ABSTRACT
The human intestinal commensal microbiota and associated metabolic products have long been regarded as contributors to host health. As the identity and activities of the various members of this community have become clearer, newly identified health-associated bacteria, such as Faecalibacterium prausnitzii, Akkermansia muciniphila, Ruminococcus bromii and Roseburia species, have emerged. Notably, the abundance of many of these bacteria is inversely correlated to several disease states. While technological and regulatory hurdles may limit the use of strains from these taxa as probiotics, it should be possible to utilize prebiotics and other dietary components to selectively enhance their growth in situ. Dietary components of potential relevance include well-established prebiotics, such as galacto-oligosaccharides, fructo-oligosaccharides and inulin, while other putative prebiotics, such as other oligosaccharides, polyphenols, resistant starch, algae and seaweed as well as host gut metabolites such as lactate and acetate, may also be applied with the aim of selectively and/or differentially affecting the beneficial bacterial community within the gastrointestinal environment. The present review provides an overview of the dietary components that could be applied in this manner.
Introduction
The human gut is estimated to contain approximately 1014 bacteria comprising more than 1000 species.Citation1,Citation2 Intestinal commensal bacteria, as well as their metabolic products, have long been recognized as important contributors to host health, including nutrition/energy homeostasis, pathogen resistance, the regulation of intestinal epithelial proliferation and a variety of other factors.Citation3 The corollary is that negative impacts on microbiota composition and functionality can contribute to illness.Citation4,Citation5 Importantly, the gut microbiota can be modulated in a beneficial way, including through the selective manipulation of particular species of interest to maintain, restore or improve host health.
One approach to positively modulate the gut microbiota is through the administration of growth enhancing substrates that can be utilized selectively by health promoting bacteria, to encourage their growth and the production of associated desirable metabolites. The rationale of selectively enhancing beneficial microbes in the gut lead to the concept of prebiotics, initially coined in 1995 by Roberfroid and GibsonCitation6 and described in greater detail below. Among the best characterized prebiotics are galacto-oligosaccharides (GOS) and inulin and its oligomer, fructo-oligosaccharides (FOS), as evidenced by numerous studies.Citation7–Citation9 The vast majority of studies relating to these and other prebiotics have focused on elucidating their effects on Bifidobacterium and Lactobacillus as strains from these genera have been known for some time to confer a health benefit to the host.Citation10 As our knowledge of the gastrointestinal microbiota expands, emphasis has now also been placed on the application of other bacteria present with possible health promoting effects and, in parallel, on the identification of prebiotics (targeted) and other dietary substrates (potentially less targeted) with the potential to stimulate the growth of these beneficial targets in the gut.
Newly identified health-associated microbes
Advances made in metagenomics and the application of emerging ‘multiomics’ technologies, in combination with cultivation techniques, have identified several putatively beneficial species of gut bacteria (). These microbes, including Faecalibacterium prausnitzii, Ruminococcus bromii and Akkermansia muciniphila, can be present in significant numbers in a healthy human gut. A single species such as F. prausnitzii may comprise more than 5% of the total intestinal community,Citation30 while there are also instances where up to 8% of the composition has been assigned to the phylum Verrucomicrobia,Citation31 i.e., corresponding to A. muciniphila at the species level.
Table 1. Overview of some representative newly identified health-promoting bacteria and the associated benefits.
Some of these newly identified health-promoting bacteria are associated with various benefits to their respective hosts. One example of such an apparent relationship is the observation that the abundance of F. prausnitzii is inversely correlated with incidence of inflammatory bowel disease (IBD), in particular Crohn’s disease.Citation19,Citation23,Citation32 As a result, F. prausnitzii has been investigated with respect to its potential to alleviate inflammation.Citation32 Another intriguing host-microbe interaction relates to that between A. muciniphila and obesity. Accumulating evidence has shown that the abundance of A. muciniphila is inversely proportional to body weight and type-1 diabetes.Citation27,Citation33 A. muciniphila is specialized as it colonizes the mucus lining and can use mucin as a sole carbon and nitrogen source, releasing growth substrates for other beneficial bacteria.Citation34,Citation35 Interestingly, in a recent study, Roseburia hominis and Roseburia intestinalis have also been found to utilize mucin as energy source indicating that mucin degradation is more widespread than was previously appreciated.Citation36 Mucin is a major component of intestinal mucus layer. The ability to utilize mucin offers an ecological benefit to these bacteria over those that are dependent on dietary nutrients, providing a source of host-derived nutrients while the geographical location provides a means of interacting with the immune system.Citation37 Notably, Christensenella minuta and Oscillospira spp. have also been linked with a lean phenotype and may be useful targets when combating obesity.Citation11,Citation24,Citation28 The establishment of potential relationships such as these support the continued efforts in identifying approaches to encourage the growth of target microbes in the gut.
The breakdown and metabolism of complex carbohydrates, particularly non-digestive carbohydrates, by gut bacteria, hugely benefits primary degraders as well as the community which lack the ability to use the parent components. R. bromii has been suggested to be a key species with respect to the breakdown of resistant starch, with the resultant products being utilized as substrates for syntrophic growth by other beneficial microbes in the gut.Citation25
While in some instances the benefits attributed to specific gut microbes are thought to be direct, in other cases they can be indirect, such as via their metabolites. This can include the ability to utilize fibers and complex carbohydrates to produce short chain fatty acids (SCFAs),Citation23,Citation25,Citation27 the most abundant of which are acetate, propionate and butyrate. SCFAs have numerous benefits including; lowering the luminal pH, contributing to increased calcium and magnesium absorption, helping to reduce potential pathogenic bacteria, serving as an energy source for epithelial cells and also involvement in immune modulation. Butyrate, as mentioned previously, is produced mainly by components of the major phylum, Firmicutes, such as Clostridium clusters XIVa and IV,Citation38 and is particularly important as it is utilized as an energy source for colonocytes and has a proposed role in protection against diseases such as colon cancer and colitis.Citation39–Citation42 It is therefore important that these health-promoting bacteria are present in sufficient numbers and are maintained within the gut.
Other species that are abundant in a healthy gut, including Lachnospiraceae such as Anaerostipes caccae, Anaerostipes hadrus, Roseburia spp., Coprococcus catus, and Eubacteriaceae such as Eubacterium rectale and Eubacterium hallii, are acetate consumers and produce butyrate via butyryl-CoA:acetate CoA-transferase,Citation43 which will be discussed in more detail later. There are a number of other examples of substrate cross-feeding that can have a substantial impact on the survival of certain bacteria and production of useful metabolites in the gut.Citation44 For instance, lactate is readily available in the gut as a result of the activity of lactic acid bacteria and can be utilized by Anaerostipes sp. and Eubacterium sp. to produce SCFAs.Citation45 Similarly, C. catus and the ruminal bacterium Megasphaera elsdenii can convert lactate to propionate via the acrylate pathway.Citation20 The metabolic diversity and dependency of these health associated microbes is complex and, while our understanding of the pathways involved in carbohydrate fermentation and cross-feeding are improving (), there is likely to be still much to learn.
Figure 1. Schematic representation of selected gut bacteria involved in carbohydrate fermentation and cross-feeding interactions resulting in the production of major microbial metabolites.
Pathways leading to the production of the three main SCFAs, acetate, butyrate and propionate, are depicted here. Acetate can be produced from acetyl-CoA or by acetogens using H2 and CO2 or formate. It can also be used for the formation of butyrate. Butyrate can be formed in two ways; either through the formation of butyryl-P or more commonly through the use of butyryl-CoA:acetate CoA-transferase which many Firmicutes possess. The main route by which propionate is generated is via the succinate pathway. However, two other pathways have also been found; i.e., the acrylate pathway which involves lactate and the propanediol pathway which utilizes deoxyhexose sugars. DHAP, dihydroxyacetonephospate; PEP, phosphoenolpyruvate. Adapted from Louis et al., 2014.Citation43
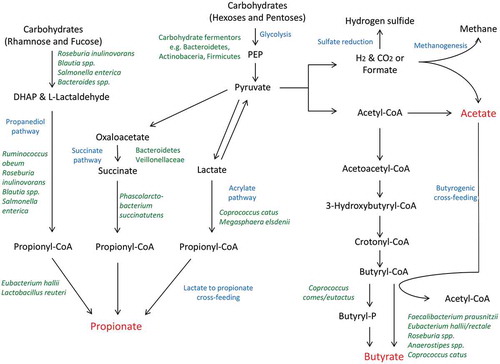
Prebiotic criteria and considerations
A large number of studies have been conducted to investigate a range of prebiotics and the effects that they have on the gut microbiota. With growing interest and knowledge, the definition of prebiotics has evolved and in December 2016, the International Scientific Association for Probiotics and Prebiotics (ISAPP) proposed that the definition be revised to ‘a substrate that is selectively utilized by host microorganisms conferring a health benefit’.Citation46 This definition expands the concept to include non-carbohydrate substances. It also was suggested that the revised definition does not limit the selective stimulation of beneficial microorganisms to the gut if in the future prebiotic targets are beyond this environment. Currently, in order to be classified as a dietary prebiotic, three broad criteria need to be fulfilled. These include (i) resistance to gastric acid and hydrolysis by mammalian enzymes and gastrointestinal absorption; (ii) ability to be utilized by the intestinal microbiota; and (iii) selectively stimulate the growth and/or the activity of intestinal bacteria associated with health-promoting effects.Citation47
A major prerequisite to the success of a particular prebiotic is that the target bacteria must be present or present at a specific threshold. If levels are below a certain threshold, due to factors such as ageing or antibiotic usage, then the prebiotic may not be effective in increasing the numbers of these desirable bacteria to the necessary level in order to confer the anticipated health benefits.Citation26 Thus, having an understanding of such factors in target populations can enhance the likelihood of beneficial prebiotic-related outcomes. However, there are a number of other factors to consider. While prebiotics, and other beneficial food bioactives, can enable the growth of targeted health-promoting bacteria, more detailed microbiome analysis has highlighted that these substrates are not always as specific as once thought.Citation46–Citation48 It is also necessary to consider that the benefits of prebiotics can sometimes be secondary, e.g., production by health promoting bacteria of metabolic products that can inhibit the growth of enteric pathogens and/or attenuate their virulence.Citation49 One can determine prebiotic impacts/specificity through the use of ex vivo fermentation studies, with fecal samples or mock gut microbial communities, or through in vivo studies. However, even then, care should be taken when interpreting outcomes as, due to inter-individual differences, the same effect may not be observed in all cases. It is also important to consider that some populations, when present at sufficiently high levels, may produce gases from prebiotic substrates that can result in bloating and abdominal discomfort.Citation50,Citation51 Additionally, some prebiotics such as inulin-derived oligosaccharides can have mildly laxative effects.Citation52 All of these considerations highlight the importance of understanding the interactions between prebiotics (as well as other dietary components), target microbes and the metabolites generated in order to determine ultimate resultant impacts on the host.
