ABSTRACT
Dendritic cells (DCs) present an ideal target for delivering immunogenic cargo due to their potent antigen-presenting capabilities. This targeting approach holds promise in vaccine development by enhancing the efficiency of antigen recognition and capture by DCs. To identify a high-affinity targeting peptide binding to rabbit DCs, rabbit monocyte-derived DCs (raMoDCs) were isolated and cultured, and a novel peptide, HS (HSLRHDYGYPGH), was identified using a phage-displayed peptide library. Alongside HS, two other DC-targeting peptides, KC1 and MY, previously validated in our laboratory, were employed to construct recombinant Lactgobacillus reuteri fusion-expressed rabbit hemorrhagic disease virus (RHDV) capsid protein VP60. These recombinant Lactobacillus strains were named HS-VP60/L. reuteri, KC1-VP60/L. reuteri, and MY-VP60/L. reuteri. The ability of these recombinant Lactobacillus to bind rabbit DCs was evaluated both in vivo and in vitro. Results demonstrated that the DC-targeting peptide KC1 significantly enhanced the capture efficiency of recombinant Lactobacillus by raMoDCs, promoted DC maturation, and increased cytokine secretion. Furthermore, oral administration of KC1-VP60/L. reuteri effectively induced SIgA and IgG production in rabbits, prolonged rabbit survival post-challenge, and reduced RHDV copies in organs. In summary, the DC-targeting peptide KC1 exhibited robust binding to raMoDCs, and recombinant Lactobacillus expressing KC1-VP60 protein antigens efficiently induced systemic and mucosal immune responses in rabbits, conferring protective efficacy against RHDV. This study offers valuable insights for the development of novel RHDV vaccines.
Introduction
Rabbit hemorrhagic disease (RHD) poses a significant threat to both domestic and wild rabbits (Oryctolagus cuniculus), leading to acute and fulminating infections that result in substantial financial losses within the rabbit product trade [Citation1,Citation2]. The causative agent, RHD virus (RHDV), belongs to the family Caliciviridae and is a non-enveloped icosahedral virus. Its genome consists of a positive-sense single-stranded RNA of 7.5 kb, housing two slightly overlapping open reading frames (ORFs): ORF1 and ORF2 [Citation3,Citation4]. ORF1 encodes the major structural capsid protein (VP60, also known as VP1) and various nonstructural proteins (NSP1–7) [Citation2]. Additionally, VP60 may also be expressed from a subgenomic RNA of approximately 2.4 kb, is a main target of host immune defense against RHDV, and plays a significant role in virus diagnosis and vaccine design [Citation5,Citation6]. Despite the challenges of growing RHDV in cell culture, current vaccination strategies involve chemically inactivating crude virus preparations obtained from diseased rabbit livers. Although effective, this method raises safety and ethical concerns [Citation7,Citation8]. Consequently, the cloning and expression of major immunogenic antigens, such as RHDV VP60, in protein expression systems offer promising avenues for RHDV vaccine research. Notably, RHDV VP60 has been successfully expressed in various heterologous systems, including bacteria, yeasts, plants, poxvirus-based vectors, and insect cells, as reported in early studies [Citation9–20].
In our previous study, we utilized an oral Lactobacillus vaccine as an antigen delivery system to express the RHDV capsid protein VP60, resulting in the induction of immune responses against RHDV in rabbits [Citation21]. Employing Lactobacillus as a delivery vector for oral vaccines to transport heterogeneous antigens offers numerous advantages. Lactobacillus can adapt to the conditions encountered during transit through the gastrointestinal tract, including bile and low pH, thereby enhancing the immune response and preserving mucosal barrier function [Citation22–27].
However, compared to other expression vectors such as Escherichia coli, yeast, and insect expression vectors, Lactobacillus faces a challenge: the production of a lower amount of antigen. Consequently, it may not induce a sufficiently robust immune response. To elicit strong immunity against pathogens, antigen-presenting cells (APCs) must efficiently capture vaccine antigens for activation [Citation28–30]. Among various types of APCs, dendritic cells (DCs) stand out as the most efficient and promising. They capture antigens and pathogens, generate MHC-peptide complexes, express co-stimulatory molecules like CD80/CD86, undergo maturation from immature DCs to mature DCs (mDCs), and ultimately mediate T cell activation for antibody production [Citation31–34].
