ABSTRACT
Pseudomonas aeruginosa is one of the leading causes of nosocomial infections worldwide and has emerged as a serious public health threat, due in large part to its multiple virulence factors and remarkable resistance capabilities. Stk1, a eukaryotic-type Ser/Thr protein kinase, has been shown in our previous work to be involved in the regulation of several signalling pathways and biological processes. Here, we demonstrate that deletion of stk1 leads to alterations in several virulence- and resistance-related physiological functions, including reduced pyocyanin and pyoverdine production, attenuated twitching motility, and enhanced biofilm production, extracellular polysaccharide secretion, and antibiotic resistance. Moreover, we identified AlgR, an important transcriptional regulator, as a substrate for Stk1, with its phosphorylation at the Ser143 site catalysed by Stk1. Intriguingly, both the deletion of stk1 and the mutation of Ser143 of AlgR to Ala result in similar changes in the above-mentioned physiological functions. Furthermore, assays of algR expression in these strains suggest that changes in the phosphorylation state of AlgR, rather than its expression level, underlie changes in these physiological functions. These findings uncover Stk1-mediated phosphorylation of AlgR as an important mechanism for regulating virulence and resistance in P. aeruginosa.
Introduction
Pseudomonas aeruginosa is a notorious gram-negative opportunistic pathogen and is one of the most common opportunistic pathogens causing human infections [Citation1]. Owing to its heightened adaptability, invasiveness, motility, and ability to colonize a variety of surfaces and tissues, P. aeruginosa has the potential to instigate outbreaks of clinical infections in hospitals, posing a significant threat to the health and even the lives of patients [Citation2–4]. Recognizing these risks, the World Health Organization has designated P. aeruginosa as a priority for the development of new antibacterial drugs [Citation5].
The pathogenesis of P. aeruginosa involves several stages, including adhesion, colonization, invasion and dissemination [Citation6], and each stage involves multiple virulence factors. Among the pathogenic factors, biofilm formation, motility, pyocyanin (PCN) production and extracellular secretion of virulence factors play important roles in the pathogenicity of P. aeruginosa [Citation7–10]. However, in addition to the above-mentioned virulence factors, the success of P. aeruginosa in initiating infections and causing persistent infections also depends on its rapid development of resistance to various antibiotics through multiple resistance mechanisms [Citation11]. The rapid development of resistance to almost all antibiotics is one of the most striking features of P. aeruginosa, which has led to the worldwide spread of specific multidrug-resistant (MDR) or extensively drug-resistant (XDR) strains [Citation12].
P. aeruginosa possesses a sizable genome (e.g. PAO1 strain is 6.26 Mbp and encodes 5572 genes), which encodes a large number of two-component systems (TCSs) that play important regulatory roles in virulence and resistance [Citation13]. TCS serve as a regulatory mechanism for bacteria to sense and respond to environmental signalling molecules [Citation14] and consists of histidine kinases and response regulatory proteins [Citation15,Citation16]. The histidine kinase, the first type of kinase discovered in bacteria, is localized in the cell membrane and is responsible for sensing external signals [Citation17]. In recent years, however, serine/threonine (Ser/Thr) protein kinases (eukaryotic-like Ser/Thr protein kinases or called Hanks-type kinases) and tyrosine kinases, which are similar to those found in eukaryotic cells, have also been found to be present in bacteria. Reversible phosphorylation of Ser/Thr residues on eukaryotic proteins is an extremely rich dynamic post-translational modification with surprising regulatory and signal transduction functions [Citation18]. However, bacterial proteins exhibit much lower levels of Ser/Thr phosphorylation compared to their eukaryotic counterparts, posing challenges in bacterial phosphorylation studies [Citation19]. Currently, phosphoproteomic characterization has been conducted on at least eight gram-negative bacteria, including P. aeruginosa, and nine gram-positive bacteria. Analysis of these bacterial phosphoproteomic data revealed that on average about 88.9% of phosphorylation sites correspond to Ser/Thr residues (Supplemental Table S1). Moreover, Ser/Thr phosphorylation has been demonstrated to exert broad regulatory roles, including bacterial primary and secondary metabolic processes, virulence, antibiotic resistance, pathogenicity, etc [Citation19], and thus Ser/Thr phosphorylation emerges as a pivotal factor influencing bacterial physiological functions [Citation20]. In fact, the significance of eukaryotic-like Ser/Thr protein kinases is underscored by their crucial regulatory roles in a variety of bacteria, including Staphylococcus aureus [Citation21,Citation22], Diplococcus pneumoniae [Citation23], Bacillus subtilis [Citation24], and Acinetobacter baumannii [Citation25], where they play essential roles in regulating bacterial virulence and resistance.
By genomic analysis, P. aeruginosa PAO1 strain is found to encode several Ser/Thr protein kinases, including PA0074 (PpkA), PA1671 (Stk1), PA5006, PA1782, and PA4780. Two additional genes encoding putative Ser/Thr protein kinases are present in some PAO1 sublines, such as MPAO1 (namely, MPAO1_RS24240 and MPAO1_RS24245; www.pseudomonas.com). Among these, PpkA and Stk1 have been experimentally confirmed as Ser/Thr protein kinases, while the others are predicted to be Ser/Thr protein kinases based on their gene sequence. Extensive research has been conducted on PpkA. Our previous study has demonstrated its role in promoting environmental adaptation and virulence [Citation26]. Other studies indicate its influence on the transcription of stress-responsive genes [Citation27] and its mediation of the phosphorylation of Fha1, a protein with regulatory functions in the type VI secretion system [Citation28]. In contrast, Stk1 has received less attention and has only been studied by Mukhopadhyay et al. [Citation29]: Stk1 was identified as a Ser/Thr protein kinase and three proteins, AlgR (previously termed AlgR1), AlgP (previously termed AlgR3), and IcmF, were speculated to be substrates for Stk1 as they contain one of the signature phosphorylation motifs (SAR or RXT). Of these three proteins, AlgR has been extensively studied and identified as an important transcriptional regulator involved in modulating various physiological functions. AlgP is a protein similar to eukaryotic histone H1 that regulates alginate synthesis [Citation30]. The function of IcmF remains unclear.
In our previous work, we characterized in detail the differentially expressed proteins upon deletion of stk1, and found that Stk1 is involved in the regulation of various signalling pathways and biological processes [Citation31]. In this study, we identified AlgR, which has the SAR (Ser143-Ala144-Arg145) motif, as a substrate of Stk1, and analysed the differences in virulence- and resistance-related physiological functions among stk1 deletion, algRS143A mutation and wild-type P. aeruginosa PAO1 strains. This exploration unveils the Stk1-mediated phosphorylation of AlgR as a novel regulatory mechanism governing virulence and resistance.
Materials and methods
Bacterial stains, plasmids and culture conditions
The bacterial strains of P. aeruginosa PAO1, Δstk1 (PAO1 knockout of stk1, constructed in our previous work [Citation31]), Δstk1:stk1 (Δstk1 containing plasmid pBBR1MCS–2-stk1) and PAO1-algRS143A (replacement of algR in PAO1 by algRS143A) (constructed in this study), and two plasmids, pBBR1MCS–2 and pET-28a(+) were used in this study. The P. aeruginosa and Escherichia coli strains were all cultured at 37 °C in LB broth with shaking at 200 rpm or on agar plates. Antibiotics, kanamycin at 100 μg/mL (for P. aeruginosa) or 50 μg/mL (for E. coli), and chloramphenicol at 25 μg/mL to LB or CTAB (Cetrimide agar), were added as required.
