chickpea seeds are considered to have the best nutritional profiles among all grain legumes (Bhagyawant et al., Citation2018; Gupta et al., Citation2016; Gupta et al., Citation2017), with attributes such as antioxidant and angiotensin I-converting enzyme (ACE-I) inhibition (Bhagyawant et al., Citation2019; Gupta & Bhagyawant, Citation2019a, Citation2019b), antifungal properties (Gupta, Gautam, et al., Citation2018c), and anticancer effects (Gupta & Bhagyawant, Citation2021; Gupta et al., Citation2018) having been identified.
Bioactive peptides produced by enzymatic hydrolysis of proteins show better biological characteristics. Such polypeptides with a specified size can be obtained by adjusting the enzymatic hydrolysis’s parameters. Many parameters affect the bioactivity of protein hydrolysates, such as the type of protease enzyme, substrate concentration, pH, incubation time, and enzyme to substrate ratio (Cruz-Casas et al., Citation2021). A hydrolysate that has potential to be used as a food additive must be made with an enzyme that is commercially accessible, permitted for use in food, and at a cost that manufacturer can afford. Studies on protein hydrolysates from Cicer by different proteases impact its antioxidant, ACE-inhibitory and anti-inflammatory activity are lacking. Thus, the goal of this study was to choose a food-grade, readily accessible, and affordable enzyme that would enable the hydrolysate of Cicer (primary, secondary, and tertiary gene pool) to identify the level of afore mentioned bioactivities.
2. Materials and methods
2.1. Materials
This study, primarily focused on the seeds of various Cicer species that belong to three distinct gene pools. Specifically, we investigated the antioxidant and ACE-inhibitory properties of seed protein hydrolysates from C. arietinum L., C. reticulatum, C. echinospermum, C. pinnatifidum, C. judaicum, and C. cuneatum. These seeds were obtained from two renowned institutions, namely Indian Institute of Pulses Research (IIPR) Kanpur, India and International Crops Research Institute for the Semi-Arid Tropics (ICRISAT) Hyderabad, India, as detailed in .
Table 1. Agronomic details of various Cicer species used in this study.
2.2. Preparation of chickpea protein peptide
As per the methodology described in a prior publication by Yust et al. (Citation2003), the Cicer seed protein was isolated from the flour. Specifically, the flour was subjected to protein extraction using distilled water, followed by stirring in a cold environment for one hour and subsequently centrifuged at 10,000 × g for 15 minutes to yield the chickpea protein isolate that designated as CPI.
2.3. Hydrolysis of chickpea protein
The approach described by Ghribi et al. (Citation2015) was employed to carry out protein hydrolysis. The enzymatic hydrolysis was executed using several proteases, including alcalase (in Tris – HCl buffer, pH 8, 55 °C), chymotrypsin (in phosphate buffer, pH 6.8, 50°C), flavourzyme (a peptidase preparation from Aspergillus oryzae) (in Tris – HCbuffer, pH 8, 55 °C), papain, pepsin and trypsin (as listed in ). The reaction mixtures containing the protein and enzyme were subjected to incubation in water bath shaker and the enzymatic reaction was subsequently terminated by boiling for 10 minutes. Finally, the Cicer protein hydrolysate obtained was designated as CPH upon centrifugation (at 10,000 × g for 10 minutes).
Table 2. Statistics of hydrolysis of chickpea protein with different enzymes.
2.4. Determination of peptide contents
The CPIs hydrolysis were monitored using the highly sensitive o-phthaldialdehyde (OPA) method, as described by Church et al. (Citation1983). Following the addition of OPA and β-mercaptoethanol to the sample, the mixture was incubated for 2 minutes and the resulting absorbance was measured at a wavelength of 340 nm.
2.5. Size-exclusion chromatography
CPIs and CPHs were subjected to gel filtration using a Sephadex G-50 column (1 cm x 30 cm) with borate buffer (0.5 M) as eluent at a flow rate of 0.7 ml/min. The eluted fractions were analysed qualitatively by SDS-PAGE and their absorbance measured at 280 nm.
2.6. SDS-PAGE (Sodium dodecyl sulphate-polyacrylamide gel electrophoresis)
The proteins derived from both CPIs and CPHs were subjected to electrophoresis using the sodium dodecyl sulfate-polyacrylamide gel electrophoresis (SDS-PAGE) method described by Laemmli (Citation1970).
2.7. DPPH radical scavenging assay
Radical scavenging activity was measured using the Bondet et al. (Citation1997) methodology. 2 ml of methanol was used to Cicer protein isolates and hydrolysates. In a dark, 30-minute incubation period, 1 milliliter of supernatant was combined with 3 milliliters of 0.1 mM DPPH and the absorbance was read at 517nm. Ascorbic acid served as a positive control.
2.8. β – carotene bleaching method
To determine the antioxidant activity of CPIs and CPHs, the method described by Koleva et al. (Citation2002) was employed. A mixture of ß- carotene (0.5 mg), Tween-40, and linoleic acid was incubated at 50°C for 2 hours and the absorbance was measured at 470 nm. To establish a positive control, BHA was utilized.
2.9. Determination of reducing power
The assessment of the reducing power of CPIs and CPHs were carried out using a method described by Ahmad et al. (Citation2015). In brief, the sample was mixed with sodium phosphate buffer and potassium ferricyanide (1%) and incubated for 20 min at 50°C. Following incubation, trichloroacetic acid (TCA) was added to the mixture, and the absorbance was measured at 700 nm.
2.10. Metal chelating activity
To determine the ferrous chelating activity, the method proposed by Decker and Welch (Citation1990) was employed. In brief, the sample was added to a solution containing 2 mM FeCl2 and 5 mM ferrozine, followed by incubation at room temperature for 10 minutes with vigorous shaking. The formation of ferrozine-Fe2+ complex was inhibited by chelating activity of sample which was measured at 562 nm.
2.11. Extraction of ACE from rabbit lung
The pharmacology laboratory of the Defence Research & Development Establishment (DRDE), Gwalior, Madhya Pradesh, India, provided rabbit lungs, which were utilized to extract the enzyme. ACE enzyme was extracted using the methodology described by Cushman and Cheung (Citation1971). The methodology involved homogenizing ground lungs in acetone followed by centrifugation and evaporation. The resulting residue was then dissolved in phosphate buffer and subjected to centrifugation to obtain a clear supernatant. This supernatant was used as a source of ACE, as reported by Gupta and Bhagyawant (Citation2018a).
2.11.1. ACE-I inhibition assay
The enzymatic assay to determine the inhibitory activity of CPIs and CPHs against ACE-I using a modified version of the method developed by Cushman and Cheung (Citation1971). In this assay, the reaction mixture consisted of 100 mM phosphate buffer (pH 8.3), 300 mM NaCl, 5 mM hippuryl-L-histidyl-L-leucine (HHL) as the substrate, and ACE. The reaction was allowed to proceed at 37°C for 30 min, after which it was terminated by adding 1 N HCl. Ethyl acetate was added to reaction mixture and vortexed for 15 seconds. The ethyl acetate layer was collected and allowed to evaporate. The residue was redissolved and subjected to absorbance measurement at 228 nm.