Targeting newly identified health-associated microbes through well-established prebiotics
There are a few extensively studied prebiotics, including FOS, GOS and inulin, which have been examined in detail using in vitro assays and in vivo animal models and human studies. These investigations have in particular highlighted the growth-promoting impact of prebiotics on members of the genera Bifidobacterium and Lactobacillus,Citation6,Citation9,Citation46,Citation53 as a consequence of the health-associated status of many strains from these taxa. However, there are many ways in which prebiotics can positively impact the host (). A direct benefit of prebiotics is enhancing the growth of target micro-organisms which in turn compete with harmful species for energy sources and exclude them proving protection or through facilitating the production of beneficial fermentation products, such as SCFAs.
Figure 2. An overview of some beneficial impacts of prebiotic supplementation on the gut microbiota.
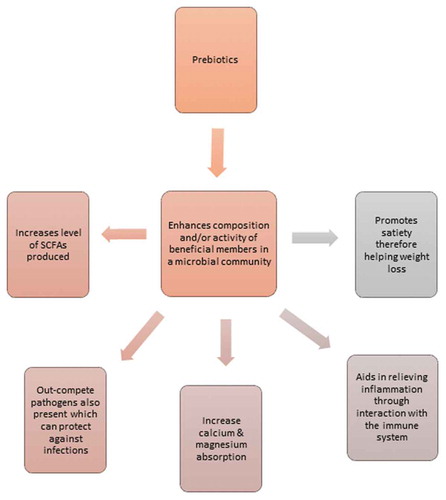
Clinical trials and human studies are pivotal when assessing the benefits of newly identified prebiotics. Of the many studied potential prebiotics, only a few substrates, including inulin, FOS and GOS, have been validated through several human studies and only those that looked at a broader gut community, and thus are of greater relevance to this review, are presented in . These studies have highlighted that the extensively examined prebiotics, for example inulin, can also have a positive effect on the level of F. prausnitzii and Anaerostipes sp. within the gut, which may explain some of the butyrogenic effects that ensue when inulin is consumed.Citation53,Citation56,Citation57 Likewise, FOS and GOS have also been demonstrated to enhance F. prausnitzii levels.Citation53,Citation55,Citation56 Of the few human studies assessing the impact of prebiotics on microbial diversity in the colon, contradictory observations with regards to SCFA levels have been reported. A study by Liu et al.Citation54 noted a decrease in the levels of butyrate producers and increase in the levels of Bifidobacterium after administration of FOS and GOS in a healthy population, which may be caused by high levels of lactic acid being produced, therefore making the environment inhospitable for butyrate producers. It is also worth noting that this intervention was conducted for a period of 14 days; longer intervention studies are needed to better evaluate the effects of prebiotic administration. Nevertheless, these results prove intriguing and emphasize the need for more human studies.
Table 2. Human studies conducted in relation to prebiotics and newly identified health-promoting bacteria.
Targeting newly identified health-associated microbes through emerging prebiotics
The prebiotic paradigm has been shifting in recent years as a result of the identification of newly recognized putatively beneficial members of the gut microbiota to target for enrichment. These studies have been facilitated through new developments in cultivation techniques and, in particular, high-throughput sequencing, culture-independent approaches. These approaches have been used to assess the impact of specific fibers as well as polyphenols, other oligosaccharides and the less explored marine derived foods including seaweed-based products, which represents a relatively untapped source of food bioactives, on the gut microbiota of animals and humans.
Polyphenols
Polyphenols are naturally occurring secondary metabolites of plants that consist of a wide category of compounds and are classified primarily based on structure.Citation63 Plant-based foods and beverages, including fruits, vegetables, coffee, tea and wine, are rich sources of dietary polyphenols. Consumption of plant foods is associated with lower risk of chronic diseases including cancer,Citation64 heart diseases,Citation65 type 2 diabetesCitation66 and other inflammatory diseases. A large number of these biological effects are attributed to phytochemical components of plant foods.Citation67,Citation68 Moreover, it has been suggested that many of the beneficial impacts of these secondary metabolites on overall health is mediated through the manipulation of gut bacteria in the colon and their transformation therein by the gut bacteria present.Citation69 One such example is cranberry extract, which is rich in polyphenols, and has been shown to ameliorate diet-induced obesity and several features of metabolic syndrome (MetS) in mice, while increasing the abundance of A. muciniphila.Citation70 Cranberry administration has also been shown to enhance mucus secretion, which could possibly create favorable conditions for A. muciniphila.Citation71 Similarly, the administration of Concord grape and California table grape extracts, rich in a class of polyphenol called proanthocyanidin, also increases the abundance of Akkermansia.Citation72,Citation73 Other polyphenols present in red wine, tea and cocoa flavonols have also been investigated. Cocoa flavanols increase the levels of lactobacilli and bifidobacteria in addition to enhancing levels of the Clostridium coccoides-Eubacterium rectale group subtly.Citation74 From a red wine perspective, intake of 272 mL per day over a 30-d period altered the composition of the gut microbiota in patients with MetS and resulted in increased levels of Bifidobacterium, Lactobacillus, F. prausnitzii and Roseburia sp.Citation58 The study also suggests that the increase in F. prausnitzii levels was associated with the decrease of blood glucose levels in MetS patients possibly due to the production of SCFAs interacting with gut hormones which in turn may have an impact on carbohydrate metabolism.Citation58 In the same study, beneficial modulatory impacts in healthy volunteers were attributed to red wine polyphenols as levels of Blautia coccoides – Eubacterium rectale group, bifidobacteria, Bacteroides uniformis and Eggerthella lenta were enhanced. Consequently, there was a significant decrease in the levels of the potentially harmful Clostridium histolyticum group, which encompasses some pathogens such as Clostridium perfringens. Although the exact mechanisms involved are not fully understood, these in vitro and human studies form the basis of utilizing certain polyphenols, alone or in combination, to alter the composition of the gut microbiota.
Oligosaccharides
In addition to the well-studied oligosaccharides, FOS and GOS, more recently there have been many studies focusing on the prebiotic effect of other oligosaccharides, such as xylo-oligosaccharides (XOS) and arabinoxylan (AX), pectin-oligosaccharides (POS), isomatlooligosaccharides (IMO), soybean oligosaccharides and human milk oligosaccharides (HMOs).
XOS is the product of the hydrolysis of xylan and can act as a substrate for gut bacteria, resulting in the production of SCFAs,Citation75 with associated health benefits. The selectivity of XOS was noted in a study where 6 out of 11 Firmicutes examined, including R. intestinalis, R. faecis, E. rectale and A. caccae, were able to utilize this substrate, along with some Bifidobacterium sp. such as B. adolescentis.Citation76 A number of studies have highlighted that XOS can have a bifidogenic effect. In one instance, a human study evaluated the impact of XOS alone and XOS in conjunction with inulin. XOS alone increased fecal Bifidobacterium and butyrate, with a decrease in acetate and p-cresol and the combination of substrates appeared to reduce the inflammatory repercussions of a Western diet.Citation77 XOS supplementation was also determined to increase Faecalibacterium sp. and Akkermansia sp. as well as bifidobacteria but did not have a significant impact on levels of lactobacilli.Citation61 This study demonstrated that XOS promoted intestinal health through modulation of the microbial community; although there was no notable net increase in SCFA production detected, due consideration must be taken when examining dose effects.
Arabinoxylan (AX), a non-digestible carbohydrate often found in the cell walls of plants, can be selectively fermented in the colon by fibrolytic gut bacteria possessing AX-degrading enzymes.Citation78 AX can be hydrolyzed to arabinoxylan-oligosaccharides (AXOS). Both AX and AXOS have demonstrated an ability to increase desirable bacteria and butyrate producers in the colon.Citation79–Citation81 Indeed, in one instance when a high-fat (HF) diet was administered to mice, the addition of AX subsequently increased levels of bifidobacteria.Citation80 AX also restored bacterial levels to that of the initial control level before HF diet induced obesity, in particular enhancing populations of the Roseburia species and Bacteroides-Prevotella species that were reduced upon HF feeding. These microbial shifts as a result of AX supplementation highlight its prebiotic potential.
Pectin and pectic oligosaccharides (POS) have been identified as emerging prebiotics as evidenced through their selective utilization by certain members of the colonic microbiota such as E. eligens and F. prausnitzii.Citation12,Citation82 The most frequent source of pectin is from citrus fruits and apple pulp, but it is also abundant in agricultural by-products such as sugar beet pulp. POS can be obtained through depolymerization of pectin and both pectin and POS escape host digestion and reach the distal colon when consumed.Citation83 In an in vitro assay of F. prausnitzii growth in the presence of different substrates, it was ascertained that growth was enhanced with apple pectin in most cases.Citation12,Citation84 Although pectin is extensively fermented in the gut, pectin utilization has not been reported for many of the bacterial groups residing in the gut. It has been demonstrated that one of the main fermentation products from pectin utilization by F. prausnitzii was butyrate. The ability of F. prausnitzii to compete for pectin when cultured with B. thetaiotaomicron and E. eligens was also noted.Citation12 Growth on some uronic acids such as galactouronic acid was also observed in F. prausnitzii. This is important as galactouronic acid is a major component of pectin and it is not reported to be utilized by many other bacterial groups other than Bacteroides sp.Citation12 The impact of sugar beet pulp and lemon peel wastes on gut microbial communities have also been assessed using in vitro fermentation assays.Citation85 Results suggested that POS of both lemon peel and sugar beet possessed better prebiotic potential than the corresponding pectin, and similar or even better than FOS (which was used as a control). Lemon peel waste oligosaccharides (LPOS) in particular resulted in an increased level of Faecalibacterium and Roseburia sp. as well as lactobacilli. Both LP and LPOS increased the level of E. rectale, although larger effects were observed with pectin. Ultimately, the study indicated that POS and pectin brought about a beneficial impact on the microbial population, presenting an intriguing opportunity for further investigation.