Numerous studies have highlighted that screening and identifying peptides binding to DCs is an effective strategy for developing DC-targeting vaccines [Citation35–38]. Over two decades ago, specific human DC-binding peptides were identified using a phage display peptide library. This discovery led to the development of a new mucosal vaccination platform that combines DC-targeting peptides with specificity and orally delivered Lactobacillus [Citation39]. This innovative approach efficiently induces mucosal and humoral immune responses through oral vaccination [Citation40]. In recent years, a growing body of experiments has supported the strategy of expressing DC-targeting peptides in recombinant Lactobacillus to enhance the efficiency of DC capture by recombinant Lactobacillus [Citation41–46].
In this study, we aimed to screen the optimal DC-targeting peptide for constructing recombinant lactobacilli expressing the DC-targeting peptide fused with the RHDV capsid protein VP60. We evaluated the ability of these recombinant lactobacilli to induce an immune response and their potential to protect rabbits from RHDV infection. Our findings suggest that targeting DCs is a promising strategy for developing vaccines against RHDV in rabbits.
Materials and methods
Bacteria and plasmids
Rabbit-derived Lactobacillus reuteri (R-L. Reu (21)-4) was isolated from 8-week-old rabbits and cultured in de Man, Rogosa, and Sharpe medium (MRS; Hopebol, Qingdao, China) without shaking. The constitutive expression plasmid pPG-T7g10-PPT, constructed in our laboratory, contained the robust HCE constitutive promoter, T7g10 transcriptional enhancer, pgsA anchor from Bacillus subtilis for stabilizing the heterologous protein on the cell membrane (surface-displaying), and rrnBT1T2 terminator. The pMD19T-VP60 recombinant plasmid, also constructed and preserved in our laboratory, contained VP60 obtained from the RHDV strain isolated and identified from clinical samples.
Animals
Three-month-old Japanese white rabbits purchased from Acheng Experimental Practice Base of Northeast Agricultural University (Harbin, China) were kept under SPF conditions with free access to standard water and diet. The animals did not exhibit abnormal behavior, death, or near death during the observation period before the experiment.
Peptide sequence
Rabbit DC-targeted peptide HS (HSLRHDYGYPGH), porcine DC-targeted peptide KC1 (KCCYPN), MY (MYPPPY), and negative control peptide (YPLPYP) were synthesized by Jinsiri Biotechnology (Nanjing, Jiangsu) and purified using high-performance liquid chromatography (purity >95%). The N-terminus was labeled with fluorescein isothiocyanate (FITC).
Isolation and identification of rabbit monocyte-derived DCs
Rabbit Peripheral blood mononuclear cells (PBMCs) were isolated from heparinized whole blood by density gradient centrifugation using Lymphosep separation medium (TBD, LTS10965), PBMCs were incubated with 20 ng/mL recombinant human granulocyte-macrophage colony-stimulating factor (GM-CSF; BD Biosciences 550,068) and 25 ng/mL recombinant human interleukin 4 (IL-4; BD Biosciences 554,605), according to previously described protocol for detailed methods [Citation47].
Morphological changes in the immature rabbit monocyte-derived DCs (raMoDCs) were observed using an optical microscope. The cells were washed thrice with PBS, then fixed with 2% glutaraldehyde at 4°C and washed thrice with PBS. The samples were freeze-dried after dehydration and dealcoholization, photographed, and analyzed using an SU820 scanning electron microscope (Hitachi, Tokyo, Japan).
Maturation of immature raMoDCs (imraMoDCs) was induced by supplementing with 2 mg/mL lipopolysaccharide (LPS; Sigma-Aldrich, L2880) for 24 h. The surface expression of the marker molecules CD86 (Abcam, ab234226) and MHC-II (Abcam, ab18232) was analyzed using fluorescence microscopy (Bio-Rad, CA, USA) on the sixth day. MHC-II, CD86, and CD14 (Abcam, ab314410) expressed on the surface of imraMoDCs or mature raMoDCs (mraMoDCs) were analyzed using flow cytometry.
ImraMoDCs collected after 6 days of culture in sterile centrifuge tubes were centrifuged at 600 × g for 10 min. One tube was added with a final concentration of 100 μg/mL of FITC-labeled glucose (BD Biosciences 569,321) and incubated in the dark at 37°C for 3 h. An equal amount of dextran was added to another tube and incubated in the dark at 4°C as a negative control, and cells without dextran were set as a blank control. After incubation, cells were washed three times with PBS to remove non-specific binding. Phagocytic capacity was detected using flow cytometry after resuspending cells in 500 µL of sterile PBS.