2.12. In vitro anti-inflammatory assay
2.12.1. HYA inhibitory activity
Sahasrabud and Deodhar’s (Citation2010) modified approach was used to evaluate the inhibition of HYA. Following a 20-min incubation period at 37°C, Bovine HYA (400 U/mL) in acetate buffer (0.1 M) and different concentrations of CPHs were added. In the end, 12.5 mM CaCl2 was added followed by incubation for 20 min at 37°C. Sodium hyaluronate was added to initiate the reaction, which was then incubated for 45 minutes at 37°C. Next, 0.4 M potassium borate and 0.4 M sodium hydroxide solution were added to the reaction mixture, and further incubated for three minutes in water at 90°C. After 20 minutes, the absorbance at 585 nm was measured after adding 10% p-Dimethyl-aminobenzaldehyde to the chilled reaction mixture. Standard drug was ibuprofen.
2.12.2. LOX inhibition
A spectrophotometric test was used to measure the LOX inhibitory activity (Perera et al., Citation2018). The approach relies on the creation of 13-hydroperoxyocta-decadienoic acid during the lipoxygenation process, which results in an increase in absorbance at 234 nm. After incubating for 15 minutes with LOX solution (2200 U/mL) and borate buffer 0.2 M (pH 9.0) or CPHs in borate buffer, the mixture was mixed with linoleic acid, and the absorbance was measured for 2 minutes at 234 nm. For CPHs, the IC50 values were determined by using percentage inhibition. The standard drug was to use ibuprofen.
3. Results and discussion
3.1. Generation of peptide from Cicer isolates
CPIs was subjected to hydrolysis by a variety of proteolytic enzymes, including alcalase, flavourzyme, chymotrypsin, papain, pepsin, and trypsin, under their respective optimum conditions (). The resulting protein hydrolysates exhibited varied degrees of bioactivity, with those produced by alcalase displaying the most potent bioactive properties (Rivero-Pino, Citation2023). Alcalase, an endopeptidase, was therefore predominantly employed in synthesis of bioactive peptides derived from the chickpea protein isolate (Gupta et al., Citation2022; Yust et al., Citation2003). The hydrolysis curve for the chickpea proteins, which spanned from 20 to 120 minutes of incubation, demonstrated that alcalase elicited the highest rate of hydrolysis initially at 40 minutes and progressively increased over the entire 120- minute duration. Among all the proteases, alcalase generated the maximum degree of hydrolysis, resulting in peptides that significantly improved antioxidant and ACE-I inhibitory activities.
3.2. SDS-PAGE
The CPIs and CPHs were subjected to qualitative separation via SDS-PAGE. The electropherogram unveiled visible bands corresponding to molecular weight (MWs) of 125, 72, 57, 42, 31, 24, 18, 15, 8, and 3.5 kDa in CPIs (). In the present study, enzymatic hydrolysis resulted in appearance of low MW bands in the CPHs (). According to Sanchez-Vioque et al. (Citation1999), the protein fractions of chickpea on SDS-PAGE ranged from 46.5 to 39.8 and 25.3 to 24.3 kDa MWs subunits. However, some of the bands of low MW were not distinctly visible in SDS-PAGE of alcalase hydrolysate. Only C. echinospermum showed a distinct band in range of 3.5 kDa in alcalase hydrolysate, whereas the flavourzyme hydrolysate pattern was characterized by three bands corresponding to MWs of 17, 6.5, and 3.5 (). Chymotrypsin generated protein subunits with MWs of 26.6, 17.0, 14.4, 6.5, and 3.5 kDa, but it did not hydrolyze fully. Papain hydrolysate produced bands with MWs of 26.6, 17.0, and 3.5 kDa, along with several hazy bands. The pepsin hydrolysate pattern generated numerous bands of high MWs that could be attributed to catalysis. Trypsin, on the other hand, revealed only one intensive band with a MW of 17 kDa (). The present study demonstrated that the alcalase hydrolysate bands disappeared on gel, indicating that alcalase hydrolysate generated low molecular weight peptides that are not retained on SDS-PAGE. The literature domain reports data on diminutive molecular weight peptides exhibiting ACE inhibitory activities that occupy the angiotensin-converting enzyme active sites (Guang & Phillips, Citation2009). The bioactive peptides consisting of 2 to 20 amino acids correspond to low molecular weight (Gupta & Bhagyawant, Citation2019b; Wang and Mejia, Citation2005). It is noteworthy that proteins can act as bioactive principles, but their large size hinders them from crossing cellular membranes. The best approach to produce short amino acid composition peptides with biological activity is through hydrolysis.
Figure 1. Sodium dodecyl sulfate-polyacrylamide gel electrophoresis separation of Cicer seed protein isolates.
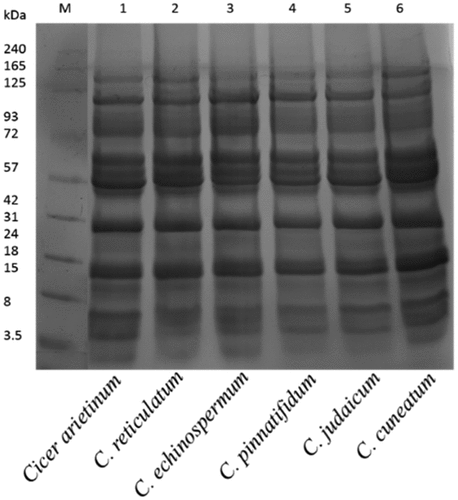
3.3. Degree of hydrolysis
Protein hydrolysis, referred to as DH, involves the cleavage of peptide bonds and quantifies the ratio of peptide bonds present in a substrate (Adler-Nissen, Citation1982; Rutherfurd, Citation2010). The DH provides a convenient means of analysing hydrolysates and different types of peptides. They are generated by hydrolysis, depending on the enzyme specificity, its nature and the reaction temperature (Rutherfurd, Citation2010). OPA is an accurate and easily-performed method for determining the DH, with a wide range of applications (Nielsen et al., Citation2001). Alcalase, an endopeptidase, hydrolyzes peptides with a broad specificity and releases hydrophobic amino acids such as Phe, Tyr, Trp, Leu, Ile, Val and Met (Markland & Smith, Citation1971). The peptides generated by alcalase exhibit better functional properties than the unhydrolyzed protein, and have been investigated for ACE-I inhibitory activity in chickpea isolates (Gupta & Bhagyawant, Citation2019b; Gupta et al., Citation2022; L. G. Hong et al., Citation2005).
In present study, the CPIs was obtained by solubilizing it in NaOH and subsequently precipitating with HCl. The resulting protein was further hydrolyzed using proteolytic enzymes and the DH was assessed by determining the peptide content. Previous studies have suggested that a DH below 50% can be achieved by hydrolysis for less than 6 h (Cai et al., Citation2013). In this study, enzymes were added at various intervals to enhance DH and maximize the production of minor peptides. The degree of hydrolysis was monitored for 120 minutes at 20-minute intervals for all Cicer species.
In , C. arietinum exhibited a trend in degree of hydrolysis across various enzymes, namely alcalase, flavourzyme, chymotrypsin, papain, pepsin, and trypsin. Notably, the enzymes yielded different levels of DH, with alcalase and flavourzyme demonstrating the highest DH of 84.3 ± 2.6% and 64.3 ± 2.6%, at 120 min respectively, while chymotrypsin exhibited the lowest DH of 49.3 ± 4.4%. After 100 minutes of hydrolysis, the DH for most of the enzymes stabilized, indicating that the process was complete.