Construction of a mutant strain of AlgR Ser143 mutated to Ala
We construct a site-directed mutant strain (AlgR Ser143 mutated to Ala, designated PAO1-algRS143A) from P. aeruginosa PAO1 strain using a sacB-based strategy [Citation32]. The upstream fragment (498 bp) and the downstream fragment (450 bp) of the mutant site were PCR-amplified using primers algR-up-F1/R1 and algR-down-F2/R2 respectively. These two fragments were then fused (948 bp) and cloned into plasmid pRE112, resulting in recombinant plasmid pRE112-algRS143A. After transfer into E. coli S17–1 (λpir), the recombinant plasmid was subsequently transferred into PAO1 via conjugation. The obtained strains were subjected to PCR amplification using the algR-F3/algR-R3 and sacB-F/R primers, and the strains capable of amplifying the desired fragments were then cultured and screened for successful mutations under sucrose-induced stress conditions. Mutants were further confirmed by PCR using primers algR-F3/algR-R and sacB-F/R. Genomic DNA from strains unable to amplify the desired fragment was extracted and the algR fragment was amplified using primers algR-up-F1 and algR-down-R2 and then sequenced to verify the mutation. All primers are listed in Supplemental Table S2.
Construction of the stk1 complementation strain
The gene fragment of stk1 (1163 bp) was PCR-amplified using PBBR1MCS–2-stk1-F/R primers (Supplemental Table S2). The resulting PCR product was ligated with plasmid PBBR1MCS–2 to obtain the recombinant plasmid PBBR1MCS–2-stk1. This recombinant plasmid was then transferred into the Δstk1 strain by conjugation to obtain the stk1 complementation strain, designated Δstk1:stk1.
Determination of the growth curve of P. aeruginosa strains
Four P. aeruginosa strains PAO1, Δstk1, Δstk1:stk1, and PAO1-algRS143A colonies were inoculated into 5 mL of LB medium and cultured overnight at 37 °C. The resulting bacterial cultures were then seeded 1:100 in 100 mL of LB medium and incubated at 37 °C, and their OD600 absorbance values were measured at 2-hour intervals. The experiment was performed in triplicate.
Homologous modelling of 3D structure
Homology modelling of the 3D structure of AlgR (PA5261) was performed using the protein homology/analogy recognition engine (Phyre) V2.0 online server (http://www.sbg.bio.ic.ac.uk/~phyre2) [Citation33] based on the amino acid sequence. The coloured 3D structure and Ser143 residue were visualized using PyMOL software (v.2.5.5).
Expression and purification of recombinant proteins
For the expression of Stk1, AlgR and AlgRS143A proteins, the constructed pET28a(+)-stk1, pET28a(+)-algR and pET28a(+)-algRS143A vectors were separately transferred into E. coli BL21(DE3) and grown in LB medium supplemented with kanamycin at 37 °C. Bacteria were grown until the OD600 of approximately 0.5 and protein expression was induced by the addition of 0.5 mM isopropyl-D-thiogalactoside (IPTG) for 4 h. The bacterial cells were harvested by centrifugation and suspended in lysis buffer (50 mM Tris-Cl (pH 7.4), 50 mM NaCl, 1 mM EDTA, 10% glycerol), then lysed by sonication followed by centrifugation to obtain total protein samples. The resulting protein samples were submitted to His-tagged Ni-NTA columns and washed with wash buffer (50 mM Tris-Cl (pH 7.4), 300 mM NaCl, 10% glycerol), followed by elution of the proteins with elution buffer (50 mM Tris-Cl (pH 7.4), 150 mM NaCl, 10% glycerol) containing the indicated concentrations of imidazole. The purified proteins were detected by SDS-PAGE, dialysed overnight in dialysis buffer (50 mM Tris-Cl (pH 7.4), 150 mM NaCl, 10 mM MgCl2, 0.5 mM EDTA, 10% glycerol) and their concentrations determined by the BCA method, followed by storage in aliquots at −80 °C until use.
In vitro phosphorylation assay
The in vitro phosphorylation assay was performed as previously described [Citation34]. For phosphorylation reactions, purified Stk1 (2.0 μg) was incubated with purified AlgR (2.0 μg) or AlgRS143A (2.0 μg) in a kinase buffer (50 mM Tris-HCl pH 8.0, 10 mM MgCl2, 1 mM DTT, 2 mM ATP) at 30 °C for 1.5 h or for the indicated times. The reaction was stopped by adding SDS-PAGE sample loading buffer and heating at 100 °C for 5 min, followed by SDS-PAGE. Western blot analysis using anti-phospho-Ser pan antibody (ImmuneChem, China) was then performed to determine the phosphorylation of the substrate proteins. An ultra-sensitive chemiluminescence imager (Tanon 5200 Multi, China) and ECL kits (4A Biotech, China) were used for this assay. The experiments were repeated at least three times.
Extracellular polysaccharide (EPS) secretion assay
Overnight grown cultures of each strain were inoculated 1:100 in fresh LB medium and then incubated to an OD600 of 0.4. Ten microlitres of culture were dropped onto the centre of a plate containing Congo red medium (2% tryptone, 0.004% Congo red, 0.002% coomassie brilliant blue, 1.5% agar) and incubated at 37 °C for 48 h to assess EPS secretion.
Measurement of pyocyanin (PCN) production
PCN was extracted from culture supernatants and measured using previously reported methods [Citation35]. In brief, overnight grown cultures were diluted 1:100 in 5 ml fresh LB medium and were cultured at 37 °C for 24. The supernatants were collected by centrifugation at 5000 rpm for 5 min. PCN was extracted by mixing with 3 mL of chloroform and then re-extraction into 1 mL of 0.2 M HCl. The OD520 value of the PCN-rich aqueous phase was measured. The concentration of PCN was determined by multiplying the OD520 value by 17.072 [Citation35].
Measurement of pyoverdine (PVD) production
PVD production was measured as previously described [Citation36]. Overnight grown bacterial cells were seeded into fresh LB medium and cultured to an OD600 of approximately 1.0, then inoculated at 1:50 into deferrated Casamino Acids (DCAA) medium and cultured at 37 °C for 24 h. The cells were removed by centrifugation and supernatants were diluted with 100 mM Tris-HCl (pH 8.0). PVD in dilutions was measured as OD405 normalized by the cell density of bacterial cultures (OD600).
Iron uptake analysis
Bacterial iron uptake samples were prepared as described in our previous work [Citation37]. Briefly, cultures of wild-type PAO1, Δstk1, and Δstk1:stk1 strains were harvested at the exponential growth phase, washed twice, and then resuspended in 50 mM NaCl to the same cell density (OD600 of ~ 2.0) and kept on ice for 30 min. For Fe3+ uptake assay, 0.3 mL of bacterial suspension was mixed with 0.7 mL of 50 mM NaCl and then warmed by incubation at 37 °C for 10 min. In addition, an equal volume of 50 mM NaCl was used instead of the mixture in the control experiment. The assays were performed in triplicate by the addition of 20 μL of freshly prepared 10 mM FeCl3. After incubating at 37°C for 30 min, the supernatant was collected by centrifugation.