Isomaltooligosaccharides (IMO) are often considered potential prebiotics. Their impact on newly identified health beneficial microbes is limited and necessitates further investigation. IMOs are naturally found in foods such as honey as well as fermented foods such as miso and soy. In addition, various commercial preparations are made enzymatically by processing an assortment of starches and are readily available on the market today. Commercially available IMOs are composed of a mixture of α(1–6) and α(1–4)-linked glucosyl oligosaccharides.Citation86 One of the glucose oligomers identified in IMOs is isomaltose, which is a major constituent of honey thereby giving IMOs a distinctive sweet honey taste. In a recent study evaluating the impact of co-supplementation of cranberry extract with IMO in HF diet fed mice, Roseburia, Faecalibacterium, and Eubacterium were enhanced with an associated increase in butyrate.Citation87
Unsurprisingly, HMOs have also been the focus of much attention, with strong effects being observed on Bifidobacterium levels in particular.Citation88 Breast milk is the natural first nutritional source for newborns and provides oligosaccharides that promote the growth of desirable Bifidobacterium sp. in the infant gut. HMOs and other nutrients in breast milk can act as substrates for bacteria in the gut, thereby stimulating the growth of beneficial bacteria located here. Two compounds in HMOs are 2ʹ-O-fucosyllactose and lacto-N-neotetraose, which was the subject of one human study evaluating their putative prebiotic effect on the human gut microbiota in 100 healthy, adult volunteers.Citation89 This HMO supplementation was shown to modify the gut microbiota with a resulting increase in the relative abundance of Actinobacteria and, in particular Bifidobacterium, while reducing the relative abundance of Firmicutes and Proteobacteria. While there are much data available relating the impact of HMOs on the infant gut, and of Bifidobacterium strains found therein,Citation90 their full effect on the overall infant gut microbial population, and resulting adult microbiota, is the subject of many on-going investigations.
Other oligosaccharides, including those from soybean and mannan, have putative prebiotic effects. Soybean oligosaccharides seem to have a positive influence on Bifidobacterium levels and lactate production.Citation91 Soybean forms a substantial part of diets in Asia and is now more frequently seen in Western diets, and benefits are attributed partly to fiber and the oligosaccharides present. Mannan-oligosaccharides (MOS) are commonly used as additives in animal feeds. MOS are not absorbed by the animal so can reach the gut microbiota where they are utilized beneficial bacteria such as bifidobacteria and lactobacilli which can out-compete potential pathogenic bacteria. The effect on animal intestinal bacteria and mechanisms involved is just beginning to be evaluated.Citation92 Although their effect on human gut intestinal inhabitants has yet to be assessed, there may be considerable merit in doing so. Combinatorial benefits of various oligosaccharides are also beginning to be assessed, which may lead to the identification of optimal levels and combinations of prebiotics for the promotion of health-associated bacteria in the gut.Citation93
Resistant starch
Resistant starch (RS) is a form of starch that cannot be degraded in the small intestine and, thus, subsequently reaches the large intestine where it is fermented by the colonic bacteria. There are four types of RS (type 1–4), each with different properties that contribute to resisting digestion. RS1 is physically inaccessible due to its compact structure, RS2 has a granular structure, which prevents digestive enzymes from hydrolyzing it, RS3 are retrograded starches and RS4 is modified with chemicals to enhance resistance. RS2 has been the focus of many investigations, both ex vivo and in humans. Interestingly, in a co-culture experiment, R. bromii demonstrated a unique ability to stimulate RS degradation by other bacteria.Citation25 When cultured with E. rectale, B. thetaiotaomicron and B. adolescemtis on YCFA (yeast extract-casitone-fatty acid) medium, a semi-defined medium, utilization of RS was enhanced in comparison to combinations without R. bromii. This is intriguing as it was determined that YCFA medium did not support the growth of R. bromii very well. This indicates that R. bromii has a superior ability to ferment RS and even possibly produce metabolites that are available for cross-feeding interactions with co-inhabitants. Many studies have particularly emphasized the importance of R. bromii as a keystone species with regard to degrading RS within the human gut.Citation25,Citation26,Citation94 The R. bromii population substantially increased in fecal samples collected from those consuming a high RS diet.Citation25
Venkataraman et al.Citation26 demonstrated that RS2, when used to supplement the normal diet of healthy volunteers, increased fecal butyrate concentrations albeit with considerable inter-individual differences. This high amylose starch enhanced SCFA production, although those with low initial levels of SCFAs did not increase substantially, indicating that possibly the baseline level of required bacteria was too low for the full benefits of supplementation to be achieved. In those where an effect was evident, there was a substantial increase in RS-degrading bacteria such as B. adolescentis and R. bromii with some individuals displaying an additional enhanced level of E. rectale.
Similarly, in a study evaluating the effect of RS2 on subjects with insulin resistance using a multi-omics approach, a high-RS diet resulted in an increased Firmicutes to Bacteroidetes ratio, with a particular enhancement of F. prausnitzii, R. bromii and Roseburia species.Citation62 The Firmicutes to Bacteroidetes ratio has been used, albeit somewhat controversially, as a marker of gut homeostasis with increased proportions Bacteroidetes being proposed to reflect a beneficial modulation of gut microbiota. There is evidence to suggest that different types of RS may lead to distinct compositional modulations. In a study examining the effect of RS2 or RS4 on the composition of the fecal microbiota in 10 human subjects, in line with the aforementioned studies RS2 enriched B. adolescentis, R. bromii and E. rectale.Citation95 On the other hand, RS4 increased the levels of Parabacteroides distasonis and R. bromii and decreased the levels of E. rectale. Both R. bromii and E. rectale were also enriched in fecal samples of obese human subjects during consumption of RS3 indicating some similarity to RS2.Citation96 This highlights the importance of the chemical structure of RS in dictating its impact on the colonic bacteria. A recent dietary intervention study looked at the impact of two different RS, one from potatoes and the other from maize, as well as inulin on the gut microbiota of healthy volunteers.Citation97 Both types of RS were RS2 although the resulting changes in the gut microbiota differed with the RS from potatoes yielding a greater increase in SCFA production than either RS from maize or inulin. Again, the presence of R. bromii was significant. It was noted that if either R. bromii or Clostridium charatabidum were present after consuming RS it was more likely for butyrate to be produced, especially when the microbial population included the butyrate-producer E. rectale. This indicates that there may be primary degraders of RS such as R. bromii and the breakdown products may subsequently be utilized by certain butyrate-producers. Bifidobacterium was also enhanced in most of the gut microbiotas after consuming any of the three fermentable fibers. The resulting effects of RS degradation on the microbial population has yet to be fully explored, however, it is evident that R. bromii has an integral role in initiating the breakdown of this resistant but fermentable starch. This selective metabolism is the driving force behind the ever-increasing interest in the use of RS as a prebiotic ingredient in functional foods.Citation98 Indeed, several commercialized products containing RS are already available on the market and there has been a continuing effort to incorporate the RS as a functional food component.
Algae and seaweed
The marine environment is often considered a vast, untapped reservoir with regards to the microorganisms and compounds present, the metabolites produced and interactions that occur in this ecosystem. New bioactives and potential prebiotic substrates are being harnessed from this rich environment. Seaweeds, also known as marine macroalgae, are rich in polysaccharides and bioactive compounds, which could be applied to improve human and animal health.Citation99,Citation100 Seaweeds can be subdivided into three categories based on their pigmentation; Rhodophyta (red seaweed), Chlorophyta (green seaweed) and Phaeophyta (brown seaweed).Citation99 Differences in composition and structure of the polysaccharides present in these seaweeds are also evident. Brown seaweed is of particular interest as it possesses polysaccharides such as fucoidan, laminarin and alginate.Citation101 Fucoidans comprise a class of fucose-rich sulfated polysaccharides often located in the cell walls of brown macroalgae. It is suggested that fucoidans with differing structures may impact the gut in a variety of ways. For example, a study carried out by Shang et al.,Citation102 established that fucoidans isolated from Ascophyllum nodosum (FuA) and Laminaria japonica (FuL) had positive but slightly differing effects on the murine gut microbiota due to their structural differences. FuA has a type I back-bone structure whereas FuL has a type II structure. FuL markedly increased the levels of Ruminococcaceae while FuA enhanced Lactobacillus, Anaeroplasma and Thalassospira. In a follow-up study on HF diet-fed mice, these fucoidans improved MetS induced by a HF diet.Citation103 Dietary supplementation with fucoidan decreased body weight in HF diet-fed mice and also improved glucose intolerance and insulin resistance. Both fucoidans separately ameliorated intestinal dysbiosis caused by HF diet and significantly increased Akkermansia abundance. An evolving body of evidence has linked A. muciniphila with a lean phenotype and often levels are lower in those with obesity and its related metabolic alterations. Additionally, SCFA producers such as Blautia and Alloprevotella were increased. This contributes to the improvement of the health status of the gut microbial community. Remarkably there did not seem to be any major differences between FuL and FuA in this instance as both influenced the gut bacterial community correspondingly. Thus, ingredients from marine resources, particularly fucoidan, have potential as mediators of gut microbes.
Other components influencing growth
Gut metabolites as modulators
Apart from dietary and environmental factors, the host-derived as well as microbial-derived metabolites in the gut can act as modulators of the gut ecosystem. Lactate is one of the many studied metabolites. Interest in lactate as a substrate to impact on the gut microbiota has increased in recent years due to the fact that some lactate-utilizing bacteria have the ability to produce butyrate.Citation14 Notably, E. hallii and A. caccae have demonstrated an ability to use both D- and L-lactate.Citation14 Evidence of lactate being utilized in cross-feeding studies is particularly intriguing as it may explain the butyrogenic effect of certain dietary bioactives, including resistant starch.Citation14,Citation104 Indeed, Bifidobacterium and Bacteroides who are known degraders of RS do not produce butyrate. It is hypothesized that these active starch degraders may produce lactate initially and that, subsequently, lactate utilizers such as E. hallii and A. caccae may produce butyrate using lactate as a substrate.Citation14 The synergistic effect of this cross-feeding mechanism highlights the intricate and elaborate relationships of co-inhabitants within the gut. Interestingly, synbiotic administration of A. caccae and GOS improved beneficial organic acid production in comparison with GOS alone.Citation105 Also, in a separate study, Roseburia sp. strain A2-183 was unable to consume lactate or grow on potato starch or FOS in pure culture, but when co-cultured with B. adolescentis L2–32 in the presence of starch or FOS, the bacterium produced butyrate.Citation104
As noted earlier, acetate is the most abundant SCFA in the colon and while it is normally an end-product of anaerobic fermentation, it can also be utilized by some butyrate-producing species within the gut.Citation106 For example, F. prausnitzii, one of the most abundant bacteria in the colon, is also a prominent acetate-consumerCitation14 and grows poorly on media deficient in acetate.Citation107 Along with F. prausnitzii, R. intestinalis and E. rectale are also known acetate consumers.Citation106,Citation108 Although they are unable to use acetate as a sole source of energy, they do show net utilization of acetate possibly to produce butyrate via acetyl-CoA formation. Strains of F. prausnitzii and Roseburia sp. possess butyryl-CoA:acetate-CoA transferase which is involved in catalyzing butyryl-CoA to butyrate.Citation106 This reaction may also be carried out by butyrate kinase instead of the transferase.Citation109 Other members of the intestinal microbiota may also play a role in making acetate available for utilization by other microbes present in the gut. A. muciniphila has the ability to degrade and utilize host mucus and consequently can produce 1,2-propanediol, acetate and propionate, thereby stimulating the nearby butyrate producers as a result of this specialized activity within this niche environment.Citation13 Barcenilla et al., working with human fecal samples, demonstrated that 95% of strains isolated that were net acetate utilizers were butyrate producers.Citation108 Half of butyrate-producing isolates examined in this study, however, exhibited net acetate consumption. Not all acetate, of course, is re-directed for butyrate synthesis. However, there appears to be a strong link between acetate consumption and butyrate production by colonic bacteria. Further exploration to establish to what degree acetate is required for colonic butyrate production is necessary in developing our understanding of this relationship.