Figure 4. C. arietinum protein hydrolysates generated by different enzymes: (a) time course (degree of hydrolysis) of hydrolysis catalyzed by different proteases (b) maximum degree of hydrolysis achieved by different proteases. Values are means ±standard deviations (SDs) of three determinations.
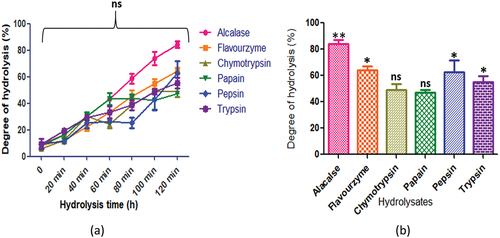
displays the hydrolysis of C. reticulatum protein by various proteases. The protein hydrolysates generated distinct peptide compositions during hydrolysis (p < .05). Specifically, alcalase yielded the highest peptide content at 120 min (82.3 ± 3.0%), followed by flavourzyme (63.32 ± 1.61%), trypsin (48.8 ± 3.3%), papain (45.3 ± 1.6%), and pepsin (52.7 ± 6.3%). In contrast, chymotrypsin (48.7 ± 2.1%) produced the highest peptide content after 100 min of hydrolysis.
Figure 5. C. reticulatum protein hydrolysates generated by different enzymes: (a) time course (degree of hydrolysis) of hydrolysis catalyzed by different proteases (b) maximum degree of hydrolysis achieved by different proteases. Values are means ±standard deviations (SDs) of three determinations.
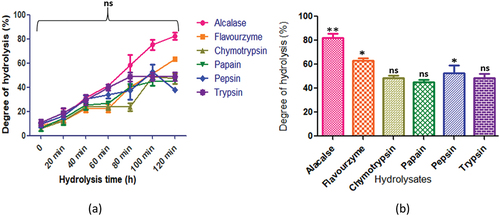
The hydrolysis of C. echinospermum protein using various enzymes generated different peptide contents as hydrolysis progressed. Alcalase produced the highest peptide content both at 100 and 120 min (74.21 ± 4.24%), with other enzymes yielding the following corresponding peptide contents: flavourzyme (64.32 ± 5.4%), pepsin (57.8 ± 4.7%), and trypsin (55.32 ± 4.0%). Chymotrypsin (52.32 ± 5.8%) and papain (50.8 ± 1.9%) demonstrated the highest peptide content after 120 min of hydrolysis ().
Figure 6. C. echinospermum protein hydrolysates generated by different enzymes: (a) time course (degree of hydrolysis) of hydrolysis catalyzed by different proteases (b) maximum degree of hydrolysis achieved by different proteases. Values are means ±standard deviations (SDs) of three determinations.
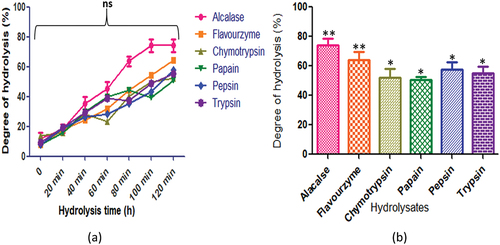
The seeds of C. pinnatifidum protein displayed varying degrees of hydrolysis, with alcalase producing the highest peptide content at 120 min (80.21 ± 2.8%). The peptide content for other enzymes was as follows: flavourzyme (65.82 ± 4.7%), chymotrypsin (50.8 ± 5.14%), papain (50.8 ± 1.9%), and pepsin (50.32 ± 3.02%). On the contrary, trypsin (59.7 ± 3.5%) exhibited the highest peptide content after 100 min of hydrolysis (). In , the degree of hydrolysis for C. judaicum protein revealed different peptide contents after 120 min of hydrolysis. Alcalase produced the highest peptide content at 100 min (75.71 ± 2.1%), while other enzymes yielded the following corresponding peptide contents: flavourzyme (65.32 ± 4.0%), chymotrypsin (50.8 ± 2.3%), papain (49.8 ± 3.3%), and pepsin (48.32 ± 3.02%). Trypsin (62.82 ± 1.9%) exhibited the highest peptide content after 120 min of hydrolysis.
Figure 7. C. pinnatifidum protein hydrolysates generated by different enzymes: (a) time course (degree of hydrolysis) of hydrolysis catalyzed by different proteases (b) maximum degree of hydrolysis achieved by different proteases. Values are means standard deviations (SDs) of three determinations.
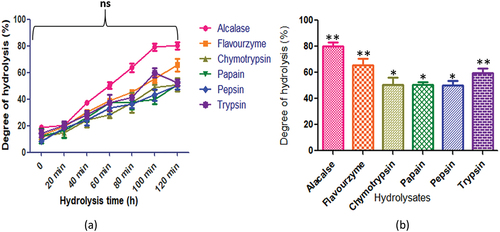
Figure 8. C. judaicum protein hydrolysates generated by different enzymes: (a) time course (degree of hydrolysis) of hydrolysis catalyzed by different proteases (b) maximum degree of hydrolysis achieved by different proteases. Values are means standard deviations (SDs) of three determinations.
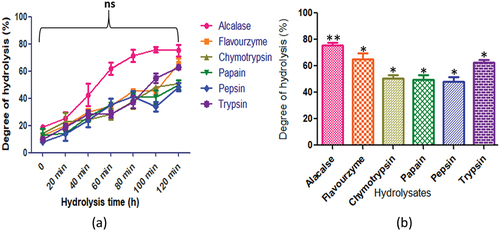
presents the degree of hydrolysis displayed by C. cuneatum. The employed enzymes, namely alcalase, flavourzyme, chymotrypsin, papain, pepsin, and trypsin, exhibited different degrees of hydrolysis (p < .05) with values of 73.21 ± 4.2%, 63.3 ± 4.0%, 50.8 ± 2.3%, 48.8 ± 1.9%, 49.8 ± 3.7%, and 62.3 ± 2.6%, respectively. After 120 minutes of hydrolysis, the highest peptide content was observed for all enzymes.
3.4. Size distribution of chickpea hydrolysates
The size and bio-activity of peptides are affected by the production processand hence, protease efficiency is assessed to release bio-active peptides. Changes in CPHs were observed in the size exclusion chromatogram, as produced by different proteases, as shown in . The data demonstrated that chymotrypsin, pepsin, and papain did not completely hydrolyze CPI, as the high molecular weight band was visible in their chromatograms but was absent in alcalase, flavourzyme, and trypsin chromatograms. The alcalase and flavourzyme generated exoprotease activity, resulting in a high-intensity peak at 280 nm of a low molecular weight band (). Similarly, the chromatograms of CPHs from chymotrypsin, papain, pepsin, and trypsin did not exhibit high peak intensity. The results suggested that alcalase and flavourzyme had greater endoprotease activity than other proteases. Low molecular weight peptides had a more significant effect on ACE inhibitory activity than high molecular weight peptides (Gupta et al., Citation2022). Therefore, alcalase and flavourzyme CPHs have potential as ingredients in antihypertensive formulations (Terashima et al., Citation2011).