Measurements of Fe3+ concentration were performed using the colorimetric ferrozine assay as described previously [Citation38]. In brief, 800 μL of the resulting supernatant was added to 100 μL of 10 mM ferrozine solution (prepared in 0.1 M ammonium acetate). Subsequently, 800 μL of the mixture was added to 150 μL of 1.4 M hydroxylamine hydrochloride (prepared in 2 M HCl). After 10 min, 50 μL of 10 M ammonium acetate (pH 9.5) was added, and the OD562 value was measured 30 min later. The OD562 values of various concentrations of FeCl3 solutions (in 0.01 M HCl and 50 mM NaCl) were measured to plot a standard curve, which was then used to calculate the concentration of Fe3+.
Twitching motility assay
Twitch motility assay was performed as previously described [Citation39] with a slight modification. The bacteria were cultured to an OD600 of 0.4 in LB medium. The cultures were then stab inoculated to LB plates containing 1% agar with a toothpick through the agar layer to the bottom of the Petri dish. The twitch plates were placed at room temperature for 30 min and then inverted incubation at 37 °C for 24 h. Then, the plates were stained with 0.5% crystal violet for 15 min after removal of the medium, and the diameter of the twitching zone was measured. The experiments were carried out in triplicates.
Quantification of biofilm formation
Biofilm formation was measured using the crystal violet staining approach [Citation40]. Overnight cultures were diluted with fresh LB medium to an OD600 of 0.7. Two hundred microlitres of cultures were added to each well of a 96-well plate and incubated at 37 °C for 24 h. The cultures were gently removed and the wells were washed three times with 200 μL of phosphate buffered saline (PBS). Then, 200 μL of methanol was added to each well to fix the biofilm for 15 min, followed by aspiration of the methanol. After air-drying, the biofilm samples were stained by adding 200 μL of 0.1% w/v crystal violet at 37 °C for 15 min. The crystal violet solution was then removed and the plate was dried at 37 °C. After washing three times with distilled water to remove residual dye, the attached biofilm was suspended in 200 µL of glacial acetic acid. Quantification of biofilms was carried out by measuring OD590 values normalized to the bacterial density of the cultures (OD600). The experiment was carried out in triplicates.
Determination of minimum inhibitory concentration (MIC)
MIC was determined using the previously described method [Citation41]. The bacteria were cultured overnight in cation-adjusted Muller-Hinton broth (CAMHB) medium and then diluted with fresh CAMHB medium to a cell density of 5 × 107 CFU/mL. The diluted culture (10 μL) was added to each well of a 96-well plate in triplicate. Then, 100 μL of different concentrations of antibiotics (prepared in the medium) were added to each well using a 2-fold serial dilution gradient. The plates were then incubated at 37 °C for 20 h, followed by measurement of the OD600 values.
Quantitative real-time PCR (qPCR)
The expression of several genes associated with biofilm formation, virulence and twitching motility in PAO1, Δstk1 and PAO1-algRS143A strains was measured by qPCR. Overnight-grown bacterial cultures were diluted 1:100 in fresh LB medium and incubated until the OD600 of 0.5. Bacterial cells were then harvested, and total RNA was extracted using the Triol method. Following quality assessment through the analysis of the OD260/OD280 ratio, the extracted total RNA was reverse transcribed into cDNA using the Fast Quant RT Kit (gDNase) (Tiangen, China). The qPCR was performed in triplicate using Power SYBR® Green PCR Master Mix (Applied Biosystems, USA) according to the manufacturer’s protocol in an ABI 7500 Real-Time PCR System (USA). The oligonucleotide primers used for qPCR were listed in Supplemental Table S2. The amplification procedure was as follows: denaturation at 95°C for 1 minute, followed by 40 cycles of 95 °C for 10 s, 60 °C for 30 s, and 72 °C for 45 s. The expression of each gene was presented as a relative fold change using the 2−ΔΔ Ct method.
Statistical analysis
All data were expressed as means ± standard deviations (SD) from at least three independent experiments. Data for means between groups were used to perform two-tailed Student’s t-test or one- or two-way ANOVA with GraphPad Prism 9.
Results
Stk1 enhances the production of pyocyanin (PCN) and pyoverdine (PVD), as well as twitching motility
PCN is a blue-green secondary metabolite produced by P. aeruginosa and is a typical virulence factor crucial for pathogenicity [Citation42,Citation43]. To assess the impact of stk1 on PCN production, we examined PCN production in three strains, including wild-type strain PAO1, the stk1-deletion mutant (Δstk1), and the stk1-complementation mutant (Δstk1:stk1), which exhibited similar growth patterns as shown in Supplemental Figure S1. The results showed that the PCN production of Δstk1 was significantly lower than that of PAO1 and Δstk1:stk1 (), suggesting that Stk1 is likely to be involved in regulating the production of PCN. This result is similar to our previous finding that deletion of ppkA, another gene encoding a Ser/Thr protein kinase, also resulted in reduced PCN production [Citation26].
Figure 1. Deletion of stk1 leads to reduced production of PCN and PVD, and attenuated twitching motility.
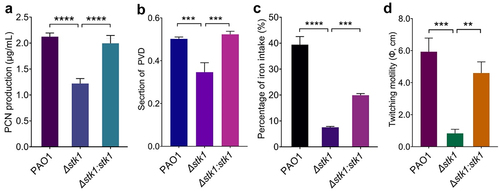
The siderophore pyoverdine (PVD), a small organic compound produced and secreted by P. aeruginosa, acts as both an iron carrier and a virulence-related signalling molecule, and is therefore one of the primary virulence factors [Citation36]. To further determine the effect of Stk1 on P. aeruginosa, we also examined the PVD production and the uptake of iron in the above-mentioned strains. The PVD produced by Δstk1 was found to be significantly lower compared to that of PAO1 and Δstk1:stk1 (). Similarly, Δstk1 displayed reduced Fe3+ uptake compared to PAO1, while Δstk1:stk1 partially restored this capacity (). These two results suggest that Stk1 promotes PVD production and is involved in exogenous iron uptake.
In our previous work, regulatory proteins involved in type IV pilus (T4P), such as FimU, PilW, PilV, PilY1, PilF, PilD and AmrZ were found to be expressed at lower levels in the Δstk1 mutant compared to PAO1 strain [Citation31] (Supplemental Table S3). Consistent with the altered expression of these proteins, the T4P-mediated twitching motility of Δstk1 was also significantly lower than that of PAO1 [Citation31]. Given the lack of data on the stk1 complementation strains, we examined the changes in twitching motility for the above three strains. The twitching motility of Δstk1:stk1 was similar to that of PAO1, but compared to both strains, the twitching motility of Δstk1 was significantly attenuated ( and Supplemental Figure S2), suggesting that Stk1 promotes bacterial twitching motility by regulating T4P.
Stk1 inhibits biofilm formation and extracellular polysaccharide (EPS) secretion
Biofilm is a complex community of bacteria encased in a self-generated matrix of extracellular polymeric substances that attach to biotic or abiotic surfaces during replication [Citation44]. In clinical studies, P. aeruginosa has been found to escape the host immune system or resist antibiotic drug killing by forming biofilms [Citation45–47], and thus biofilm formation is closely related to bacterial pathogenicity and antibiotic resistance. For this purpose, we investigated changes in biofilm formation in the absence of stk1. The results showed that biofilm formation was significantly increased in Δstk1 compared to PAO1 and Δstk1:stk1 (), suggesting that the presence of stk1 inhibits biofilm formation.
Figure 2. Effect of the deletion of stk1 on biofilm production and EPS secretion.