Screening of raMoDC-targeting peptides by phage display
The Ph.D.-12 phage display library (NEB, E8210S), displaying a linear 12-mer random peptide on the N-terminus of the PIII protein of bacteriophage M13, was utilized to screen for the DC-targeting peptide. Screening of raMoDCs using in vitro cell screening method, differential screening used PBMCs as negative selection cells, according to previously described protocol for detailed methods [Citation29].
Determination of binding ability of selected phages using enzyme-linked immunosorbent assay, fluorescence microscope, and flow cytometry
PBMCs and raMoDCs were fixed on 0.03 mg/mL polylysine-coated 96-well plates for 30 min at 25°C. Cells were blocked with PBS (2% w/v BSA) for 30 min at 4°C. Phage clones (1010 pfu/mL) were added to wells and incubated at 4°C for 20 h. PBS and wild M13 phages served as negative controls. After three washes with PBST, cells were incubated with mouse anti-M13 bacteriophage polyclonal antibody (diluted 1:1,500 in 2% BSA, LSBiological, LS-C146750) at 37°C for 40 min. Subsequently, the cells were incubated with horseradish peroxidase (HRP)-labeled mouse anti-goat IgG antibody (diluted 1:4,000, ZSGB Biotech, ZB-2305). Finally, the cells were washed with PBST, and color development was initiated using a 3,3’,5,5’-tetramethylbenzidine enzyme substrate. The absorbance at OD450 was measured.
On day 6 of raMoDC cultivation, the complete medium was gently aspirated using pipettes, and the cells were washed twice with PBS. RaMoDCs were incubated with FITC-labeled rabbit DC-targeted peptide HS, porcine DC-targeted peptides KC1 and MY, and FITC-labeled negative control peptide for 30 min at 4°C. CD86-PE monoclonal antibody labeled DCs were added to each sample and incubated at 37°C in the dark for 30 min. After being washed three times with PBS, the raMoDCs were fixed with 4% paraformaldehyde for 5 min at 37°C and observed under a fluorescence microscope (Bio-Rad, CA, USA). FITC-labeled peptide (25 µg) was incubated with 106 raMoDCs at 4°C for 10 min. After washing three times with PBS, the cells were subjected to flow cytometry (BD Biosciences, San Jose, CA, USA).
Construction of recombinant Lactobacillus
The VP60 coding sequence (sequence ID: AF240686.1) was amplified from the RHDV cDNA using PCR. HS (HSLRHDYGYPGH), KC1 (KCCYPN), MY (MYPPPY), and the control peptide (YPLPYP) were separately fused to the C-terminus of VP60 using the primers listed in . The HS-VP60, KC1-VP60, and MY-VP60 genes were amplified using forward and reverse primers (HS-F/R, KC1-F/R, and MY-F/R, respectively), with the plasmid pMD19T-VP60 as the template. PCR amplification was performed as follows: 95°C for 5 min; 30 cycles at 98°C for 10 s, 55°C for 1 min, 68°C for 30 s, and 68°C for a final extension of 10 min. PCR products were confirmed by DNA sequencing. Subsequently, HS-VP60, KC1-VP60, and MY-VP60 were cloned into the expression plasmid pPG-T7g10-PPT to generate pPG-HS-VP60, pPG-KC1-VP60, and pPG-MY-VP60, respectively. The flag tag was inserted into the HS-VP60, KC1-VP60, MY-VP60, and VP60 fusion genes, and a recombinant expression plasmid was constructed. To construct a recombinant Lactobacillus strain, recombinant plasmids were electrotransferred into L. reuteri and verified by PCR, double digestion, and sequencing.
Table 1. Sequence of Primers.
Restriction enzyme recognition sites used for cloning are shown in bold and italics. DC-targeting peptides are shown in bold. Rigid amino acids are underlined.