3.5. Antioxidant activity
Antioxidants in food play a significant role in inhibiting damage (Samaranayaka & Li-chan, Citation2011). The antioxidant activity facilitates the scavenging of radicals through proton donation and metal-ion chelation (Jung et al., Citation1995; Mine, Citation2010; Xie et al., Citation2008). Biopeptides are functional small amino acid sequences that demonstrate anticancer and blood pressure-lowering (ACE inhibitory) properties (Dikmen et al., Citation2017; F. Hong et al., Citation2008). They remain inactive in native protein sequences and can be produced through enzymatic hydrolysis to exert various physiological functions. The CPIs and CPHs were evaluated for their antioxidant activity using various methods, as discussed below.
3.5.1. DPPH free radical scavenging activity
Free radicals are highly reactive and can be scavenged by various substances, including DPPH•. This particular radical has an electron that displays maximum absorbance at 517 nm. When DPPH• is prepared in ethanol, its absorbance decreases gradually due to scavenging activity, changing the color of the reaction from crimson to yellow (Yamaguchi et al., Citation1998). The reduction in absorbance may be related to proton-donating substances (Xie et al., Citation2008, Cheng et al., Citation2006).
Additionally, high DH after enzyme digestion may represent extra accessibility by DPPH• for its activity, as it enhances the hydrophobic amino acid side chains (Cheng et al., Citation2006; Qin et al., Citation2010; Tang et al., Citation2009; Yamaguchi et al., Citation1998). shows diverse changes in radical scavenging capacity achieved by different proteases in the C. arietinum hydrolysates. The highest radical scavenging activity was observed with alcalase treatment (72.32 ± 5.45%), while the lowest was observed with papain treatment (42.32 ± 3.02%) (). Peptides generated by flavourzyme, chymotrypsin, pepsin, and trypsin exhibited 54.32%, 48.32%, 47.82%, and 57.82% scavenging activity, respectively. The hydrolysates of wild chickpea (C. reticulatum) showed the highest DPPH activity with alcalase produced fractions (67.32 ± 4.04%) and the lowest with pepsin hydrolysate (38.32 ± 5.45%) (). Hydrolysates obtained by incubation with various proteases for 120 min, including C. echinospermum, C. pinnatifidum, C. judaicum, and C. cuneatum, showed different DPPH activity levels, with the highest activity observed with alcalase enzyme treatment (54.71 ± 4.94%, 75.71 ± 4.94%, 71.21 ± 4.24%, and 6.42 ± 2.8%, respectively) (). The lowest activity was observed with papain treatment in C. judaicum (44.32 ± 3.02%) and C. cuneatum (47.32 ± 1.21%) enzymatic hydrolysates.
Figure 11. Radical-scavenging ability of CPH generated by various proteases after 2 h of incubation. Panels (a–f) correspond to Cicer arietinum, C. reticulatum, C. echinospermum, C. pinnatifidum, C. judaicum and C. cuneatum.
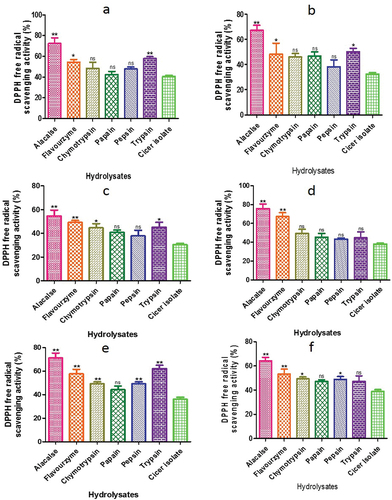
Previous studies have suggested that high DPPH activities in hydrolysates or peptides are related to the presence of hydrophobic amino acids (Li et al., Citation2008) and that scavenging activity increases with increment of DH (Chen et al., Citation2005; Jamdar et al., Citation2010). Similar trends have been observed in other species (You et al., Citation2009). The authors of this study have provided data that supports the increase in scavenging activity with increasing DH (Gupta, Srivastava, et al., Citation2018d).
3.5.2. β carotene bleaching assay
The measurement of antioxidant activity often utilizes bioactive peptides, which can be assessed through the discoloration of β-carotene assay. This assay is highly sensitive to the free radical mediated oxidation of linoleic acid, and the oxidation product of lipid can react with protein hydrolysates to bring about further oxidation. In this study, the lipid peroxidation of Cicer protein and its hydrolysates was evaluated by assessing their ability to hinder linoleic acid oxidation (). The CPIs and CPHs were effective in inhibiting β-carotene oxidation, with the alcalase hydrolysate of C. cuneatum demonstrating the highest antioxidant activity (74.11%). Furthermore, using alcalase, C. arietinum had better antioxidant activity 70.12 ± 2.46%, while the papain hydrolysate had the lowest (46.06 ± 1.02%). The antioxidant activity index of peptides in free radical-associated lipid peroxidation is dependent on their molecular size, chemical properties, and electron shifting capability of amino acid sequences (Qian et al., Citation2008).
3.5.3. Reducing power
The antioxidant activity of a peptide is associated with its reducing power (Huang et al., Citation2004). To determine the concentration of Fe2+, the reducing power of CPI and CPHs was measured at 700 nm (Ferreira et al., Citation2007). The range of reducing power values observed for CPIs and CPHs were 1.06–2.10, with the highest values being produced by alcalase at 120 min (2.1, 2.18, 1.98. 2.21, 2.09 and 2.19) and the lowest values by papain (1.06, 0.90, 0.94, 0.99, 0.99 and 1.09) (). The reducing power of the hydrolysates increased as the hydrolysis time increased from 20 to 120 min. All Cicer hydrolysates showed better reducing power than protein isolates. The reducing power of alcalase hydrolysates was higher than that of chickpea protein hydrolysate purified fractions (Li et al., Citation2008) and higher than that of wheat germ protein hydrolysates (Kim et al., Citation2017). The reducing power produced by alcalase in the wheat germ protein hydrolysates was substantial and contributed to their total antioxidant activity. Therefore, the data revealed that Cicer protein hydrolysates synthesized by alcalase, trypsin, and flavourzyme showed excellent capacity towards reducing power.
3.5.4. Metal chelating activity
The capacity of hydrolysates to chelate Fe2+ was used to measure the antioxidant activity. This chelating ability was measured by decrease in absorbance of ferrozine-Fe2+ complexes following the addition of hydrolysates. The CPHs displayed a range of Fe2+ chelating activity, ranging from 66.23 ± 2.1% to 42.13 ± 1.57% chelation (). Among the different proteases used for hydrolysis, alcalase produced the highest Fe2+ chelating ability, while chymotrypsin produced the lowest. Other studies have also reported that hemp and mung protein hydrolysates produced by various proteases exhibited better Fe2+ chelating capability than seeds (Gupta, Srivastava, et al., Citation2018d).
Figure 14. Metal chelating activity of CPH generated by various proteases after 2 h of incubation. Panels (a–f) refer to Cicer arietinum, C. reticulatum, C. echinospermum, C. pinnatifidum, C. judaicum and C. cuneatum respectively.