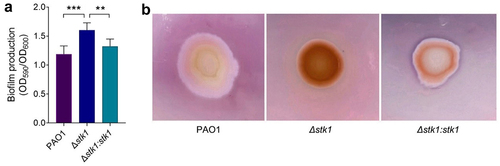
The formation of biofilm is a self-protective growth mode of bacteria that secrete EPS, including alginate, Psl, and Pel, which not only stabilize the biofilm structure, but also improve resistance to antibiotics and the immune system [Citation48,Citation49]. We examined the secretion of EPS by Congo red staining. Colonies of Δstk1 were stained dark red, whereas PAO1 and Δstk1:stk1 were only slightly stained, suggesting that the EPS production capacity of Δstk1 was significantly enhanced compared to that of PAO1 and Δstk1:stk1 (), and this result is consistent with the changes in biofilm formation. Taken together, these results confirmed that Stk1 inhibited the biofilm formation.
Stk1 reduces resistance to ceftazidime, gentamicin and tobramycin
We further determined the minimum inhibitory concentration (MIC) of PAO1, Δstk1 and Δstk1:stk1 strains against four types of Group A antibiotics with FDA clinical indications, including ceftazidime (CAZ), gentamicin (GEN), tobramycin (TOB) and ciprofloxacin (CIP), to investigate the effect of stk1 on bacterial resistance. These antibiotics are typical of each type of antibiotic used in the clinical treatment of P. aeruginosa infections, including β-lactams (CAZ), quinolones (CIP), and aminoglycosides (GEN and TOB) [Citation50,Citation51]. Compared to PAO1 and Δstk1:stk1, Δstk1 showed a significant increase in MIC for three antibiotics, CAZ, GEN and TOB (8-, 8- and 4-fold, respectively) (), promoting bacterial resistance (≥4-fold of MIC), while the MIC for CIP was not significantly changed.
Table 1. MIC of the indicated antibiotics for the strains PAO1, Δstk1, Δstk1:stk1 and PAO1-algRS143A.
AlgR is a substrate of Stk1 and its Ser143 can be phosphorylated by this kinase
Mukhopadhyay et al. [Citation29] identified Stk1 as a Hank’s Ser/Thr protein kinase by finding that Stk1 catalyzes the phosphorylation of histone H1 in an in vitro assay. They also proposed that AlgR has a signature motif, SAR (Ser143-Ala144-Arg145), that can be recognized by the Ser/Thr kinase and is, therefore, a potential substrate for Stk1. However, so far, it is still unclear whether AlgR is a substrate for Stk1. Therefore, to determine AlgR as a substrate of Stk1, we first performed three-dimensional (3D) structural homology modelling of AlgR based on amino acid sequence, and found that the SAR motif is exposed on the surface of the protein and the hydroxyl group on the Ser143 side chain extends to the outside (), indicating that Ser143 of AlgR has the potential to be phosphorylated by Stk1.
Figure 3. AlgR is a substrate of Stk1 and its Ser143 can be phosphorylated by this kinase.
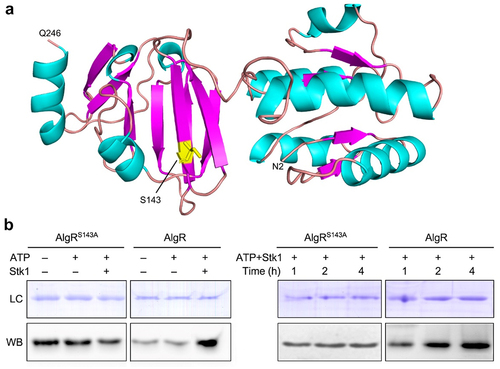
To establish Ser143 as the phosphorylation site of Stk1, we cloned, expressed and purified Stk1, AlgR, and AlgRS143A (the Ser143 of AlgR mutated to Ala) (Supplemental Figure S3), and then performed an in vitro phosphorylation assay. The phosphorylation level of AlgR in the complete reaction system containing AlgR, Stk1 and ATP was much higher than of the control reaction system containing AlgR alone or both AlgR and ATP, whereas in the complete reaction system of AlgRS143A, the phosphorylation level did not change with or without both Stk1 and ATP (, left panel). This result suggests that Ser143 of AlgR can be phosphorylated by Stk1, but it also suggests that when AlgR and AlgRS143A are expressed in E. coli, their other Ser sites are also phosphorylated.
To further verify the phosphorylation of Ser143, we examined the phosphorylation levels at different reaction times, and found that the phosphorylation level of AlgR increased with increasing reaction time, whereas the phosphorylation level of AlgRS143A did not undergo a significant increase (, right panel). Taken together, these results confirm that AlgR is a substrate of Stk1 and that the Ser143 site of AlgR can be phosphorylated by Stk1.
Both deletion of stk1 and algRS143A mutation increased biofilm production, EPS secretion and antibiotic resistance
To investigate whether Stk1 regulates the physiological functions of P. aeruginosa by catalysing the phosphorylation of AlgR, we constructed a mutant strain in which Ser143 of AlgR was mutated to Ala (PAO1-algRS143A, Supplemental Figure S4) and analysed the differences in several physiological functions between this strain and PAO1 and Δstk1. We first compared the differences in the amount of biofilm produced by the three strains and the results showed that Δstk1 and PAO1-algRS143A produced similar amounts of biofilm, but both were significantly higher than PAO1 (). As EPS is an important component of the biofilm, we thus further compared the difference in EPS secretion by these strains and found that Δstk1 and PAO1-algRS143A secreted significantly more EPS than PAO1, although the EPS production of PAO1-algRS143A was slightly lower than that of Δstk1 ().
Figure 4. Both the deletion of stk1 and the mutation of algRS143A led to increased biofilm production, EPS secretion and antibiotic resistance.
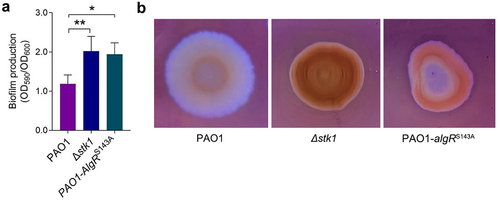
These results indicate that stk1 deletion and algRS143A mutation have similar effects on biofilm and EPS formation, suggesting that the regulatory role of Stk1 is likely mediated by phosphorylation of AlgR. In fact, AlgR has been reported to promote the production of c-di-GMP, an important second messenger in bacteria that regulates various physiological functions, which in turn regulates the biofilm formation [Citation35].
Moreover, since deletion of stk1 resulted in a significant change in MIC to CAZ, GEN and TOB antibiotics, we further compared the MIC between PAO1-algRS143A and Δstk1. The MIC of PAO1-algRS143A to GEN was found to be in agreement with that of Δstk1, but the MIC to CAZ and TOB was slightly lower than that of Δstk1 ().
Both the stk1 deletion and the algRS143A mutation resulted in reduced PCN production and attenuated twitching motility
After incubation of P. aeruginosa for 12 h or longer, a blue-green colour of the bacterial cultures can be clearly observed, which is due to the production and secretion of PCN by the bacterium. As shown in , the blue-green colour of both Δstk1 and PAO1-algRS143A cultures was significantly lighter than that of PAO1, indicating that the PCN production of both Δstk1 and PAO1-algRS143A strains was lower than that of PAO1. To quantify the difference in PCN production by these strains, the amount of PCN was measured at different incubation times. Not surprisingly, Δstk1 and PAO1-algRS143A produced less PCN than PAO1 when incubated for 24 h or longer (). These data indicate that phosphorylation of AlgR by Stk1 has a regulatory effect on PCN production, and that mutations in both Stk1 and AlgR affect PCN production.