N-acetylglucosamine (GlcNAc) and N-acetylgalactosamine (GalNAc) are host-derived monosaccharide derivatives of glucose and galactose, respectively, and are found abundantly in the mucus lining of the gut.Citation35 As noted, A. muciniphila is an intestinal bacterium that resides in this ecological niche.Citation29,Citation110 This beneficial bacterium is adept at colonizing the mucus lining and has the ability to utilize a number of sugars including GlcNAc and GalNAc, although optimal growth in vitro is achieved with media containing mucin.Citation35 Other components of mucin may further enhance its growth.
The more recent generation of culture-independent, sequence-based understanding of gut communities facilitates the use of computational modelling to elucidate metabolic functions possessed by a single speciesCitation22,Citation111 or microbial gut communities,Citation112 as well as predicting possible growth substrates based on the pathways present. Based on whole genome sequences, models have been developed to predict the metabolic capabilities of F. prausnitziiCitation22 and A. muciniphilaCitation111,Citation113 in silico. The ability of microbes to utilize a variety of carbohydrates, including the host-derived sugar GlcNAc, and amino acids or other growth promoters can be confirmed with cultivation experiments. In evaluating the predicted conditions, GlcNAc was found to enhance the growth of F. prausnitzii better than glucose.Citation22 This ability was also observed in several tested strains of F. prausnitzii suggesting that multiple strains can grow well on GlcNAc, highlighting its ability to utilize both diet- and host-derived substrates.Citation12 Although GlcNAc is not commonly found in foods, it is found in mushrooms as GlcNAc is a monomer of chitinCitation114 and GlcNAc supplements are also available on the market.
Minerals
Minerals, such as iron, magnesium, sodium and calcium, are essential elements for living organisms, including most bacteria, and play pivotal roles in many biological processes. They are present abundantly in many food sources and, when these foods are ingested; microbes in the gut have access to these minerals. Although a little is known about the regulation of mineral absorption mediated by gut bacteria and their impact on the gut microbiota, information on the importance of major dietary minerals on health is available. Of these, iron has sparked the interest of many researchers over the last number of years, with a focus on the impact on malnourished children. Iron deficiency is very prevalent among African children with the WHO estimating 62.3% of preschool children are anemic.Citation115 Iron-containing micronutrient powders (MNPs) are added to foods in a bid to reduce iron deficiency and prevent anemia in children but the impact of iron supplementation on gut microbiota composition and functions has yielded conflicting results.Citation116–Citation119 These differences might be partly due to age-related differences in various cohorts, diverse geographical settings, the variety of iron compounds involved and the doses administered in the different studies, which are summarized below.
The impact iron exerts on the production of bacterial metabolites, in particular butyrate, has also been the focus of some investigations. Butyrate seems to be strongly affected in response to iron. In one in vitro colonic fermentation model, moderately low levels of iron increased butyrate production whereas very low levels impaired its production.Citation119 The same study found that under high iron conditions, an increase in abundance of propionate-producing Bacteroidaceae and a decrease in butyrate-producing Lachnospiraceae were reflected based on the metabolites produced. Butyrate levels produced by R. intestinalis in batch culture were negatively affected by low iron levels while lactate and formate were enhanced. The results were reversed with high iron levels suggesting that levels of a single mineral can have a big impact on gut microbiota. However, some recent data has indicated that iron supplementation has adverse effects on the gut microbiota.Citation116,Citation117,Citation120 Notably iron supplementation resulted in decreased levels of desirable bacterial groups such as Bifidobacteriaceae and Lactobacillus and increased levels of Enterobacteriaceae. The production of siderophores in many pathogenic bacteria may have been responsible for the latter phenomenon.Citation116 One intriguing study by Paganini et al.Citation120 involving three groups of Kenyan infants demonstrated that the addition of GOS with a MNP containing iron (FeGOS group) mitigated the adverse effects observed in comparison to children that were administered the MNP and iron without the prebiotic (Fe group). The FeGOS group exhibited a similar gut microbiota composition to the control group (MNP without iron or GOS) but had lower levels of virulence and toxin genes (VTGs) and increased production of SCFAs. On the other hand, a shift in microbiota composition to a more adult-like community was observed in the Fe group. Thus, the prebiotic seemed to stabilize the gut microbial community by limiting the negative effects induced by the addition of iron. Further, the addition of GOS did not seem to affect the bioavailability of iron. The MNP used in this study was fortified with 5 g of iron, i.e., much lower than the average amount of 12.5 g used in standard MNP supplements in earlier studies.Citation121 The lower dose maintained efficacy in that the iron was of high bioavailability and reduced anemia so therefore may be more suitable than the current high dose levels which are between 10 and 12.5 mg of fortified iron according to the WHO.Citation122 As noted, the varying doses and iron compounds investigated throughout the literature highlight a need for standardization in supplementation regimes. However, achieving this across different cohorts is difficult as many factors contribute to iron bioavailability and differences in microbiota across different populations makes it difficult to establish the complete impact of iron.
The effect of other minerals on the gut microbiota, in particular bacteria recently identified as beneficial, is currently not well understood. While there are some insights to suggest that other minerals, such as zinc, can have an impact,Citation123,Citation124 little is currently known.
Synbiotics
Synbiotics have also attracted significant attention in recent years. Synbiotics are combinations of a probiotic and an appropriate prebiotic designed to enhance specifically the growth and survival of the probiotic or other desirable bacteria within the gastrointestinal environment, enabling beneficial effects to be induced more effectively.Citation125 This provides a very practical method of overcoming the difficulties that a probiotic may endure when initially introduced into the gut environment. Challenges in designing a synbiotic include selecting the right combination of pro- and prebiotic and ensuring sufficient quantities of each. As bifidobacteria and lactobacilli are well-characterized members of the human intestinal consortium and the benefits of specific strains with respect to human health have been established, microbes from these genera are most frequently included in synbiotics. FOS, GOS, inulin and lactulose are common choices for synbiotic formulations. Some newly identified health-promoting bacteria have been the focus of investigations to assess the consequence of combining their use with some well-established prebiotics. For example, a synbiotic administration of A. caccae L2 with GOS to rats enhanced intestinal organic acid production more effectively than just GOS alone.Citation105 It was hypothesized that GOS increased levels of bifidobacteria, resulting in greater lactate production, which in turn promoted A. caccae growth, therefore, facilitating the production of butyrate by A. caccae. This demonstrates one way of utilizing the complex cross-feeding mechanisms to selectively manipulate target microbes.
Conclusion
The human gut harbors a diverse collection of microbes whose interactions and functionality have yet to be completely elucidated. However, it is already clear that these microbes play an integral role in human health and well-being. An increasing awareness that various microbes that can influence human health has been a catalyst for an ever-rising number of investigations into benefits of gut microbiota manipulation. Prebiotics, and other nutrients, targeted to specific health-promoting bacteria are establishing themselves as a significant means of improving host health and disease. This has been evident in the considerable body of work that has accumulated in the last few years by demonstrating that species composition within the microbiota can be modified with very few changes in food consumption. The expansion of the prebiotic definition to substrates that go beyond the classical prebiotics and the inclusion of bacteria other than Bifidobacterium and Lactobacillus have enabled a greater examination of this intriguing relationship between diet and the microbiota. It is anticipated that these substances can soon be harnessed to promote populations of newly-identified health promoting bacteria in the gut.
Additional information
Funding
References
- Sender R, Fuchs S, Milo R. Revised estimates for the number of human and bacteria cells in the body. PLoS Biol. 2016 Aug;14(8):e1002533. PubMed PMID: 27541692; PubMed Central PMCID: PMC4991899. doi:10.1371/journal.pbio.1002533.
- Qin J, Li R, Raes J, Arumugam M, Burgdorf KS, Manichanh C, Nielsen T, Pons N, Levenez F, Yamada T, et al. A human gut microbial gene catalogue established by metagenomic sequencing. Nature PubMed PMID: 20203603; PubMed Central PMCID: PMC3779803. 2010 Mar 4;464(7285):59–65. doi:10.1038/nature08821.
- Guilloteau P, Martin L, Eeckhaut V, Ducatelle R, Zabielski R, Van Immerseel F. From the gut to the peripheral tissues: the multiple effects of butyrate. Nutr Res Rev. 2010 Dec;23(2):366–384. PubMed PMID: WOS:000284716700012. doi:10.1017/s0954422410000247.
- Sekirov I, Russell SL, Antunes LC, Finlay BB. Gut microbiota in health and disease. Physiol Rev. 2010 Jul;90(3):859–904. PubMed PMID: 20664075. doi:10.1152/physrev.00045.2009.
- Blumberg R, Powrie F. Microbiota, disease, and back to health: a metastable journey. Sci Transl Med PubMed PMID: 22674557; PubMed Central PMCID: PMC5020897. 2012 Jun 6;4(137):137rv7. doi:10.1126/scitranslmed.3004184.
- Gibson GR, Roberfroid MB. Dietary modulation of the human colonic microbiota: introducing the concept of prebiotics. J Nutr. 1995 Jun;125(6):1401–1412. doi:10.1093/jn/125.6.1401. PubMed PMID: 7782892.
- Kaplan H, Rw H. Fermentation of fructooligosaccharides by lactic acid bacteria and bifidobacteria. Appl Environ Microbiol. 2000 Jun;66(6):2682–2684. PubMed PMID: 10831458; PubMed Central PMCID: PMC110601.
- Macfarlane GT, Steed H, Macfarlane S. Bacterial metabolism and health-related effects of galacto-oligosaccharides and other prebiotics. J Appl Microbiol. 2008 Feb;104(2):305–344. PubMed PMID: 18215222. doi:10.1111/j.1365-2672.2007.03520.x.
- Macfarlane S, Macfarlane GT, Cummings JH. Review article: prebiotics in the gastrointestinal tract. Aliment Pharm Therap PubMed PMID: WOS:000239799800001; English. 2006 Sep 1;24(5):701–714. doi:10.1111/j.1365-2036.2006.03042.x.