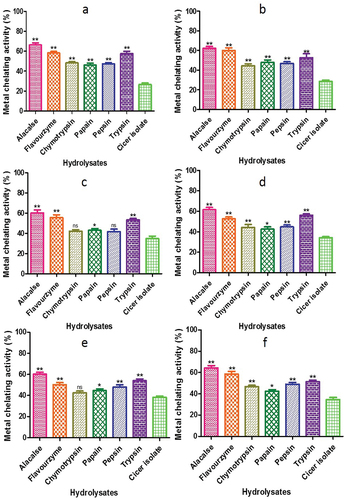
Iron and other metals function as precipitants in many food systems because of the faster process by which lipid hydroperoxides break down into very reactive alkoxyl radicals (McClements & Decker, Citation2000). The ability of hydrolyzed proteins, derived from diverse origins, to chelate iron is contingent upon the type of enzyme employed, the characteristics of the starting protein, and the extent of hydrolysis (Pihlanto, Citation2006). Amino acid sequences are present in all protein sources, and these sequences have varying effects on the ability of metal ions to chelate. For example, caseins with polar sections, found in a variety of protein sources, are composed of serine-serine-serine-glutamic acid-glutamic acid sequences and phosphorylated residues. By forming a combination of calcium, iron, and zinc, these components function as effective cleavers (Shahidi and Zhong, Citation2015). Jamdar et al. (Citation2010) observed comparable outcomes examining the impact of hydrolysis conditions and intensity on the iron ion chelating proteins hydrolyzed by peanuts.
3.6. ACE inhibitory activity
showed distinct trends in the ACE inhibition of various fractions. Alcalase hydrolysate of C. arietinum exhibited the highest ACE inhibition of 66.18 ± 2.65%. In C. reticulatum and C. echinospermum, alcalase-generated peptides demonstrated the highest ACE inhibition of 64.22 ± 1.32% and 60.19 ± 1.46%, respectively, whereas papain resulted in the lowest ACE inhibition of 42.90 ± 2.19% and 41.89 ± 1.28%. Among the various Cicer species, alcalase peptides had highest ACE inhibition of 63.59 ± 2.87% in C. pinnatifidum and the lowest activity of 41.10 ± 1.76% was recorded with pepsin. Similarly, the highest and lowest ACE inhibition for C. judaicum and C. cuneatum was observed with alcalase-generated peptide, while the lowest activity was recorded with chymotrypsin-generated peptide (40.60 ±.1.72%, 41.29 ± 1.38%, respectively). The results suggest that the presence of high molecular weight (MW) peptides in chymotrypsin, papain, pepsin, and trypsin may have led to decreased ACE inhibitory activity, whereas low MW peptides showed greater ACE-inhibitory activity. Similarly, Gupta and Bhagyawant (Citation2019b) observed that, in comparison to flavourzyme at 100 min (IC50: 0.365 mg/mL), C. arietinum generated by Alcalase enzyme at 60 min exhibited the strongest ACE-inhibitory activity (IC50: 0.182 mg/mL). The reason for the greatest rise in ACEinhibitory activity is that alcalase produces peptides that are absorbed in the small intestine due to their resistance to gastrointestinal proteases. This suggests that the food industry may employ these peptides to lower blood pressure in individuals. This finding is consistent with previous studies by Zhu et al. (Citation2010) and Segura-Campos et al. (Citation2010), which showed that low MW peptide fractions had the best ACE-inhibitory activity. Therefore, the antihypertensive potential of CPHs is mainly dependent on the type of protease used for hydrolysis (Gupta et al., Citation2022). Gao et al. (Citation2010) also showed that the ACE-inhibitory activity of cotton seed protein hydrolysates was dependent on the protease type used, with papain hydrolysate being the most active among the six proteases tested.
3.7. Anti-inflammatory activity
Hyaluronidases (HYAs) and lipoxygenases (LOXs) are significant factors for the inflammatory process. The CPHs showed a similar inhibition value for each enzyme. Additionally, hyaluronidase inhibition was merely slight (IC50 = 57.2 ± 1.7 μg/ml), while lipoxygenase inhibition of alcalase hydrolysate shown an elevated response (IC50 = 32.6 ± 1.4 μg/ml for C. arietinum) comparable with standards (IC50 = 19.5 ± 2.2 μg/ml). C. reticulatum, C. echinospermum, C. pinnatifidum, C. judaicum, and C. cuneatum alcalase hydrolysate showed moderate impact with (IC50 = 59.4 ± 1.1, 62.1 ± 1.5, 69.6 ± 2.2, 70.3 ± 1.9, 65.6 ± 2.5 μg/ml) respectively, hyaluronidase inhibition. On the other hand lipoxygenase inhibition of alcalase hydrolysate demonstrated better efficiency (IC50 = 40.2 ± 0.9, 38.5 ± 0.5, 49.4 ± 1.8, 44.6 ± 0.8, 42.5 ± 1.7 μg/ml) respectively. It was reported that LOX and HYA inhibitory activities are closely related to the radical scavenging activity, and also the anti-inflammatory activity of the concentrated hydrolysates may be linked to the higher antioxidant activity (Alexander et al., Citation2024; Girish et al., Citation2009; Rudrapal, Eltayeb, et al., Citation2023, Citation2024). This is the first report that investigated the in vitro LOX and HYA activities of CPHs. The control of hyaluronic acid metabolism is mostly dependent on hyaluronidase inhibitors. Of the known hyaluronidase inhibitors, bioactive substances produced from plants are crucial. Numerous studies on the inhibition of hyaluronidase have demonstrated the strong anti-hyaluronidase properties of antioxidants such flavonoids and phenolic acids (Girish et al., Citation2009).
4. Conclusions
This study evaluated the effects of enzymatic hydrolysis on a CPI of C. arietinum, C. reticulatum, C. echinospermum, C. pinnatifidum, C. judaicum, and C. cuneatum‘s antioxidant and ACE inhibitory qualities. The ACE-inhibitory activity was increased during enzymatic hydrolysis of alcalase generated peptides, whereas chymotrypsin-generated peptides exhibited the least bioactivity. Alcalase hydrolysate of C. arietinum exhibited the highest ACE inhibition (66.18 ± 2.65%). Additionally, hyaluronidase inhibition was meret (IC50 = 57.2 ± 1.7 μg/ml), while lipoxygenase inhibition of alcalase hydrolysate showed an elevated response (IC50 = 32.6 ± 1.4 μg/ml for C. arietinum) comparable with standards (IC50 = 19.5 ± 2.2 μg/ml). Since alcalase is a serine protease, it brings about reflecting conformational changes that improve the efficiency of Cicer peptide. The outcomes showed that the antioxidant, metal chelating and ACE inhibitory activity of the protein of Cicer isolates was significantly enhanced by the enzymatic hydrolysis of protein. It is acceptable to infer from the evaluation of these parameters that the alcalase hydrolysis exhibited highest bioactivity. Thus, bioactive peptides derived from the enzymatic hydrolysis of chickpea protein were excellent source for the bioactivity as well as the ability to create and combine different food items to enhance overall health.
4.1. Future directions and implementations in regular life
The findings of this study prescribe significant implications for development of functional food particularly for the people with hypertension. Further studies warrent development of functional food products based on Cicer protein hydrolysates. These peptides can be further characterized for the development of new antihypertensive drugs under in-vivo conditions. The use of Cicer protein hydrolysates as a natural antihypertensive agent can be promoted as an open choice to synthetic drugs. Health authorities and policymakers can play an important role in promoting the use of such natural products. Public awareness campaigns and education programs can be conducted to educate people about the benefits of Cicer protein hydrolysates. And their role in preventing and managing hypertension. Thereby improving the quality of life for millions of people around the world.