Figure 5. Deletion of stk1 and mutation of algRS143A both resulted in decreased PCN production and attenuated twitching motility.
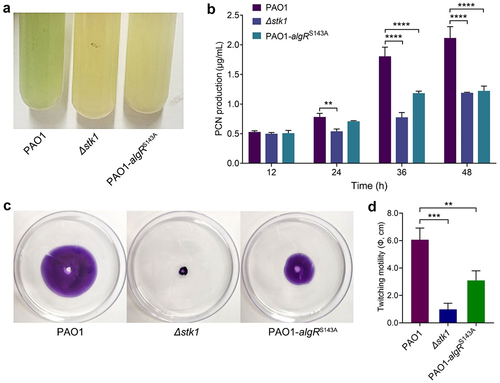
Regarding the twitching motility of these strains, we found that the colony diameters of both Δstk1 and PAO1-algRS143A were significantly smaller than that of PAO1 (), although the diameter of PAO1-algRS143 was slightly larger than that of Δstk1, indicating that the function of T4P of both strains was affected, which in turn weakened the twitching motility. A previous study showed that AlgR activates the transcription of the fimU-pilVWXY1E and regulates the biosynthesis of T4P [Citation52]. Thus, our results suggest that phosphorylation of AlgR is required for its transcriptional activation function, and that Stk1 is the kinase responsible for its phosphorylation.
Three AlgR-controlled genes showed similar expression patterns upon stk1 deletion and algRS143A mutation
AlgR is a transcriptional regulator that is known to control the transcription of genes involved in PCN production, EPS (alginate) secretion and T4P [Citation52], etc. To further investigate whether Stk1 regulates these physiological functions via phosphorylation of AlgR, the expression of genes including czcR, algD, and pilV was examined. The protein encoded by czcR is a negative regulator of PCN [Citation35,Citation53], the protein encoded by algD is a positive regulator of EPS (alginate) [Citation54], and pilV is one of the genes in the T4P assembly operon. Expression analysis of these genes showed that the expressions of czcR and algD were significantly increased in both Δstk1 and PAO1-algRS143A as compared to PAO1, whereas the expression of pilV was significantly decreased (). As expected, the changes in expression of these genes coincided with the changes in physiological functions, in cluding PCN production, EPS secretion and twitching motility. Notably, the expression of algR was not significantly altered in PAO1, Δstk1 and PAO1-algRS143A strains (), suggesting that it is the change in the phosphorylation state of AlgR, rather than in its expression, that accounts for the expression changes in its controlled genes and the resulting changes in physiological function. Taken together, these results demonstrate that Stk1 mediates the phosphorylation of AlgR to regulate virulence- and resistance-related physiological functions.
Figure 6. Determination of the relative expression of algR and several algR-regulated genes in PAO1, Δstk1 and PAO1-algRS143A by qPCR.
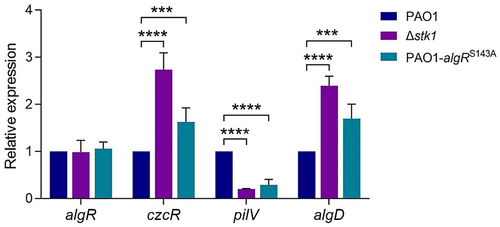
Discussion
In recent years, eukaryotic-like Ser/Thr protein kinases have been identified in a variety of bacteria, and these kinases play key roles in various biological processes [Citation19]. P. aeruginosa has also been found to encode several Ser/Thr protein kinases, such as Stk1 [Citation28]. In our previous work, we found that Stk1 plays important roles in several biological processes [Citation31]. Therefore, in this study, we further investigated the effects of stk1 on various physiological functions associated with bacterial virulence and resistance. As expected, deletion of stk1 resulted in reduced PCN and PVD production and impaired twitching motility (), but promoted biofilm production, EPS secretion, and antibiotic resistance ( and ).
Stk1 has been known as a Ser/Thr protein kinase for two decades [Citation29], but its substrate and physiological function remain unidentified. As a Ser/Thr protein kinase, Stk1 most likely exerts its regulatory function by catalysing the phosphorylation of its substrate protein, which in turn alters the activity of this substrate protein. Since previous studies have shown that Stk1 recognizes the SAR or RXT signature motif [Citation29], and AlgR contains the SAR motif, it is likely that AlgR is one of the substrates of Stk1.
AlgR, which together with the histidine kinase AlgZ (also known as FimS) forms the TCS AlgZR [Citation55], is a response regulator with an unusual LytTR DNA-binding domain [Citation56] and plays an important role in the regulation of gene expression in P. aeruginosa [Citation57]. The phosphorylation state of AlgR has been shown to affect the secretion of PCN [Citation35,Citation55], T4P biosynthesis [Citation52,Citation58,Citation59] or virulence [Citation55]. Moreover, in addition to being phosphorylated by AlgZ, AlgR can also be phosphorylated by other histidine kinases such as CheA and can use carbamoyl phosphate and acetyl phosphate as phosphate donors [Citation60], which leads us to hypothesize that AlgR may also be phosphorylated by other kinases, such as Stk1. To determine whether AlgR is phosphorylated by Stk1, we first modelled the 3D structure of AlgR, and in turn found that the SAR motif is located on the surface of the structure (), indicating that the SAR is capable of binding to Stk1 and thus being phosphorylated by it. This finding strengthens our hypothesis that AlgR is a substrate of Stk1. To verify this hypothesis, we then performed in vitro assays and found that the Ser143 residue of AlgR was indeed phosphorylated by Stk1 (), confirming that AlgR is a substrate for Stk1.
As a transcriptional regulator, AlgR can bind to the promoters of several genes whose expression it controls. It has been shown that phosphorylation of AlgR allows it to bind to more potential sites [Citation61,Citation62], which in turn allows AlgR to control more genes [Citation63,Citation64]. In fact, Kong et al. [Citation35] detected 157 potential loci bound by AlgR in P. aeruginosa genome by using chromatin immunoprecipitation sequencing (ChIP-seq) approach. Of course, there remains the possibility that false positive binding sites were detected due to their assays reliance on overexpressed AlgR [Citation55], and how many of these detected genes are directly controlled by AlgR needs to be further determined.
However, several genes have now been identified that are bound to and controlled by AlgR. For example, AlgR can bind to the promoter sequence of czcR, a response regulator of another TCS, CzcRS, and thus control the expression of this gene [Citation35]. In contrast, CzcR is a transcriptional repressor of several genes involved in PCN biosynthesis, such as phzA1, and therefore AlgR negatively regulates PCN production [Citation53]. We compared PCN production in three strains, PAO1, Δstk1 and PAO1-algRS143A, and found that both Δstk1 and PAO1-algRS143A were much lower than PAO1 (). However, the expression of algR was not significantly altered in either Δstk1 or PAO1-algRS143A (), indicating that the phosphorylation state of AlgR is the important regulator of PCN production.