Protein expression and identification
Protein expression was analyzed by western blotting and indirect immunofluorescence. Recombinant Lactobacillus were broken down by ultrasound and subjected to electrophoresis using 10% SDS-PAGE. Mouse anti-FLAG monoclonal antibody (diluted 1:1,000; Abcam, ab125243) served as the primary antibody at 4 °C overnight, and HRP-conjugated goat anti-mouse IgG (diluted 1:5,000; ZSGB Biotech, ZB-2305) functioned as the secondary antibody at 37 °C for 30 min. Moreover, HS-VP60/L. reuteri, KC1-VP60/L. reuteri, MY-VP60/L. reuteri, VP60/L. reuteri, and vector/L. reuteri were cultured overnight at 37°C in MRS broth containing chloramphenicol (10 μg/mL). Using indirect immunofluorescence assay (IFA) to identify the expression of recombinant lactic acid bacteria, the primary antibody is anti-FLAG-tagged mouse monoclonal antibody (diluted 1:1,000), and the secondary antibody is FITC-conjugated anti-mouse IgG secondary antibodies (diluted 1:250). The experimental method for IFA can be referred to previously described protocol [Citation47].
Analysis of raMoDC-targeting ability of recombinant Lactobacillus
Cultivation of recombinant Lactobacillus
Recombinant Lactobacillus were cultured overnight at 37°C in MRS broth at a ratio of 1:50. The bacterial pellet was collected by centrifugation at 1,500 × g for 5 min, washed thrice with sterile PBS, and resuspended in RPMI-1640. Recombinant Lactobacillus were then used to stimulate raMoDCs.
Field-emission scanning electron microscope
RaMoDCs were cultured for 6 days, and recombinant Lactobacillus were incubated on coverslips for 30, 60, and 120 min. The cells were washed three times with PBS to remove excess recombinant Lactobacillus that did not adhere. RaMoDCs were then fixed with 2% glutaraldehyde at 4°C and washed thrice with PBS. The samples were freeze-dried after dehydration and dealcoholization, photographed, and analyzed using an SU820 SEM (Hitachi, Tokyo, Japan).
Quantitative real-time polymerase chain reaction analysis of mRNA expression of toll-like receptors and cytokines in raMoDCs
RaMoDCs were incubated with HS-VP60/L. reuteri, KC1-VP60/L. reuteri, MY-VP60/L. reuteri, or VP60/L. reuteri, and vector/L. reuteri, and LPS at 37°C for 12 h. Total RNA was extracted from non-stimulated raMoDCs and raMoDCs incubated with LPS and recombinant Lactobacillus using an RNAprep Pure Micro Kit (TIANGEN, DP419), following the manufacturer’s instructions. The complementary DNA template for quantitative real-time polymerase-chain reaction (qRT-PCR) was obtained by reverse transcription, and primers for qRT-PCR were designed using Primer 5 (). The experimental method for qRT-PCR can be performed according to a previously described protocol [Citation47]. Relative mRNA expression was calculated using the formula delta Ct (ΔΔCt) = (Ct (stimulated-raMoDCs, target gene) – Ct (stimulated-raMoDCs, β-actin)) – (Ct (non-stimulated-raMoDCs, target gene) – Ct (non-stimulated-raMoDCs, β-actin)). The results were calculated by 2–ΔΔCt.
Table 2. Details of the specific primer sequences used for qPCR experiments.
Animal grouping and immunizing procedure
Recombinant Lactobacillus was cultured in MRS medium for 16 h without agitation, washed with sterile PBS, and resuspended in PBS to a final concentration of 1010 colony-forming units (CFU)/mL. In this study, rabbits were individually numbered and then grouped using a random number table. Twenty rabbits were divided into five groups. Four rabbits were orally immunized with a mixture of 5 × 1010 CFU/mL pPG-HS-VP60/L. reuteri, KC1-VP60/L. reuteri, MY-VP60/L. reuteri, and VP60/L. reuteri (immunization group), the immune dose of Lactobacillus is based on our previous research [Citation21]. Four rabbits were orally administered 5 mL of sterile PBS (PBS group). In the challenge experiment, 12 rabbits were divided into three groups: four rabbits were orally administered 5 mL of sterile PBS (PBS group), 5 × 1010 CFU/mL KC1-VP60/L. reuteri (immunization group), and four rabbits were not immunized. On the 14th day of immune with KC1-VP60/L. reuteri, four rabbits were subcutaneously injected with 1 mL of RHDV liver grinding solution in the neck and raised in the immunization group, PBS group, and rabbits injected with RHDV in the same cage (). When analyzing data, experimenters and animal operators do not know which group of rabbits are immunized with which Lactobacillus or PBS to reduce the impact of subjective bias. This study was carried out in accordance with the recommendations in the Guide for Animal Research: Reporting of In Vivo Experiments (ARRIVE) [Citation48]. The protocol was approved by the Ethical Committee for Animal Experiments at Northeast Agricultural University, China.