- Davis LM, Martinez I, Walter J, Hutkins R. A dose dependent impact of prebiotic galactooligosaccharides on the intestinal microbiota of healthy adults. Int J Food Microbiol PubMed PMID: 21059476. 2010 Dec 15;144(2):285–292. doi:10.1016/j.ijfoodmicro.2010.10.007.
- Goodrich JK, Waters JL, Poole AC, Sutter JL, Koren O, Blekhman R, Beaumont M, Van Treuren W, Knight R, Bell JT, et al. Human genetics shape the gut microbiome. Cell PubMed PMID: 25417156; PubMed Central PMCID: PMC4255478. 2014 Nov 6;159(4):789–799. doi:10.1016/j.cell.2014.09.053.
- Lopez-Siles M, Khan TM, Duncan SH, Harmsen HJM, Garcia-Gil LJ, Flint HJ. Cultured representatives of two major phylogroups of human colonic Faecalibacterium prausnitzii can utilize pectin, uronic acids, and host-derived substrates for growth. Appl Environ Microbiol. 2012 Jan;78(2):420–428. PubMed PMID: 22101049; PubMed Central PMCID: PMC3255724. doi:10.1128/AEM.06858-11.
- Belzer C, Lw C, Aalvink S, Chamlagain B, Piironen V, Knol J, de Vos WM, Dubilier N. Microbial metabolic networks at the mucus layer lead to diet-independent butyrate and vitamin B12 production by intestinal symbionts. mBio. 2017 Sep 19;8(5). PubMed PMID: 28928206; PubMed Central PMCID: PMC5605934. doi:10.1128/mBio.00770-17.
- Duncan SH, Louis P, Flint HJ. Lactate-utilizing bacteria, isolated from human feces, that produce butyrate as a major fermentation product. Appl Environ Microbiol. 2004 Oct;70(10):5810–5817. PubMed PMID: 15466518; PubMed Central PMCID: PMC522113. doi:10.1128/AEM.70.10.5810-5817.2004.
- Riviere A, Gagnon M, Weckx S, Roy D, De Vuyst L. Mutual cross-feeding interactions between bifidobacterium longum subsp longum NCC2705 and eubacterium rectale ATCC 33656 explain the bifidogenic and butyrogenic effects of arabinoxylan oligosaccharides. Appl Environ Microbiol. 2015 Nov;81(22):7767–7781. PubMed PMID: WOS:000363463800010; English. doi:10.1128/Aem.02089-15.
- Louis P, Young P, Holtrop G, Flint HJ. Diversity of human colonic butyrate-producing bacteria revealed by analysis of the butyryl-CoA: acetateCoA-transferase gene. Environ Microbiol. 2010 Feb;12(2):304–314. PubMed PMID: WOS:000274234600003; English. doi:10.1111/j.1462-2920.2009.02066.x.
- Louis P, Flint HJ. Diversity, metabolism and microbial ecology of butyrate-producing bacteria from the human large intestine. FEMS Microbiol Lett. 2009 May;294(1):1–8. PubMed PMID: WOS:000264882000001; English. doi:10.1111/j.1574-6968.2009.01514.x.
- Allen-Vercoe E, Daigneault M, White A, Panaccione R, Duncan SH, Flint HJ, O’Neal L, Lawson PA. Anaerostipes hadrus comb. nov., a dominant species within the human colonic microbiota; reclassification of Eubacterium hadrum Moore et al. 1976. Anaerobe. 2012 Oct;18(5):523–529. PubMed PMID: WOS:000310943200008; English. doi:10.1016/j.anaerobe.2012.09.002.
- Machiels K, Joossens M, Sabino J, De Preter V, Arijs I, Eeckhaut V, Ballet V, Claes K, Van Immerseel F, Verbeke K, et al. A decrease of the butyrate-producing species Roseburia hominis and Faecalibacterium prausnitzii defines dysbiosis in patients with ulcerative colitis. Gut. 2014 Aug;63(8):1275–1283. PubMed PMID: 24021287. doi:10.1136/gutjnl-2013-304833.
- Reichardt N, Duncan SH, Young P, Belenguer A, McWilliam Leitch C, Scott KP, Flint HJ, Louis P. Phylogenetic distribution of three pathways for propionate production within the human gut microbiota. ISME J. 2014 Jun;8(6):1323–1335. PubMed PMID: 24553467; PubMed Central PMCID: PMC4030238. doi:10.1038/ismej.2014.14.
- Duncan SH, Hold GL, Barcenilla A, Stewart CS, Flint HJ. Roseburia intestinalis sp nov., a novel saccharolytic, butyrate-producing bacterium from human faeces. Int J Syst Evol Micr. 2002 Sep;52. 1615–1620. PubMed PMID: WOS:000178117500021; English. doi: 10.1099/ijs.0.02143-0.
- Heinken A, Khan MT, Paglia G, Rodionov DA, Harmsen HJM, Thiele I. Functional metabolic map of Faecalibacterium prausnitzii, a beneficial human gut microbe. J Bacteriol. 2014 Sep;196(18):3289–3302. PubMed PMID: 25002542; PubMed Central PMCID: PMC4135701. doi:10.1128/JB.01780-14.
- Miquel S, Martin R, Rossi O, Bermúdez-Humarán LG, Chatel JM, Sokol H, Thomas M, Wells JM, Langella P. Faecalibacterium prausnitzii and human intestinal health. Curr Opin Microbiol. 2013 Jun;16(3):255–261. PubMed PMID: 23831042. doi:10.1016/j.mib.2013.06.003.
- Konikoff T, Gophna U. Oscillospira: a central, enigmatic component of the human gut microbiota. Trends Microbiol. 2016 Jul;24(7):523–524. PubMed PMID: 26996766. doi:10.1016/j.tim.2016.02.015.
- Ze XL, Duncan SH, Louis P, Flint HJ. Ruminococcus bromii is a keystone species for the degradation of resistant starch in the human colon. ISME J. 2012 Aug;6(8):1535–1543. PubMed PMID: WOS:000306495800009; English. doi:10.1038/ismej.2012.4.
- Venkataraman A, Sieber JR, Schmidt AW, Waldron C, Theis KR, Schmidt TM. Variable responses of human microbiomes to dietary supplementation with resistant starch. Microbiome. 2016 Jun 29;4(1):33. doi:10.1186/s40168-016-0178-x. 10.1186/s40168-016-0178-x. PubMed PMID: 27357127; PubMed Central PMCID: PMC4928258.
- Cani PD, de Vos WM. Next-generation beneficial microbes: the case of akkermansia muciniphila. Front Microbiol PubMed PMID: 29018410; PubMed Central PMCID: PMC5614963. 2017;8:1765. doi:10.3389/fmicb.2017.01765.
- Everard A, Belzer C, Geurts L, Ouwerkerk JP, Druart C, Bindels LB, Guiot Y, Derrien M, Muccioli GG, Delzenne NM, et al. Cross-talk between Akkermansia muciniphila and intestinal epithelium controls diet-induced obesity. Proc Natl Acad Sci U S A PubMed PMID: WOS:000320500000075; English. 2013 May 28;110(22):9066–9071. doi:10.1073/pnas.1219451110.
- Schneeberger M, Everard A, Gomez-Valades AG, Matamoros S, Ramírez S, Delzenne NM, Gomis R, Claret M, Cani PD. Akkermansia muciniphila inversely correlates with the onset of inflammation, altered adipose tissue metabolism and metabolic disorders during obesity in mice. Sci Rep. 2015 Nov 13;5:16643. PubMed PMID: 26563823; PubMed Central PMCID: PMC4643218. doi:10.1038/srep16643.
- Gl H, Schwiertz A, Ri A, Blaut M, Flint HJ. Oligonucleotide probes that detect quantitatively significant groups of butyrate-producing bacteria in human feces. Appl Environ Microbiol. 2003 Jul;69(7):4320–4324. PubMed PMID: 12839823; PubMed Central PMCID: PMC165216.
- Fujio-Vejar S, Vasquez Y, Morales P, Magne F, Vera-Wolf P, Ugalde JA, Navarrete P, Gotteland M. The gut microbiota of healthy chilean subjects reveals a high abundance of the phylum verrucomicrobia. Front Microbiol PubMed PMID: 28713349; PubMed Central PMCID: PMC5491548. 2017;8:1221. doi:10.3389/fmicb.2017.01221.
- Sokol H, Pigneur B, Watterlot L, Lakhdari O, Bermúdez-Humarán LG, Gratadoux -J-J, Blugeon S, Bridonneau C, Furet J-P, Corthier G, et al. Faecalibacterium prausnitzii is an anti-inflammatory commensal bacterium identified by gut microbiota analysis of Crohn disease patients. Proc Natl Acad Sci U S A. 2008 Oct 28;105(43):16731–16736. PubMed PMID: 18936492; PubMed Central PMCID: PMC2575488. doi:10.1073/pnas.0804812105.
- Derrien M, Belzer C, de Vos WM. Akkermansia muciniphila and its role in regulating host functions. Microb Pathog. 2017 May;106:171–181. PubMed PMID: 26875998. doi:10.1016/j.micpath.2016.02.005.
- Derrien M, Collado MC, Ben-Amor K, Salminen S, de Vos WM. The mucin degrader Akkermansia muciniphila is an abundant resident of the human intestinal tract. Appl Environ Microbiol. 2008 Mar;74(5):1646–1648. PubMed PMID: WOS:000253792700041; English. doi:10.1128/Aem.01226-07.
- Derrien M, Vaughan EE, Plugge CM, de Vos WM. Akkermansia muciniphila gen. nov., sp nov., a human intestinal mucin-degrading bacterium. Int J Syst Evol Micr. 2004 Sep;54:1469–1476. PubMed PMID: WOS:000224259100007; English. doi: 10.1099/ijs.0.2873-0.
- Tramontano M, Andrejev S, Pruteanu M, Klunemann M, Kuhn M, Galardini M, Jouhten P, Zelezniak A, Zeller G, Bork P, et al. Nutritional preferences of human gut bacteria reveal their metabolic idiosyncrasies. Nat Microbiol. 2018 Mar 19. PubMed PMID: 29556107. doi: 10.1038/s41564-018-0123-9.
- Ma N, Guo P, Zhang J, He T, Kim SW, Zhang G, Ma X. Nutrients mediate intestinal bacteria-mucosal immune crosstalk. Front Immunol. 2018;9:5. PubMed PMID: 29416535; PubMed Central PMCID: PMC5787545. doi:10.3389/fimmu.2018.00005.
- Leonel AJ, Alvarez-Leite JI. Butyrate: implications for intestinal function. Curr Opin Clin Nutr. 2012 Sep;15(5):474–479. PubMed PMID: WOS:000307826500012; English. doi:10.1097/MCO.0b013e32835665fa.