In addition, AlgR also modulates twitching motility, a flagella-independent mode of solid surface translocation, by regulating the biosynthesis of T4P [Citation65]. Twitching motility is very important for the pathogenicity of P. aeruginosa because the first step in establishing infection is adhesion to and colonization of the epithelium, which is mediated, in part, by T4P [Citation66]. To date, the regulation of T4P biogenesis is well established, namely that AlgR directly activates the expression of the fimU-pilVWXXY1Y2E operon, which is essential for the assembly and export of functional T4P [Citation52]. Further studies showed that only phosphorylated AlgR can activate the fimU-pilVWXY1Y2E operon [Citation52]. Our results indicate that Δstk1 almost lost its twitching motility, and PAO1-algRS143A also had significantly reduced twitching motility (). This change in twitching motility is evidenced by the pilV expression, which is significantly reduced in both strains (). In addition, pilV expression in PAO1-algRS143A, although also significantly reduced, was higher than in Δstk1 (), thus reducing rather than completely eliminating twitching motility. This suggests that phosphorylation of AlgR may not be the only pathway by which Stk1 regulates T4P function.
Furthermore, AlgR is also an important regulator of alginate production. Alginate is an important component of EPS produced by P. aeruginosa [Citation67] and plays a role in the pathogenesis of cystic fibrosis (CF) [Citation68]. The production of high levels of alginate drives the conversion of non-mucilaginous P. aeruginosa to mucilaginous strains, which is a key step in the pathogenesis of CF [Citation69,Citation70]. The key enzyme for alginate synthesis is GDP-mannose dehydrogenase, which is encoded by algD, the first gene in the alginate biosynthetic operon. AlgR binds to the upstream sequence of algD and consequently regulates the expression of the gene [Citation54,Citation71]. AlgD in turn regulates the expression of several related genes, algABC, thereby promoting alginate production [Citation54,Citation71]. Deletion of stk1 and the algRS143A mutation both results in a significant increase in the expression of algD () and the secretion of more EPS (), indicating that the phosphorylation state of AlgR affects the expression of algD, which in turn leads to differences in EPS secretion. In addition, the increased secretion of EPS and up-regulation of algD in both Δstk1 and PAO1-algRS143A were consistent with the finding that both strains had significantly enhanced abilities to form biofilms ().
Conclusion
This study revealed that the Ser/Thr protein kinase Stk1 is involved in the regulation of several virulence- and resistance-related biological processes. Further studies showed that Stk1 controls the expression of genes involved in the physiological functions such as PCN, alginate, biofilm and pilus by catalysing the phosphorylation of the transcriptional regulator AlgR, leading to changes in their physiological functions and antibiotic resistance. These findings not only uncover Stk1-mediated phosphorylation of AlgR as a novel mechanism to promote virulence and resistance in P. aeruginosa, but also contribute to the development of novel antimicrobial drugs or vaccines based on protein kinases or their mediated phosphorylation modifications.
Author contributions
Rui Li: Writing – original draft, Methodology, Validation, Data curation. Xuan Zhu: Writing – original draft, Methodology, Investigation, Data curation, Formal analysis. Pengfei Zhang: Methodology, Investigation, Data curation, Formal analysis. Xuan Wu: Formal analysis, Methodology. Qian Jin: Formal analysis, Methodology. Jianyi Pan: Funding acquisition, Project administration, Supervision, Investigation, Writing – review & editing.
Supplemental Material
Download MS Word (566.7 KB)Disclosure statement
No potential conflict of interest was reported by the author(s).
Data availability statement
The data that support the findings of this study are openly available in figshare at https://doi.org/10.6084/m9.figshare.25551474.v3, reference number 25,551,474.
Supplementary material
Supplemental data for this article can be accessed online at https://doi.org/10.1080/21505594.2024.2367649
Additional information
Funding
References
- Stover CK, Pham XQ, Erwin AL, et al. Complete genome sequence of Pseudomonas aeruginosa PAO1, an opportunistic pathogen. Nature. 2000;406(6799):959–14. doi: 10.1038/35023079
- Hancock RE, Speert DP. Antibiotic resistance in Pseudomonas aeruginosa: mechanisms and impact on treatment. Drug Resist Updat. 2000;3(4):247–255. doi: 10.1054/drup.2000.0152
- Lyczak JB, Cannon CL, Pier GB. Establishment of Pseudomonas aeruginosa infection: lessons from a versatile opportunist. Microbes Infect. 2000;2(9):1051–1060. doi: 10.1016/s1286-4579(00)01259-4
- Gellatly SL, Hancock RE. Pseudomonas aeruginosa: new insights into pathogenesis and host defenses. Pathog Dis. 2013;67(3):159–173. doi: 10.1111/2049-632x.12033
- Tacconelli E, Carrara E, Savoldi A, et al. Discovery, research, and development of new antibiotics: the WHO priority list of antibiotic-resistant bacteria and tuberculosis. Lancet Infect Dis. 2018;18:318–327. doi: 10.1016/s1473-3099(17)30753-3
- Vasil ML. Pseudomonas aeruginosa: Biology, mechanisms of virulence, epidemiology. J Pediatr. 1986;108(5):800–805. doi: 10.1016/S0022-3476(86)80748-X
- Theerthankar D, Jim M. Pseudomonas aeruginosa extracellular secreted molecules have a dominant role in biofilm development and bacterial virulence in cystic fibrosis lung infections, Ch 5. In: Dinesh S, editor. Progress in understanding cystic fibrosis. Rijeka: IntechOpen; 2017. p 5.
- Meirelles LA, Newman DK. Both toxic and beneficial effects of pyocyanin contribute to the lifecycle of Pseudomonas aeruginosa. Mol Microbiol. 2018;110(6):995–1010. doi: 10.1111/mmi.14132
- Khan F, Pham DTN, Oloketuyi SF, et al. Regulation and controlling the motility properties of Pseudomonas aeruginosa. Appl Microbiol Biotechnol. 2020;104(1):33–49. doi: 10.1007/s00253-019-10201-w
- Tuon FF, Dantas LR, Suss PH, et al. Pathogenesis of the Pseudomonas aeruginosa Biofilm: a Review. Pathogens. 2022;11(3):11. doi: 10.3390/pathogens11030300
- Breidenstein EB, de la Fuente-Núñez C, Hancock REW, et al. Pseudomonas aeruginosa: all roads lead to resistance. Trends Microbiol. 2011;19(8):419–426. doi: 10.1016/j.tim.2011.04.005