Author contributions
Conceptualization: N.G. and S.S.B.; methodology, data collection and original data analysis: N.G.; data presentation, writing: N.G. and S.S.B.; reviewing and editing: N.G. and S.S.B.; A.H. and E.F.A.; funding acquisition: S.S.B.; A.H. and E.F.A. All authors have read and agreed to the published version of the manuscript.
Acknowledgments
The author is grateful to the Jiwaji University, Gwalior (India) for making this research study possible. The author is also thankful to the study subjects who volunteered to be a part of this study. The authors would like to extend their sincere appreciation to the Researchers Supporting Project Number (RSP2024R134), King Saud University, Riyadh, Saudi Arabia.
Disclosure statement
The authors declare that the research was conducted in the absence of any commercial or financial relationships that could be construed as a potential conflict of interest.
Additional information
Funding
References
- Adler-Nissen, J. (1982). Limited enzymic degradation of proteins: A new approach in the industrial application of hydrolases. Journal of Chemical Technology & Biotechnology, 32(1), 138–17. https://doi.org/10.1002/jctb.5030320118
- Ahmad, M., Baba, W. N., Wani, T., Gani, T. A., Gani, A., Shah, U., Wani, S. M., & Masoodi, F. A. (2015). Effect of green tea powder on thermal, rheological & functional properties of wheat flour and physical, nutraceutical & sensory analysis of cookies. Journal of Food Science and Technology, 52(9), 5799–5807. https://doi.org/10.1007/s13197-014-1701-3
- Alexander, R., Khaja, A., Debiec, N., Fazioli, A., Torrance, M., & Razzaque, M. S. (2024). Health-promoting benefits of lentils: Anti-inflammatory and anti-microbial effects. Current Research in Physiology, 7, 100124. https://doi.org/10.1016/j.crphys.2024.100124
- Bhagyawant, S. S., Gautam, A. K., Narvekar, D. T., Gupta, N., Bhadkaria, A., Srivastava, N., & Upadhyaya, H. D. (2018). Biochemical diversity evaluation in chickpea accessions employing mini-core collection. Physiology & Molecular Biology of Plants, 24(6), 1165–1183. https://doi.org/10.1007/s12298-018-0579-3
- Bhagyawant, S. S., Narvekar, D. T., Gupta, N., Bhadkaria, A., Gautam, A. K., & Srivastava, N. (2019). Chickpea (Cicer arietinum L.) lectin exhibit inhibition of ACE-I, α-amylase and α-glucosidase activity. Protein and Peptide Letters, 26(7), 494–501. https://doi.org/10.2174/0929866526666190327130037
- Bondet, V., Brand Williams, W., & Berset, C. (1997). Kinetics and mechanisms of antioxidant activity using the DPPH free radical method. LWT - Food Science & Technology, 30(6), 609–615. https://doi.org/10.1006/fstl.1997.0240
- Cai, L., Xiao, L., Liu, C., & Ying, T. (2013). Functional properties and bioactivities of pine nut (Pinus gerardiana) protein isolates and its enzymatic hydrolysates. Food and Bioprocess Technology, 6(8), 2109–2117. https://doi.org/10.1007/s11947-012-0885-7
- Chen, G., Zhao, L., Cong, T., Bao, S., & Bao, S.-F. (2005). In vitro study on antioxidant activities of peanut protein hydrolysate. Journal of the Science of Food & Agriculture, 87(2), 357–362. https://doi.org/10.1002/jsfa.2744
- Cheng, Y., Wang, Z., & Xu, S. (2006). Antioxidant properties of wheat germ protein hydrolysates evaluated in vitro. Journal of Central South University of Technology, 13(2), 160–165. https://doi.org/10.1007/s11771-006-0149-7
- Church, F. C., Swaisgood, H. E., Porter, D. H., & Catignani, G. L. (1983). Spectrophotometric assay using o-phthaldialdehyde for determination of proteolysis in milk and isolated milk proteins. Journal of Dairy Science, 66(6), 1219–1227. https://doi.org/10.3168/jds.S0022-0302(83)81926-2
- Cruz-Casas, D. E., Aguilar, C. N., Ascacio-Valdés, J. A., Rodríguez-Herrera, R., Chávez-González, M. L., & Flores-Gallegos, A. C. (2021). Enzymatic hydrolysis and microbial fermentation: The most favorable biotechnological methods for the release of bioactive peptides. Food Chemistry: Molecular Sciences, 3, 100047. https://doi.org/10.1016/j.fochms.2021.100047
- Cushman, D. W., & Cheung, H. S. (1971). Spectrophotometric assay and properties of the angiotensin-converting enzyme of rabbit lung. Biochemical Pharmacology, 20(7), 1637–1648. https://doi.org/10.1016/0006-2952(71)90292-9
- Decker, E. A., & Welch, B. (1990). Role of ferritin as a lipid oxidation catalyst in muscle food. Journal of Agricultural & Food Chemistry, 38(3), 674–677. https://doi.org/10.1021/jf00093a019
- Dikmen, C. D., Yucetepe, A., Guler, F. K., Daskaya, H., & Ozceli, B. (2017). Angiotensin-I-Converting enzyme (ACE)-inhibitory peptides from plants. Nutrients, 9(4), 316. https://doi.org/10.3390/nu9040316
- Erdmann, K., Cheung, B. W. Y., & Schroder, H. (2008). The possible roles of food-derived bioactive peptides in reducing the risk of cardiovascular disease. The Journal of Nutritional Biochemistry, 19(10), 643–654. https://doi.org/10.1016/j.jnutbio.2007.11.010
- Ferreira, I. C. F. R., Baptista, P., Vilas-Boas, M., & Barros, L. (2007). Free-radical scavenging capacity and reducing power of wild edible mushrooms from Northeast Portugal: Individual cap and stipe activity. Food Chemistry, 100(4), 1511–1516. https://doi.org/10.1016/j.foodchem.2005.11.043
- Gao, D., Chang, T., Li, H., & Cao, Y. (2010). Angiotensin I-converting enzyme inhibitor derived from cottonseed protein hydrolysate. African Journal of Biotechnology, 9(53), 8977–8982.