- Wong JMW, de Souza R, Kendall CWC, Emam A, Jenkins DJA. Colonic health: fermentation and short chain fatty acids. J Clin Gastroenterol. 2006 Mar;40(3):235–243. PubMed PMID: WOS:000236816600015; English. doi:10.1097/00004836-200603000-00015.
- Canani RB, Di Costanzo M, Leone L, Pedata M, Meli R, Calignano A. Potential beneficial effects of butyrate in intestinal and extrainitestinal diseases. World J Gastroentero. 2011 Mar 28;17(12):1519–1528. PubMed PMID: WOS:000289611300001; English. doi:10.3748/wjg.v17.i12.1519.
- Liu H, Wang J, He T, Becker S, Zhang G, Li D, Ma X. Butyrate: a double-edged sword for health? Adv Nutr. 2018 Jan 1;9(1):21–29. PubMed PMID: 29438462; PubMed Central PMCID: PMC6333934. doi:10.1093/advances/nmx009.
- Huang C, Song P, Fan P, Hou C, Thacker P, Ma X. Dietary sodium butyrate decreases postweaning diarrhea by modulating intestinal permeability and changing the bacterial communities in weaned piglets. J Nutr. 2015 Dec;145(12):2774–2780. PubMed PMID: 26491121. doi:10.3945/jn.115.217406.
- Louis P, Hold GL, Flint HJ. The gut microbiota, bacterial metabolites and colorectal cancer. Nat Rev Microbiol. 2014 Oct;12(10):661–672. PubMed PMID: WOS:000342267900008; English. doi:10.1038/nrmicro3344.
- Rios-Covian D, Ruas-Madiedo P, Margolles A, Gueimonde M, de Los Reyes-Gavilán CG, Salazar N. Intestinal short chain fatty acids and their link with diet and human health. Front Microbiol. 2016 Feb 17;7:Artn 185. PubMed PMID: WOS:000370222600001; English. doi:10.3389/Fmicb.2016.00185.
- Munoz-Tamayo R, Laroche B, Walter E, Doré J, Duncan SH, Flint HJ, Leclerc M. Kinetic modelling of lactate utilization and butyrate production by key human colonic bacterial species. FEMS Microbiol Ecol. 2011 Jun;76(3):615–624. PubMed PMID: WOS:000290314900018; English. doi:10.1111/j.1574-6941.2011.01085.x.
- Gibson GR, Hutkins R, Sanders ME, Prescott SL, Reimer RA, Salminen SJ, Scott K, Stanton C, Swanson KS, Cani PD, et al. Expert consensus document: the International Scientific Association for Probiotics and Prebiotics (ISAPP) consensus statement on the definition and scope of prebiotics. Nat. Rev. Gastroenterol. Hepatol. 2017 Aug;14(8):491–502. PubMed PMID: 28611480. doi:10.1038/nrgastro.2017.75.
- Gibson GR, Probert HM, Loo JV, Rastall RA, Roberfroid MB. Dietary modulation of the human colonic microbiota: updating the concept of prebiotics. Nutr Res Rev. 2004 Dec;17(2):259–275. PubMed PMID: 19079930. doi:10.1079/NRR200479.
- Bindels LB, Delzenne NM, Cani PD, Walter J. Towards a more comprehensive concept for prebiotics. Nat Rev Gastroenterol Hepatol. 2015 May;12(5):303–310. PubMed PMID: 25824997. doi:10.1038/nrgastro.2015.47.
- Sun Y, O‘Riordan MX. Regulation of bacterial pathogenesis by intestinal short-chain Fatty acids. Adv Appl Microbiol. 2013;85:93–118. PubMed PMID: 23942149; PubMed Central PMCID: PMC4029053. doi:10.1016/B978-0-12-407672-3.00003-4.
- Cummings JH, Macfarlane GT. Gastrointestinal effects of prebiotics. Brit J Nutr. 2002 May;87:S145–S151. PubMed PMID: WOS:000176469700002; English. doi:10.1079/Bn/2002530.
- Marteau P, Seksik P. Tolerance of probiotics and prebiotics. J Clin Gastroenterol. 2004 Jul;38(6):S67–S69. PubMed PMID: WOS:000222317000005; English. doi:10.1097/01.mcg.0000128929.37156.a7.
- Cummings JH, Macfarlane GT, Englyst HN. Prebiotic digestion and fermentation. Am J Clin Nutr. 2001 Feb;73(2):415s–420s. PubMed PMID: WOS:000166608200010; English. doi:10.1093/ajcn/73.2.415s.
- Dewulf EM, Cani PD, Claus SP, Fuentes S, Puylaert PGB, Neyrinck AM, Bindels LB, de Vos WM, Gibson GR, Thissen J-P, et al. Insight into the prebiotic concept: lessons from an exploratory, double blind intervention study with inulin-type fructans in obese women. Gut. 2013 Aug;62(8):1112–1121. PubMed PMID: 23135760; PubMed Central PMCID: PMC3711491. doi:10.1136/gutjnl-2012-303304.
- Liu F, Li P, Chen M, Luo Y, Prabhakar M, Zheng H, He Y, Qi Q, Long H, Zhang Y, et al. Fructooligosaccharide (FOS) and Galactooligosaccharide (GOS) increase bifidobacterium but reduce butyrate producing bacteria with adverse glycemic metabolism in healthy young population. Sci Rep. 2017 Sep 18;7(1):11789. 10.1038/s41598-017-10722-2. PubMed PMID: 28924143; PubMed Central PMCID: PMC5603605. doi:10.1038/s41598-017-10722-2.
- Azcarate-Peril MA, Ritter AJ, Savaiano D, Monteagudo-Mera A, Anderson C, Magness ST, Klaenhammer TR. Impact of short-chain galactooligosaccharides on the gut microbiome of lactose-intolerant individuals. Proc Natl Acad Sci U S A. 2017 Jan 17;114(3):E367–E375. PubMed PMID: 28049818; PubMed Central PMCID: PMC5255593. doi:10.1073/pnas.1606722113.
- Ramirez-Farias C, Slezak K, Fuller Z, Duncan A, Holtrop G, Louis P. Effect of inulin on the human gut microbiota: stimulation of Bifidobacterium adolescentis and Faecalibacterium prausnitzii. Br J Nutr. 2009 Feb;101(4):541–550. PubMed PMID: 18590586. doi:10.1017/S0007114508019880.
- Vandeputte D, Falony G, Vieira-Silva S, Wang J, Sailer M, Theis S, Verbeke K, Raes J. Prebiotic inulin-type fructans induce specific changes in the human gut microbiota. Gut. 2017 Nov;66(11):1968–1974. PubMed PMID: 28213610; PubMed Central PMCID: PMC5739857. doi:10.1136/gutjnl-2016-313271.
- Moreno-Indias I, Sanchez-Alcoholado L, Perez-Martinez P, Andrés-Lacueva C, Cardona F, Tinahones F, Queipo-Ortuño MI. Red wine polyphenols modulate fecal microbiota and reduce markers of the metabolic syndrome in obese patients. Food Funct. 2016 Apr;7(4):1775–1787. PubMed PMID: 26599039. doi:10.1039/c5fo00886g.
- Queipo-Ortuno MI, Boto-Ordonez M, Murri M, Gomez-Zumaquero JM, Clemente-Postigo M, Estruch R, Cardona Diaz F, Andrés-Lacueva C, Tinahones FJ. Influence of red wine polyphenols and ethanol on the gut microbiota ecology and biochemical biomarkers. Am J Clin Nutr. 2012 Jun;95(6):1323–1334. PubMed PMID: 22552027. doi:10.3945/ajcn.111.027847.
- Tzounis X, Rodriguez-Mateos A, Vulevic J, Gibson GR, Kwik-Uribe C, Spencer JPE. Prebiotic evaluation of cocoa-derived flavanols in healthy humans by using a randomized, controlled, double-blind, crossover intervention study. Am J Clin Nutr. 2011 Jan;93(1):62–72. PubMed PMID: WOS:000285453500010; English. doi:10.3945/ajcn.110.000075.
- Finegold SM, Li ZP, Summanen PH, Downes J, Thames G, Corbett K, Dowd S, Krak M, Heber D. Xylooligosaccharide increases bifidobacteria but not lactobacilli in human gut microbiota. Food Funct. 2014 Mar;5(3):436–445. PubMed PMID: WOS:000333226000003; English. doi:10.1039/c3fo60348b.
- Maier TV, Lucio M, Lee LH, VerBerkmoes NC, Brislawn CJ, Bernhardt J, Lamendella R, McDermott JE, Bergeron N, Heinzmann SS, et al. Impact of dietary resistant starch on the human gut microbiome, metaproteome, and metabolome. mBio. 2017 Oct 17;8(5). PubMed PMID: 29042495; PubMed Central PMCID: PMC5646248. doi:10.1128/mBio.01343-17.
- Tsao R. Chemistry and biochemistry of dietary polyphenols. Nutrients. 2010 Dec;2(12):1231–1246. PubMed PMID: WOS:000298239500003; English. doi:10.3390/nu2121231.
- Lunet N, Lacerda-Vieira A, Barros H. Fruit and vegetables consumption and gastric cancer: a systematic review and meta-analysis of cohort studies. Nutr Cancer. 2005;53(1):1–10. PubMed PMID: 16351501. doi:10.1207/s15327914nc5301_1.
- Chong MF, Macdonald R, Lovegrove JA. Fruit polyphenols and CVD risk: a review of human intervention studies. Br J Nutr. 2010 Oct;104(Suppl 3):S28–39. doi:10.1017/S0007114510003922. PubMed PMID: 20955648.
- Liu YJ, Zhan J, Liu XL, Wang Y, Ji J, He -Q-Q. Dietary flavonoids intake and risk of type 2 diabetes: a meta-analysis of prospective cohort studies. Clin Nutr. 2014 Feb;33(1):59–63. PubMed PMID: 23591151. doi:10.1016/j.clnu.2013.03.011.
- Zamora-Ros R, Forouhi NG, Sharp SJ, González CA, Buijsse B, Guevara M, van der Schouw YT, Amiano P, Boeing H, Bredsdorff L, et al. Dietary intakes of individual flavanols and flavonols are inversely associated with incident type 2 diabetes in European populations. J Nutr. 2014 Mar;144(3):335–343. PubMed PMID: 24368432; PubMed Central PMCID: PMC3927546. doi:10.3945/jn.113.184945.
- Park OJ, Surh YJ. Chemopreventive potential of epigallocatechin gallate and genistein: evidence from epidemiological and laboratory studies. Toxicol Lett. 2004 Apr 15;150(1):43–56. PubMed PMID: 15068824. doi:10.1016/j.toxlet.2003.06.001.