- Fernández-Billón M, Llamías-Cabot AE, Jordana-Lluch E, et al. Mechanisms of antibiotic resistance in Pseudomonas aeruginosa biofilms. Biofilm. 2023;5:100129. doi: 10.1016/j.bioflm.2023.100129
- Wang BX, Cady KC, Oyarce GC, et al. Two-component signaling systems regulate diverse virulence-associated traits in pseudomonas aeruginosa. Appl Environ Microbiol. 2021;87(11):87. doi: 10.1128/aem.03089-20
- Braun Y, Smirnova AV, Schenk A, et al. Component and protein domain exchange analysis of a thermoresponsive, two-component regulatory system of Pseudomonas syringae. Microbiology (Reading). 2008;154(9):2700–2708. doi: 10.1099/mic.0.2008/018820-0
- Rodrigue A, Quentin Y, Lazdunski A, et al. Two-component systems in Pseudomonas aeruginosa: why so many? Trends Microbiol. 2000;8(11):498–504. doi: 10.1016/s0966-842x(00)01833-3
- Francis S VI, Porter EC, Porter, et al. Two-component systems required for virulence in Pseudomonas aeruginosa. FEMS Microbiol Lett. 2017;364(11):364. doi: 10.1093/femsle/fnx104
- Bleves S, Viarre V, Salacha R, et al. Protein secretion systems in Pseudomonas aeruginosa: a wealth of pathogenic weapons. Int J Med Microbiol. 2010;300:534–543. doi: 10.1016/j.ijmm.2010.08.005
- Sharma K, D’Souza RC, Tyanova S, et al. Ultradeep human phosphoproteome reveals a distinct regulatory nature of Tyr and Ser/Thr-based signaling. Cell Rep. 2014;8(5):1583–1594. doi: 10.1016/j.celrep.2014.07.036
- Yagüe P, Gonzalez-Quiñonez N, Fernánez-García G, et al. Goals and challenges in bacterial phosphoproteomics. Int J Mol Sci. 2019 20;20(22):5678. doi: 10.3390/ijms20225678
- Ge R, Shan W. Bacterial phosphoproteomic analysis reveals the correlation between protein phosphorylation and bacterial pathogenicity. Genomics Proteomics Bioinf. 2011;9(4–5):119–127. doi: 10.1016/s1672-0229(11)60015-6
- Beltramini AM, Mukhopadhyay CD, Pancholi V. Modulation of cell wall structure and antimicrobial susceptibility by a staphylococcus aureus eukaryote-like serine/threonine kinase and phosphatase. Infect Immun. 2009;77(4):1406–1416. doi: 10.1128/iai.01499-08
- Ohlsen K, Donat S. The impact of serine/threonine phosphorylation in Staphylococcus aureus. Int J Med Microbiol. 2010;300(2–3):137–141. doi: 10.1016/j.ijmm.2009.08.016
- Nováková L, Sasková L, Pallová P, et al. Characterization of a eukaryotic type serine/threonine protein kinase and protein phosphatase of streptococcus pneumoniae and identification of kinase substrates. FEBS J. 2005;272(5):1243–1254. doi: 10.1111/j.1742-4658.2005.04560.x
- Soufi B, Kumar C, Gnad F, et al. Stable isotope labeling by amino acids in cell culture (SILAC) applied to quantitative proteomics of Bacillus subtilis. J Proteome Res. 2010;9:3638–3646. doi: 10.1021/pr100150w
- Aranda J, Bardina C, Beceiro A, et al. Acinetobacter baumannii RecA protein in repair of DNA damage, antimicrobial resistance, general stress response, and virulence. J Bacteriol. 2011;193(15):3740–3747. doi: 10.1128/jb.00389-11
- Pan J, Zha Z, Zhang P, et al. Serine/threonine protein kinase PpkA contributes to the adaptation and virulence in Pseudomonas aeruginosa. Microb Pathog. 2017;113:5–10. doi: 10.1016/j.micpath.2017.10.017
- Goldová J, Ulrych A, Hercík K, et al. A eukaryotic-type signalling system of Pseudomonas aeruginosa contributes to oxidative stress resistance, intracellular survival and virulence. BMC Genomics. 2011;12(1):437. doi: 10.1186/1471-2164-12-437
- Sana TG, Hachani A, Bucior I, et al. The second type VI secretion system of Pseudomonas aeruginosa strain PAO1 is regulated by quorum sensing and fur and modulates internalization in epithelial cells. J Biol Chem. 2012;287(32):27095–27105. doi: 10.1074/jbc.M112.376368
- Mukhopadhyay S, Kapatral V, Xu W, et al. Characterization of a Hank’s type serine/threonine kinase and serine/threonine phosphoprotein phosphatase in Pseudomonas aeruginosa. J Bacteriol. 1999;181(21):6615–6622. doi: 10.1128/jb.181.21.6615-6622.1999
- Kato J, Misra TK, Chakrabarty AM. AlgR3, a protein resembling eukaryotic histone H1, regulates alginate synthesis in Pseudomonas aeruginosa. Proc Natl Acad Sci USA. 1990;87(8):2887–2891. doi: 10.1073/pnas.87.8.2887
- Zhu X, Feng C, Zhou L, et al. Impacts of ser/thr protein kinase stk1 on the proteome, twitching motility, and competitive advantage in pseudomonas aeruginosa. Front Microbiol. 2021;12:738690. doi: 10.3389/fmicb.2021.738690
- Liang H, Deng X, Li X, et al. Molecular mechanisms of master regulator VqsM mediating quorum-sensing and antibiotic resistance in Pseudomonas aeruginosa. Nucleic Acids Res. 2014;42:10307–10320. doi: 10.1093/nar/gku586
- Kelley LA, Mezulis S, Yates CM, et al. The Phyre2 web portal for protein modeling, prediction and analysis. Nat Protoc. 2015;10(6):845–858. doi: 10.1038/nprot.2015.053
- Liu H, Ye C, Fu H, et al. Stk and Stp1 participate in Streptococcus suis serotype 2 pathogenesis by regulating capsule thickness and translocation of certain virulence factors. Microb Pathog. 2021;152:104607. doi: 10.1016/j.micpath.2020.104607
- Kong W, Zhao J, Kang H, et al. ChIP-seq reveals the global regulator AlgR mediating cyclic di-GMP synthesis in Pseudomonas aeruginosa. Nucleic Acids Res. 2015;43(17):8268–8282. doi: 10.1093/nar/gkv747
- Imperi F, Tiburzi F, Visca P. Molecular basis of pyoverdine siderophore recycling in Pseudomonas aeruginosa. Proc Natl Acad Sci USA. 2009;106(48):20440–20445. doi: 10.1073/pnas.0908760106
- Ye C, Ge Y, Zhang Y, et al. Deletion of vp0057, a gene encoding a ser/thr protein kinase, impacts the proteome and promotes iron uptake and competitive advantage in vibrio parahaemolyticus. J Proteome Res. 2021;20(1):250–260. doi: 10.1021/acs.jproteome.0c00361
- Viollier E, Inglett PW, Hunter K, et al. The ferrozine method revisited: Fe(II)/Fe(III) determination in natural waters. Appl Geochem. 2000;15(6):785–790. doi: 10.1016/S0883-2927(99)00097-9
- Wu H, Lee B, Yang L, et al. Effects of ginseng on Pseudomonas aeruginosa motility and biofilm formation. FEMS Immunol Med Microbiol. 2011;62(1):49–56. doi: 10.1111/j.1574-695X.2011.00787.x
- Luo J, Dong B, Wang K, et al. Baicalin inhibits biofilm formation, attenuates the quorum sensing-controlled virulence and enhances Pseudomonas aeruginosa clearance in a mouse peritoneal implant infection model. PLOS ONE. 2017;12(4):e0176883. doi: 10.1371/journal.pone.0176883
- Institute CaLS. Methods for dilution antimicrobial susceptibility tests for bacteria that grow aerobically. 9th ed, vol. Approved Standard M07-A9. Wayne (PA): Clinical and Laboratory Standards Institute; 2012.