- Garcia, M. C., Puchalska, P., Esteve, C., & Marina, M. L. (2013). Vegetable foods: A cheap source of proteins and peptides with antihypertensive, antioxidant and other less occurrence bioactivities. Talanta, 106, 328–349. https://doi.org/10.1016/j.talanta.2012.12.041
- Ghribi, A. M., Sila, A., Przybylski, R., Arroume, N. N., Makhlouf, I., Blecker, C., Attia, H., Dhulster, P., Bougatef, A., & Besbes, S. (2015). Purification and identification of novel antioxidant peptides from enzymatic hydrolysate of chickpea (Cicer arietinum L.) protein concentrate. Journal of Functional Foods, 12, 516–525. https://doi.org/10.1016/j.jff.2014.12.011
- Girish, K., Kemparaju, K., Nagaraju, S., & Vishwanath, B. (2009). Hyaluronidase inhibitors: A biological and therapeutic perspective. Current Medicinal Chemistry, 16(18), 2261–2288. https://doi.org/10.2174/092986709788453078
- Guang, C., & Phillips, R. D. (2009). Plant food-derived angiotensin I converting enzyme inhibitory peptides. Journal of Agricultural & Food Chemistry, 57(12), 5113–5120. https://doi.org/10.1021/jf900494d
- Gupta, N., & Bhagyawant, S. S. (2018a). Angiotensin-I converting enzyme (ACE-I) inhibitory and antiproliferative potential of chickpea seed protein hydrolysate. Annals of Plant Sciences, 7(3), 2149–2153. https://doi.org/10.21746/aps.2018.7.3.10
- Gupta, N., & Bhagyawant, S. S. (2019a). Enzymatic treatment improves ACE-I inhibition and antiproliferative potential of chickpea. Vegetos, 32(3), 363–369. https://doi.org/10.1007/s42535-019-00031-6
- Gupta, N., & Bhagyawant, S. S. (2019b). Impact of hydrolysis on functional properties, antioxidant, ACE-I inhibitory and antiproliferative activity of Cicer arietinum and Cicer reticulatum hydrolysates. Nutrire, 44(1), 5. https://doi.org/10.1186/s41110-019-0095-4
- Gupta, N., & Bhagyawant, S. S. (2021). Bioactive peptide of Cicer arietinum L. induces apoptosis in human endometrial cancer via DNA fragmentation and cell cycle arrest. 3 Biotech, 11(2), 63. https://doi.org/10.1007/s13205-020-02614-6
- Gupta, N., Bisen, P. S., & Bhagyawant, S. S. (2018b). Chickpea lectin inhibits human breast cancer cell proliferation and induces apoptosis through cell cycle arrest. Protein and Peptide Letters, 25(5), 492–499. https://doi.org/10.2174/0929866525666180406142900
- Gupta, N., Gautam, A. K., & Bhagyawant, S. S. (2018c). Biochemical characterisation of lectin from wild chickpea (Cicer reticulatum L.) with potential inhibitory action against human cancer cells. Journal of Food Biochemistry, 43(2), e12712. https://doi.org/10.1111/jfbc.12712
- Gupta, N., Quazi, S., Jha, S. K., Siddiqi, M. K., Verma, K., Sharma, S., Khan, R. H., & Bhagyawant, S. S. (2022). Chickpea peptide: A nutraceutical molecule corroborating neurodegenerative and ACE-I inhibition. Nutrients, 14(22), 4824. https://doi.org/10.3390/nu14224824
- Gupta, N., Shrivastava, N., & Bhagyawant, S. S. (2017). Multivariate analysis based on nutritional value, antinutritional profile and antioxidant capacity of forty chickpea genotypes grown in India. Journal of Nutrition & Food Sciences, 7(3), 3. https://doi.org/10.4172/2155-9600.1000600
- Gupta, N., Shrivastava, N., Singh, P. K., & Bhagyawant, S. S. (2016). Phytochemical evaluation of moth bean (Vigna aconitifolia L.) seeds and their divergence. Biochemistry Research International, 2016, 1–6. https://doi.org/10.1155/2016/3136043
- Gupta, N., Srivastava, N., Bhagyawant, S. S. (2018d). Vicilin—A major storage protein of mungbean exhibits antioxidative potential, antiproliferative effects and ACE inhibitory activity. PLOS ONE, 13(2), e0191265. https://doi.org/10.1371/journal.pone.0191265
- Hong, F., Ming, L., Yi, S., Zhanxia, L., Yongquan, W., & Chi, L. (2008). The antihypertensive effect of peptides: A novel alternative to drugs. Peptides, 29(6), 1062–1071. https://doi.org/10.1016/j.peptides.2008.02.005
- Hong, L. G., Wei, L. G., Liu, H., & Hui, S. Y. (2005). Mung-bean protein hydrolysates obtained with alcalase exhibit angiotensin I-converting enzyme inhibitory activity. Food Science & Technology International, 11(4), 281–287. https://doi.org/10.1177/1082013205056781
- Huang, D. J., Chen, H. J., How, W. C., Lin, C. D., & Lin, Y. H. (2004). Active recombinant thioredoxin h protein with antioxidant activities from sweet potato (Ipomoea batatas [L.] lam Tainong 57) storage roots. Journal of Agricultural & Food Chemistry, 52(15), 4720–4724. https://doi.org/10.1021/jf0498618
- Jamdar, S., Rajalakshmi, V., Pednekar, M., Juan, F., Yardi, V., & Sharma, A. (2010). Influence of degree of hydrolysis on functional properties, antioxidant activity and ACE inhibitory activity of peanut protein hydrolysate. Food Chemistry, 121(1), 178–184. https://doi.org/10.1016/j.foodchem.2009.12.027
- Jung, M., Kim, S., & Kim, S. (1995). Riboflavin-sensitized photooxidation of ascorbic acid: Kinetics and amino acid effects. Food Chemistry, 53(4), 397–403. https://doi.org/10.1016/0308-8146(95)99834-M
- Kim, N. (2017). Production of wheat gluten hydrolyzates by enzymatic process at high pressure. Food Science & Biotechnology, 26(6), 1587–1593. https://doi.org/10.1007/s10068-017-0152-9
- Koleva, I. I., van Beek, T. A., Linssen, J. P. H., de Groot, A., & Evstatieva, L. N. (2002). Screening of plant extracts for antioxidant activity: A comparative study on three testing methods. Phytochemical Analysis: PCA, 13(1), 8–17. https://doi.org/10.1002/pca.611
- Laemmli, U. K. (1970). Cleavage of structural proteins during the assembly of the head of bacteriophage T4. Nature, 227(5259), 680–685. https://doi.org/10.1038/227680a0
- Li, Y., Jiang, B., Zhang, T., Mu, W., & Liu, J. (2008). Antioxidant and free radical-scavenging activities of chickpea protein hydrolysate (CPH). Food Chemistry, 106(2), 444–450. https://doi.org/10.1016/j.foodchem.2007.04.067
- Manzoor, M., Singh, J., & Gani, A. (2022). Exploration of bioactive peptides from various origin as promising nutraceutical treasures: In vitro, in silico and in vivo studies. Food Chemistry, 373, 131395. https://doi.org/10.1016/j.foodchem.2021.131395
- Markland, F. S., & Smith, E. L. (1971). Subtilisins: Primary structure, chemical and physical properties. In P. D. Boyer (Ed.), The enzyme (Vol. 1, pp. 561–608). Academic Press.
- McClements, D. J., & Decker, E. A. (2000). Lipid oxidation in oil-in-water emulsions: Impact of molecular environment on chemical reactions in heterogeneous food systems. Journal of Food Science, 65(8), 1270–1282. https://doi.org/10.1111/j.1365-2621.2000.tb10596.x
- Mine, Y. (2010). Bioactive proteins and peptides as functional foods and nutraceuticals. Wiley-Blackwell.