- Cardona F, Andres-Lacueva C, Tulipani S, Tinahones FJ, Queipo-Ortuno MI. Benefits of polyphenols on gut microbiota and implications in human health. J Nutr Biochem. 2013 Aug;24(8):1415–1422. PubMed PMID: 23849454. doi:10.1016/j.jnutbio.2013.05.001.
- Anhe FF, Roy D, Pilon G, Dudonné S, Matamoros S, Varin TV, Garofalo C, Moine Q, Desjardins Y, Levy E, et al. A polyphenol-rich cranberry extract protects from diet-induced obesity, insulin resistance and intestinal inflammation in association with increased Akkermansia spp. population in the gut microbiota of mice. Gut. 2015 Jun;64(6):872–883. PubMed PMID: 25080446. doi:10.1136/gutjnl-2014-307142.
- Pierre JF, Heneghan AF, Feliciano RP, Shanmuganayagam D, Roenneburg DA, Krueger CG, Reed JD, Kudsk KA. Cranberry proanthocyanidins improve the gut mucous layer morphology and function in mice receiving elemental enteral nutrition. JPEN J Parenteral Enteral Nutr. 2013 May-Jun;37(3):401–409. PubMed PMID: 23064255; PubMed Central PMCID: PMC4564871. doi:10.1177/0148607112463076.
- Baldwin J, Collins B, Wolf PG, Martinez K, Shen W, Chuang -C-C, Zhong W, Cooney P, Cockrell C, Chang E, et al. Table grape consumption reduces adiposity and markers of hepatic lipogenesis and alters gut microbiota in butter fat-fed mice. J Nutr Biochem. 2016 Jan;27:123–135. PubMed PMID: 26423887; PubMed Central PMCID: PMC4933288. doi:10.1016/j.jnutbio.2015.08.027.
- Roopchand DE, Carmody RN, Kuhn P, Moskal K, Rojas-Silva P, Turnbaugh PJ, Raskin I. Dietary polyphenols promote growth of the gut bacterium akkermansia muciniphila and attenuate high-fat diet-induced metabolic syndrome. Diabetes. 2015 Aug;64(8):2847–2858. PubMed PMID: 25845659; PubMed Central PMCID: PMC4512228. doi:10.2337/db14-1916.
- Tzounis X, Vulevic J, Kuhnle GG, George T, Leonczak J, Gibson GR, Kwik-Uribe C, Spencer JPE. Flavanol monomer-induced changes to the human faecal microflora. Br J Nutr. 2008 Apr;99(4):782–792. PubMed PMID: 17977475. doi:10.1017/S0007114507853384.
- Samanta AK, Jayapal N, Jayaram C, Roy S, Kolte AP, Senani S, Sridhar M. Xylooligosaccharides as prebiotics from agricultural by-products: production and applications. Bioact Carbohydr Dietary Fibre. 2015 Jan 01;5(1):62–71. doi:10.1016/j.bcdf.2014.12.003.
- Scott KP, Martin JC, Duncan SH, Flint HJ. Prebiotic stimulation of human colonic butyrate-producing bacteria and bifidobacteria, in vitro. FEMS Microbiol Ecol. 2014 Jan;87(1):30–40. PubMed PMID: 23909466. doi:10.1111/1574-6941.12186.
- Lecerf JM, Depeint F, Clerc E, Dugenet Y, Niamba CN, Rhazi L, Cayzeele A, Abdelnour G, Jaruga A, Younes H, et al. Xylo-oligosaccharide (XOS) in combination with inulin modulates both the intestinal environment and immune status in healthy subjects, while XOS alone only shows prebiotic properties. Br J Nutr. 2012 Nov 28;108(10):1847–1858. PubMed PMID: 22264499. doi:10.1017/S0007114511007252.
- Flint HJ, Scott KP, Duncan SH, Louis P, Forano E. Microbial degradation of complex carbohydrates in the gut. Gut Microbes. 2012 Jul-Aug;3(4):289–306. PubMed PMID: 22572875; PubMed Central PMCID: PMC3463488. doi:10.4161/gmic.19897.
- Gullon B, P G, Tavaria F, Pintado M, Gomes AM, Alonso JL, Parajó JC. Structural features and assessment of prebiotic activity of refined arabinoxylooligosaccharides from wheat bran. J Funct Foods. 2014 Jan;6:438–449. PubMed PMID: WOS:000331423000044; English. doi:10.1016/j.jff.2013.11.010.
- Neyrinck AM, Possemiers S, Druart C, Van de Wiele T, De Backer F, Cani PD, Larondelle Y, Delzenne NM, Brennan L. Prebiotic effects of wheat arabinoxylan related to the increase in bifidobacteria, roseburia and bacteroides/prevotella in diet-induced obese mice. PLoS One. 2011 Jun 9;6(6):ARTN e20944. PubMed PMID: WOS:000291612900042; English. doi:10.1371/journal.pone.0020944.
- Van Craeyveld V, Swennen K, Dornez E, Van de Wiele T, Marzorati M, Verstraete W, Delaedt Y, Onagbesan O, Decuypere E, Buyse J, et al. Structurally different wheat-derived arabinoxylooligosaccharides have different prebiotic and fermentation properties in rats. J Nutr. 2008 Dec;138(12):2348–2355. PubMed PMID: WOS:000261038300010; English. doi:10.3945/jn.108.094367.
- Chung WSF, Meijerink M, Zeuner B, Holck J, Louis P, Meyer AS, Wells JM, Flint HJ, Duncan SH. Prebiotic potential of pectin and pectic oligosaccharides to promote anti-inflammatory commensal bacteria in the human colon. FEMS Microbiol Ecol. 2017 Nov;93(11):ARTN fix127. PubMed PMID: WOS:000416389100005; English. doi:10.1093/femsec/fix127.
- Gullon B, B G, Martinez-Sabajanes M, Yáñez R, Parajó JC, Alonso JL. Pectic oligosaccharides: manufacture and functional properties. Trends Food Sci Tech. 2013 Apr;30(2):153–161. PubMed PMID: WOS:000318391900006; English. doi:10.1016/j.tifs.2013.01.006.
- Koulsos A, Lima M, Conterno L, Gasperotti M, Bianchi M, Fava F, Vrhovsek U, Lovegrove J, Tuohy K. Effects of commercial apple varieties on human gut microbiota composition and metabolic output using an in vitro colonic model. Nutrients. 2017 Jun;9(6):Artn 533. PubMed PMID: WOS:000404177100004; English. doi:10.3390/Nu9060533.
- Gomez B, Gullon B, Yanez R, Schols H, Alonso JL. Prebiotic potential of pectins and pectic oligosaccharides derived from lemon peel wastes and sugar beet pulp: A comparative evaluation. J Funct Foods. 2016 Jan;20:108–121. doi:10.1016/j.jff.2015.10.029. PubMed PMID: WOS:000375634900011.
- Goffin D, Delzenne N, Blecker C, Hanon E, Deroanne C, Paquot M. Will isomalto-oligosaccharides, a well-established functional food in Asia, break through the European and American market? The status of knowledge on these prebiotics. Crit Rev Food Sci Nutr. 2011 May;51(5):394–409. PubMed PMID: 21491266. doi:10.1080/10408391003628955.
- Singh DP, Singh S, Bijalwan V, Kumar, V. Khare P, Baboota RK, Singh P, Boparai RK, Singh J, Kondepudi KK, et al. Co-supplementation of isomalto-oligosaccharides potentiates metabolic health benefits of polyphenol-rich cranberry extract in high fat diet-fed mice via enhanced gut butyrate production. Eur J Nutr. 2017 Nov 10. PubMed PMID: 29127476. doi:10.1007/s00394-017-1561-5.
- Re W, Ninonuevo M, Da M, Lebrilla CB, German JB. In vitro fermentability of human milk oligosaccharides by several strains of bifidobacteria. Mol Nutr Food Res. 2007 Nov;51(11):1398–1405. PubMed PMID: 17966141. doi:10.1002/mnfr.200700150.
- Elison E, Vigsnaes LK, Rindom Krogsgaard L, Rasmussen J, Sørensen N, McConnell B, Hennet T, Sommer MOA, Bytzer P. Oral supplementation of healthy adults with 2‘-O-fucosyllactose and lacto-N-neotetraose is well tolerated and shifts the intestinal microbiota. Br J Nutr. 2016 Oct;116(8):1356–1368. PubMed PMID: 27719686; PubMed Central PMCID: PMC5082288. doi:10.1017/S0007114516003354.
- Marcobal A, Barboza M, Jw F, Block DE, German JB, Lebrilla CB, Mills DA. Consumption of human milk oligosaccharides by gut-related microbes. J Agr Food Chem. 2010 May 12;58(9):5334–5340. PubMed PMID: WOS:000277237500018; English. doi:10.1021/jf9044205.
- Rycroft CE, Jones MR, Gibson GR, Rastall RA. A comparative in vitro evaluation of the fermentation properties of prebiotic oligosaccharides. J Appl Microbiol. 2001 Nov;91(5):878–887. PubMed PMID: 11722666.
- Zheng J, Li H, Zhang X, Jiang M, Luo C, Lu Z, Xu Z, Shi J. Prebiotic mannan-oligosaccharides augment the hypoglycemic effects of metformin in correlation with modulating gut microbiota. J Agric Food Chem. 2018 Jun 13;66(23):5821–5831. PubMed PMID: 29701959. doi:10.1021/acs.jafc.8b00829.
- Ehara T, Izumi H, Tsuda M, Nakazato Y, Iwamoto H, Namba K, Takeda Y. Combinational effects of prebiotic oligosaccharides on bifidobacterial growth and host gene expression in a simplified mixed culture model and neonatal mice. Br J Nutr. 2016 Jul;116(2):270–278. PubMed PMID: 27198516. doi:10.1017/S0007114516001987.
- Abell GC, Cooke CM, Bennett CN, Conlon MA, McOrist AL. Phylotypes related to Ruminococcus bromii are abundant in the large bowel of humans and increase in response to a diet high in resistant starch. FEMS Microbiol Ecol. 2008 Dec;66(3):505–515. PubMed PMID: 18616586. doi:10.1111/j.1574-6941.2008.00527.x.
- Martinez I, Kim J, Duffy PR, Schlegel VL, Walter J, Heimesaat MM. Resistant starches types 2 and 4 have differential effects on the composition of the fecal microbiota in human subjects. PLoS One. 2010 Nov 29;5(11):e15046. PubMed PMID: 21151493; PubMed Central PMCID: PMC2993935. doi:10.1371/journal.pone.0015046.
- Walker AW, Ince J, Duncan SH, Webster LM, Holtrop G, Ze X, Brown D, Stares MD, Scott P, Bergerat A, et al. Dominant and diet-responsive groups of bacteria within the human colonic microbiota. ISME J. 2011 Feb;5(2):220–230. PubMed PMID: 20686513; PubMed Central PMCID: PMC3105703. doi:10.1038/ismej.2010.118.