- Britigan BE, Roeder TL, Rasmussen GT, et al. Interaction of the Pseudomonas aeruginosa secretory products pyocyanin and pyochelin generates hydroxyl radical and causes synergistic damage to endothelial cells. Implications for Pseudomonas-associated tissue injury. J Clin Invest. 1992;90(6):2187–2196. doi: 10.1172/jci116104
- Lau GW, Ran H, Kong F, et al. Pseudomonas aeruginosa pyocyanin is critical for lung infection in mice. Infect Immun. 2004;72(7):4275–4278. doi: 10.1128/iai.72.7.4275-4278.2004
- Rehm BH. Bacterial polymers: biosynthesis, modifications and applications. Nat Rev Microbiol. 2010;8(8):578–592. doi: 10.1038/nrmicro2354
- Lewis K. Riddle of biofilm resistance. Antimicrob Agents Chemother. 2001;45(4):999–1007. doi: 10.1128/aac.45.4.999-1007.2001
- Thi MTT, Wibowo D, Rehm BHA. Pseudomonas aeruginosa Biofilms. Int J Mol Sci. 2020;21(22):21. doi: 10.3390/ijms21228671
- Reig S, Le Gouellec A, Bleves S. What is new in the anti-pseudomonas aeruginosa clinical development pipeline since the 2017 WHO Alert? Front Cell Infect Microbiol. 2022;12:909731. doi: 10.3389/fcimb.2022.909731
- Rasamiravaka T, Labtani Q, Duez P, et al. The formation of biofilms by Pseudomonas aeruginosa: a review of the natural and synthetic compounds interfering with control mechanisms. Biomed Res Int. 2015;2015:759348. doi: 10.1155/2015/759348
- Srinivasan R, Santhakumari S, Poonguzhali P, et al. Bacterial biofilm inhibition: a focused review on recent therapeutic strategies for combating the biofilm mediated infections. Front Microbiol. 2021;12:676458. doi: 10.3389/fmicb.2021.676458
- Valerius NH, Koch C, Høiby N. Prevention of chronic Pseudomonas aeruginosa colonisation in cystic fibrosis by early treatment. Lancet. 1991;338(8769):725–726. doi: 10.1016/0140-6736(91)91446-2
- Roudashti S, Zeighami H, Mirshahabi H, et al. Synergistic activity of sub-inhibitory concentrations of curcumin with ceftazidime and ciprofloxacin against Pseudomonas aeruginosa quorum sensing related genes and virulence traits. World J Microbiol Biotechnol. 2017;33(3):50. doi: 10.1007/s11274-016-2195-0
- Belete B, Lu H, Wozniak DJ. Pseudomonas aeruginosa AlgR regulates type IV pilus biosynthesis by activating transcription of the fimU-pilVWXY1Y2E operon. J Bacteriol. 2008;190(6):2023–2030. doi: 10.1128/jb.01623-07
- Dieppois G, Ducret V, Caille O, et al. The transcriptional regulator CzcR modulates antibiotic resistance and quorum sensing in Pseudomonas aeruginosa. PLOS ONE. 2012;7(5):e38148. doi: 10.1371/journal.pone.0038148
- Wozniak DJ, Ohman DE. Transcriptional analysis of the Pseudomonas aeruginosa genes algR, algB, and algD reveals a hierarchy of alginate gene expression which is modulated by algT. J Bacteriol. 1994;176(19):6007–6014. doi: 10.1128/jb.176.19.6007-6014.1994
- Little AS, Okkotsu Y, Reinhart AA, et al. Pseudomonas aeruginosa Algr phosphorylation status differentially regulates pyocyanin and pyoverdine production. MBio. 2018;9(1):9. doi: 10.1128/mBio.02318-17
- Nikolskaya AN, Galperin MY. A novel type of conserved DNA-binding domain in the transcriptional regulators of the AlgR/AgrA/LytR family. Nucleic Acids Res. 2002;30(11):2453–2459. doi: 10.1093/nar/30.11.2453
- Lizewski SE, Lundberg DS, Schurr MJ. The transcriptional regulator AlgR is essential for Pseudomonas aeruginosa pathogenesis. Infect Immun. 2002;70(11):6083–6093. doi: 10.1128/iai.70.11.6083-6093.2002
- Whitchurch CB, Erova TE, Emery JA, et al. Phosphorylation of the Pseudomonas aeruginosa response regulator AlgR is essential for type IV fimbria-mediated twitching motility. J Bacteriol. 2002;184(16):4544–4554. doi: 10.1128/jb.184.16.4544-4554.2002
- Marko VA, Kilmury SLN, MacNeil LT, et al. Pseudomonas aeruginosa type IV minor pilins and PilY1 regulate virulence by modulating FimS-AlgR activity. PLOS Pathog. 2018;14(5):e1007074. doi: 10.1371/journal.ppat.1007074
- Deretic V, Leveau JH, Mohr CD, et al. In vitro phosphorylation of AlgR, a regulator of mucoidy in Pseudomonas aeruginosa, by a histidine protein kinase and effects of small phospho-donor molecules. Mol Microbiol. 1992;6(19):2761–2767. doi: 10.1111/j.1365-2958.1992.tb01455.x
- Okkotsu Y, Tieku P, Fitzsimmons LF, et al. Pseudomonas aeruginosa AlgR phosphorylation modulates rhamnolipid production and motility. J Bacteriol. 2013;195(24):5499–5515. doi: 10.1128/jb.00726-13
- Okkotsu Y, Little AS, Schurr MJ. The Pseudomonas aeruginosa AlgZR two-component system coordinates multiple phenotypes. Front Cell Infect Microbiol. 2014;4:82. doi: 10.3389/fcimb.2014.00082
- Whitchurch CB, Alm RA, Mattick JS. The alginate regulator AlgR and an associated sensor FimS are required for twitching motility in Pseudomonas aeruginosa. Proc Natl Acad Sci USA. 1996;93(18):9839–9843. doi: 10.1073/pnas.93.18.9839
- Stacey SD, Williams DA, Pritchett CL, et al. The Pseudomonas aeruginosa two-component regulator AlgR directly activates rsma expression in a phosphorylation-independent manner. J Bacteriol. 2017;199(18). doi: 10.1128/jb.00048-17
- Mattick JS. Type IV pili and twitching motility. Annu Rev Microbiol. 2002;56(1):289–314. doi: 10.1146/annurev.micro.56.012302.160938
- Comolli JC, Hauser AR, Waite L, et al. Pseudomonas aeruginosa gene products PilT and PilU are required for cytotoxicity in vitro and virulence in a mouse model of acute pneumonia. Infect Immun. 1999;67(7):3625–3630. doi: 10.1128/iai.67.7.3625-3630.1999
- Mann EE, Wozniak DJ. Pseudomonas biofilm matrix composition and niche biology. FEMS Microbiol Rev. 2012;36(4):893–916. doi: 10.1111/j.1574-6976.2011.00322.x
- Pedersen SS, Kharazmi A, Espersen F, et al. Pseudomonas aeruginosa alginate in cystic fibrosis sputum and the inflammatory response. Infect Immun. 1990;58(10):3363–3368. doi: 10.1128/iai.58.10.3363-3368.1990
- Terry JM, Piña SE, Mattingly SJ. Role of energy metabolism in conversion of nonmucoid Pseudomonas aeruginosa to the mucoid phenotype. Infect Immun. 1992;60(4):1329–1335. doi: 10.1128/iai.60.4.1329-1335.1992
- Wood LF, Leech AJ, Ohman DE. Cell wall-inhibitory antibiotics activate the alginate biosynthesis operon in Pseudomonas aeruginosa: roles of σ 22 (AlgT) and the AlgW and Prc proteases. Mol Microbiol. 2006;62(2):412–426. doi: 10.1111/j.1365-2958.2006.05390.x
- Lizewski SE, Schurr JR, Jackson DW, et al. Identification of AlgR-regulated genes in Pseudomonas aeruginosa by use of microarray analysis. J Bacteriol. 2004;186(17):5672–5684. doi: 10.1128/jb.186.17.5672-5684.2004