- Moure, A., Domínguez, H., & Parajó, J. C. (2006). Antioxidant properties of ultrafiltration-recovered soy protein fractions from industrial effluents and their hydrolysates. Process Biochemistry, 41(2), 447–456. https://doi.org/10.1016/j.procbio.2005.07.014
- Nielsen, P., Petersen, D., & Dambmann, C. (2001). Improved method for determining food protein degree of hydrolysis. Journal of Food Science, 66(5), 642–646. https://doi.org/10.1111/j.1365-2621.2001.tb04614.x
- Perera, H. D. S. M., Samarasekera, J. K. R. R., Handunnetti, S. M., Weerasena, O. V. D. S. J., Weeratunga, H. D., Jabeen, A., & Choudhary, M. I. (2018). In vitro pro-inflammatory enzyme inhibition and anti-oxidant potential of selected sri lankan medicinal plants. BMC Complementary and Alternative Medicine, 18(1), 271. https://doi.org/10.1186/s12906-018-2335-1
- Pihlanto, A. (2006). Antioxidative peptides derived from milk proteins. International Dairy Journal, 16(11), 1306–1314. https://doi.org/10.1016/j.idairyj.2006.06.005
- Qian, Z. J., Jung, W. K., & Kim, S. K. (2008). Free radical scavenging activity of a novel antioxidative peptide purified from hydrolysate of bullfrog skin, Rana catesbeiana Shaw. Bioresource Technology, 99(6), 1690–1698. https://doi.org/10.1016/j.biortech.2007.04.005
- Qin, L., Zhu, B. W., Zhou, D. Y., Wu, H. T., Tan, H., Yang, J. F., Li, D. M., Dong, X. P., & Murata, Y. (2010). Preparation and antioxidant activity of enzymatic hydrolysates from purple sea urchin (Strongylocentrotus nudus) gonad. LWT Food Science and Technology, 44(4), 1113–1118. https://doi.org/10.1016/j.lwt.2010.10.013
- Rivero-Pino, F. (2023). Bioactive food-derived peptides for functional nutrition: Effect of fortification, processing and storage on peptide stability and bioactivity within food matrices. Food Chemistry, 406, 135046. https://doi.org/10.1016/j.foodchem.2022.135046
- Rudrapal, M., Eltayeb, W. A., Rakshit, G., El-Arabey, A. A., Khan, J., Aldosari, S. M., Alshehri, B., & Abdalla, M. (2023). Dual synergistic inhibition of COX and LOX by potential chemicals from Indian daily spices investigated through detailed computational studies. Scientific Reports, 13(1), 8656. https://doi.org/10.1038/s41598-023-35161-0
- Rudrapal, M., Kirboga, K. K., Abdalla, M., & Maji, S. (2024). Explainable artificial intelligence-assisted virtual screening and bioinformatics approaches for effective bioactivity prediction of phenolic cyclooxygenase-2 (COX-2) inhibitors using PubChem molecular fingerprints. Molecular Diversity. https://doi.org/10.1007/s11030-023-10782-9
- Rudrapal, M., Vallinayagam, S., Aldosari, S., Khan, J., Albadrani, H., Al-Shareeda, A., & Kamal, M. (2023). Valorization of Adhatoda vasica leaves: Extraction, in vitro analyses and in silico approaches. Frontiers in Nutrition, 10. https://doi.org/10.3389/fnut.2023.1161471
- Rutherfurd, S. M. (2010). Methodology for determining degree of hydrolysis of proteins in hydrolysates: A review. Journal of AOAC International, 93(5), 1515–1522. https://doi.org/10.1093/jaoac/93.5.1515
- Sahasrabud, A., & Deodhar, M. (2010). Anti-hyaluronidase, anti-elastase activity of Garcinia indica. International Journal of Botany, 6(3), 299–303. https://doi.org/10.3923/ijb.2010.299.303
- Samaranayaka, A. G. P., & Li-Chan, E. C. Y. (2011). Food-derived peptidic antioxidants: A review of their production, assessment, and potential applications. Journal of Functional Foods, 3(4), 229–254. https://doi.org/10.1016/j.jff.2011.05.006
- Sanchez-Vioque, R., Clemente, A., Vioque, J., Bautista, J., & Millan, F. (1999). Protein isolates from chickpea (Cicer arietinum L.): Chemical composition, functional properties and protein characterization. Food Chemistry, 64(2), 237–243. https://doi.org/10.1016/S0308-8146(98)00133-2
- Segura-Campos, M. R., Chel-Guerrero, L. A., & Betancur-Ancona, D. A. (2010). Angiotensin-I converting enzyme inhibitory and antioxidant activities of peptide fractions extracted by ultrafiltration of cowpea Vigna unguiculata hydrolysates. Journal of the Science of Food & Agriculture, 90(14), 2512–2518. https://doi.org/10.1002/jsfa.4114
- Shahidi, F., & Zhong, Y. (2015). Measurement of antioxidant activity. Journal of Functional Foods, 18, 757–781. https://doi.org/10.1016/j.jff.2015.01.047
- Tang, C. H., Wang, X. S., & Yang, X. Q. (2009). Enzymatic hydrolysis of hemp (cannabis sativa L.) protein isolate by various proteases and antioxidant properties of the resulting hydrolysates. Food Chemistry, 114(4), 1484–1490. https://doi.org/10.1016/j.foodchem.2008.11.049
- Terashima, M., Oe, M., Ogura, K., & Matsumura, S. (2011). Inhibition strength of short peptides derived from an ACE inhibitory peptide. Journal of Agricultural & Food Chemistry, 59(20), 11234–11237. https://doi.org/10.1021/jf202902r
- Udenigwe, C. C., & Aluko, R. E. (2012). Food protein-derived bioactive peptides: Production, processing, and potential health benefits. Journal of Food Science, 77(1), 11–24. https://doi.org/10.1111/j.1750-3841.2011.02455.x
- Wang, W., & Mejia, E. G. D. (2005). A new frontier in soy bioactive peptides that may prevent-related chronic diseases. Comprehensive Reviews in Food Science and Food Safety, 4(4), 63–78. https://doi.org/10.1111/j.1541-4337.2005.tb00075.x
- Xie, Z., Huang, J., Xu, X., & Jin, Z. (2008). Antioxidant activity of peptides isolated from alfalfa leaf protein hydrolysate. Food Chemistry, 111(2), 370–376. https://doi.org/10.1016/j.foodchem.2008.03.078
- Yamaguchi, T., Takamura, H., Matoba, T., & Terao, J. (1998). HPLC method for evaluation of the free radical-scavenging activity of foods by using 1,1-diphenyl-2-picrylhydrazyl. Bioscience Biotechnology and Biochemistry, 62(6), 1201–1204. https://doi.org/10.1271/bbb.62.1201
- Ying, X., Agyei, D., Udenigwe, C., Adhikari, B., & Wang, B. (2021). Manufacturing of plant-based bioactive peptides using enzymatic methods to meet health and sustainability targets of the sustainable development goals. Frontiers in Sustainable Food Systems, 5, 1–22. https://doi.org/10.3389/fsufs.2021.769028
- You, L., Zhao, M., Cui, C., Zhao, H., & Yang, B. (2009). Effect of degree of hydrolysis on the antioxidant activity of loach (Misgurnus anguillicaudatus) protein hydrolysates. Inno and Food Sci and Emergency, 10(2), 235–240. https://doi.org/10.1016/j.ifset.2008.08.007
- Yust, M. M., Pedroche, J., Girón-Calle, J., Alaiz, M., Millán, F., & Vioque, J. (2003). Production of ace inhibitory peptides by digestion of chickpea legumin with alcalase. Food Chemistry, 81(3), 363–369. https://doi.org/10.1016/S0308-8146(02)00431-4
- Zhu, Z. B., Qiu, N. X., & Yi, J. H. (2010). Production and characterization of angiotensin converting enzyme (ACE) inhibitory peptides from apricot (Prunus armeniaca L.) kernel protein hydrolysate. European Food Research & Technology, 231(1), 13–19. https://doi.org/10.1007/s00217-010-1235-5