- Baxter NT, Schmidt AW, Venkataraman A, Cheng M, Zhang B, Zhang H, Gerstenecker GS, Pakrasi HB, Gross ML, Blankenship RE. Dynamics of human gut microbiota and short-chain fatty acids in response to dietary interventions with three fermentable fibers. mBio. 2019 Jan 29;10(1). PubMed PMID: 30696735; PubMed Central PMCID: PMC6355990. doi:10.1128/mBio.02566-18.
- Zaman SA, Sarbini SR. The potential of resistant starch as a prebiotic. Crit Rev Biotechnol. 2016;36(3):578–584. PubMed PMID: 25582732. doi:10.3109/07388551.2014.993590.
- O‘Sullivan L, Murphy B, McLoughlin P, Duggan P, Lawlor PG, Hughes H, Gardiner GE. Prebiotics from marine macroalgae for human and animal health applications. Mar Drugs. 2010 Jul 8;7. 2038–2064. PubMed PMID: WOS:000280347100006; English. doi: 10.3390/md8072038.
- Okolie CL, Rajendran SRCK, Udenigwe CC, Aryee ANA, Mason B. Prospects of brown seaweed polysaccharides (BSP) as prebiotics and potential immunomodulators. J Food Biochem. 2017 Oct;41(5):ARTN e12392. PubMed PMID: WOS:000412117000006; English. doi:10.1111/jfbc.12392.
- Zvyagintseva TN, Shevchenko NM, Popivnich IB, Isakov VV, Scobun AS, Sundukova EV, Elyakova LA. A new procedure for the separation of water-soluble polysaccharides from brown seaweeds. Carbohyd Res. 1999 Nov 23;322(1–2):32–39. doi:10.1016/S0008-6215(99)00206-2.
- Shang Q, Shan X, Cai C, Hao J, Li G, Yu G. Dietary fucoidan modulates the gut microbiota in mice by increasing the abundance of Lactobacillus and Ruminococcaceae. Food Funct. 2016 Jul 13;7(7):3224–3232. PubMed PMID: 27334000. doi:10.1039/c6fo00309e.
- Shang QS, Song GR, Zhang MF, Shi J, Xu C, Hao J, Li G, Yu G. Dietary fucoidan improves metabolic syndrome in association with increased Akkermansia population in the gut microbiota of high-fat diet-fed mice. J Funct Foods. 2017 Jan;28:138–146. PubMed PMID: WOS:000392885000017. doi:10.1016/j.jff.2016.11.002.
- Belenguer A, Duncan SH, Calder AG, Holtrop G, Louis P, Lobley GE, Flint HJ. Two routes of metabolic cross-feeding between Bifidobacterium adolescentis and butyrate-producing anaerobes from the human gut. Appl Environ Microbiol. 2006 May;72(5):3593–3599. PubMed PMID: 16672507; PubMed Central PMCID: PMC1472403. doi:10.1128/AEM.72.5.3593-3599.2006.
- Sato T, Matsumoto K, Okumura T, Yokoi W, Naito E, Yoshida Y, Nomoto K, Ito M, Sawada H. Isolation of lactate-utilizing butyrate-producing bacteria from human feces and in vivo administration of Anaerostipes caccae strain L2 and galacto-oligosaccharides in a rat model. FEMS Microbiol Ecol. 2008 Dec;66(3):528–536. PubMed PMID: WOS:000261060600006; English. doi:10.1111/j.1574-6941.2008.00528.x.
- Duncan SH, Barcenilla A, Stewart CS, Pryde SE, Flint HJ. Acetate utilization and butyryl coenzyme A (CoA): acetate-CoAtransferase in butyrate-producing bacteria from the human large intestine. Appl Environ Microbiol. 2002 Oct;68(10):5186–5190. PubMed PMID: 12324374; PubMed Central PMCID: PMC126392.
- Duncan SH, Hold GL, Harmsen HJ, Stewart CS, Flint HJ. Growth requirements and fermentation products of Fusobacterium prausnitzii, and a proposal to reclassify it as Faecalibacterium prausnitzii gen. nov., comb. nov. Int J Syst Evol Microbiol. 2002 Nov;52(Pt6)):2141–2146. PubMed PMID: 12508881. doi:10.1099/00207713-52-6-2141.
- Barcenilla A, Pryde SE, Martin JC, Duncan SH, Stewart CS, Henderson C, Flint HJ. Phylogenetic relationships of butyrate-producing bacteria from the human gut. Appl Environ Microbiol. 2000 Apr;66(4):1654–1661. PubMed PMID: 10742256; PubMed Central PMCID: PMC92037.
- Louis P, Duncan SH, McCrae SI, Millar J, Jackson MS, Flint HJ. Restricted distribution of the butyrate kinase pathway among butyrate-producing bacteria from the human colon. J Bacteriol. 2004 Apr;186(7):2099–2106. PubMed PMID: 15028695; PubMed Central PMCID: PMC374397.
- Collado MC, Derrien M, Isolauri E. Intestinal integrity and Akkermansia muciniphila, a mucin-degrading member of the intestinal microbiota present in infants, adults, and the elderly. Appl Environ Microbiol. 2007 Dec;73(23):7767–7770. PubMed PMID: WOS:000251474400038; English. doi:10.1128/Aem.01477-07.
- Ottman N, Davids M, Suarez-Diez M, Boeren S, Schaap PJ, Martins Dos Santos VAP, Smidt H, Belzer C, de Vos WM. Genome-scale model and omics analysis of metabolic capacities of akkermansia muciniphila reveal a preferential mucin-degrading lifestyle. Appl Environ Microbiol. 2017 Sep 15;83(18). PubMed PMID: 28687644; PubMed Central PMCID: PMC5583483. doi: 10.1128/AEM.01014-17.
- Magnusdottir S, Heinken A, Kutt L, Ravcheev DA, Bauer E, Noronha A, Greenhalgh K, Jäger C, Baginska J, Wilmes P, et al. Generation of genome-scale metabolic reconstructions for 773 members of the human gut microbiota. Nat Biotechnol. 2017 Jan;35(1):81–89. PubMed PMID: 27893703. doi:10.1038/nbt.3703.
- van der Ark KCH, Aalvink S, Suarez-Diez M, Schaap PJ, de Vos WM, Belzer C. Model-driven design of a minimal medium for Akkermansia muciniphila confirms mucus adaptation. Microb Biotechnol. 2018 May;11(3):476–485. PubMed PMID: 29377524; PubMed Central PMCID: PMC5902328. doi:10.1111/1751-7915.13033.
- Neyrinck AM, Bindels LB, De Backer F, Pachikian BD, Cani PD, Delzenne NM. Dietary supplementation with chitosan derived from mushrooms changes adipocytokine profile in diet-induced obese mice, a phenomenon linked to its lipid-lowering action. Int Immunopharmacol. 2009 Jun;9(6):767–773. PubMed PMID: WOS:000266568800019; English. doi:10.1016/j.intimp.2009.02.015.
- WHO. The global prevalence of anaemia in 2011. Geneva (Switzerland): World Health Organization; 2015.
- Jaeggi T, Kortman GAM, Moretti D, Chassard C, Holding P, Dostal A, Boekhorst J, Timmerman HM, Swinkels DW, Tjalsma H, et al. Iron fortification adversely affects the gut microbiome, increases pathogen abundance and induces intestinal inflammation in Kenyan infants. Gut. 2015 May;64(5):731–742. PubMed PMID: 25143342. doi:10.1136/gutjnl-2014-307720.
- Kortman GA, Dutilh BE, Maathuis AJ, Engelke UF, Boekhorst J, Keegan KP, Nielsen FG, Betley J, Weir JC, Kingsbury Z, et al. Microbial metabolism shifts towards an adverse profile with supplementary iron in the TIM-2 in vitro model of the human colon. Front Microbiol. 2015;6:1481. PubMed PMID: 26779139; PubMed Central PMCID: PMC4701948. doi:10.3389/fmicb.2015.01481.
- Paganini D, Zimmermann MB. The effects of iron fortification and supplementation on the gut microbiome and diarrhea in infants and children: a review. Am J Clin Nutr. 2017 Dec;106(Suppl 6):1688S–1693S. PubMed PMID: 29070552; PubMed Central PMCID: PMC5701709. doi:10.3945/ajcn.117.156067.
- Dostal A, Lacroix C, Bircher L, Pham VT, Follador R, Zimmermann MB, Chassard C. Iron modulates butyrate production by a child gut microbiota in vitro. mBio. 2015 Nov 17;6(6):e01453–15. PubMed PMID: 26578675; PubMed Central PMCID: PMC4659462. doi:10.1128/mBio.01453-15.
- Paganini D, Uyoga MA, Kortman GAM, Cercamondi CI, Moretti D, Barth-Jaeggi T, Schwab C, Boekhorst J, Timmerman HM, Lacroix C, et al. Prebiotic galacto-oligosaccharides mitigate the adverse effects of iron fortification on the gut microbiome: a randomised controlled study in Kenyan infants. Gut. 2017 Nov;66(11):1956–1967. PubMed PMID: 28774885. doi:10.1136/gutjnl-2017-314418.
- De-Regil LM, Suchdev PS, Vist GE, Walleser S, Peña-Rosas JP. Home fortification of foods with multiple micronutrient powders for health and nutrition in children under two years of age (Review). Evidence-Based Child Health: A Cochrane RevJ. 2013 Jan;8(1):112–201. PubMed PMID: 23878126. doi:10.1002/ebch.1895.
- WHO. WHO guideline: use of multiple micronutrient powders for point-of-use fortification of foods consumed by infants and young children aged 6–23 months and children aged 2–12 years. English. Geneva (Switzerland): Licence: CC BY-NC-SA 3.0 IGO. ed; 2016.
- Yu T, Zhu C, Chen S, Gao L, Lv H, Feng R, Zhu Q, Xu J, Chen Z, Jiang Z. Dietary high zinc oxide modulates the microbiome of ileum and colon in weaned piglets. Front Microbiol. 2017;8:825. PubMed PMID: 28536569; PubMed Central PMCID: PMC5422713. doi:10.3389/fmicb.2017.00825.
- U KM U, Fatima S. Role of zinc in shaping the gut microbiome; proposed mechanisms and evidence from the literature. J Gastrointest Dig Syst. 2018;8(1):548. doi:10.4172/2161-069X.1000548.
- Krumbeck JA, Walter J, Hutkins RW. Synbiotics for improved human health: recent developments, challenges, and opportunities. Annu Rev Food Sci Technol. 2018 Mar 25;9:451–479. PubMed PMID: 29350558. doi:10.1146/annurev-food-030117-012757.