Figure 1. Schematic diagram of the immunization, challenge, and cohabitation of rabbits. Twenty rabbits were divided into five groups for immunization. Twelve rabbits were divided into three groups for challenge. The box indicates rabbits in the orally immunized KC1-VP60/L. reuteri (Dark Pink), PBS (blue), and rabbits infected with RHDV (green) were raised in the same cage.
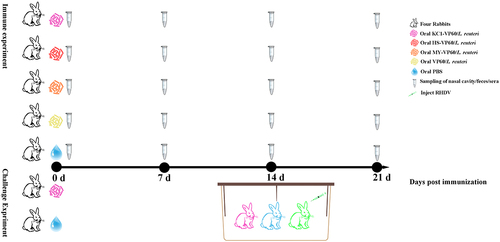
Anti-RHDV specific sIgA levels and serum-specific IgG levels of immunized rabbits
After immunization, anal and nasal swabs of rabbits were collected daily and soaked in 1 mL of cold sterile PBS at 4°C overnight. After centrifugation at 10,000 × g for 10 min at 4°C, the supernatant was stored at −40°C. Specific sIgA levels in the samples were detected using ELISA [Citation49]. The experimental method for ELISA was performed according to a previously described protocol [Citation47]. Blood was collected from the immunized rabbits through the ear vein before immunization and on the 7th, 14th, and 21st days after the initial immunization. The blood was stimulated at 37°C for 1 h, left overnight at 4°C, and centrifuged at 4°C at 10,000 × g for 5 min. Serum was prepared, and serum-specific IgG responses were measured using ELISA. The method was the same as that for sIgA, but the secondary antibody was HRP-conjugated goat anti-rabbit IgG (diluted 1:5,000; ZSGB Biotech, ZB-2306)
Challenge experiment and viral load in infected tissue detection
After raising the immunization group, PBS group, and rabbits injected with RHDV in the same cage, the mental state, appetite, diarrhea, and other clinical symptoms of the rabbits were recorded. The time of death of the rabbits was also recorded. The constructed recombinant-positive plasmid pMD-19T Simple-VP60 was transformed into a standard substance in a 10-fold gradient. RT-qPCR amplification was performed using plasmids of various gradients as templates, and software was used for analysis to obtain standard curves. cDNA was obtained from the liver, spleen, lungs, and kidneys of the infected rabbits. Using the obtained cDNA as a template, three parallel RT-qPCR assays were designed to detect the viral load in the infected tissues. Configure the RT-qPCR system with a reaction program of 95°C for 10 min, enter the cycle at 95°C for 15 s, 60°C for 1 min, and 40 cycles.
Statistical analysis
Experimental data were statistically analyzed using GraphPad Prism (version 8.0.2). Data were presented as mean ± standard deviation and analyzed using two-way ANOVA with multiple comparison tests using SPSS. The different letters (a vs. b, a vs. c, and b vs. c) indicate significant differences (p < 0.01) at the same time point.
Results
Isolation and characterization of raMoDCs
To obtain raMoDCs, rabbit PBMCs were cultured in the presence of recombinant human GM-CSF (rhGM-CSF) and rhIL-4 for 5 days, followed by LPS stimulation for 1 day. Morphological changes were observed on days 1, 2, 6, and 7 using both optical microscopy and scanning electron microscopy. The isolated monocytes initially appeared round, with cell aggregates forming on day 2. By day 6, most cells exhibited short dendrites, and upon LPS stimulation on day 7, the cells became larger with irregular shapes, forming long pseudopodia – characteristic of mDCs morphology ().
Figure 2. Typical morphology and molecular phenotype of raMoDCs(a) morphology of imraMoDCs (Day 6) and mraMoDCs (Day 7, treated with LPS for 24 h for maturation) observed through optical microscopy and scanning electron microscopy. (b) Immunostaining of imraMoDCs and mraMoDCs for MHC-II (green), CD86 (red), and nuclei stained with DAPI (blue). (c) Flow cytometry analysis showing the expression of surface markers (CD172a, MHC-II, CD86, and CD14) on imraMoDCs and mraMoDCs. (d) Flow cytometry assessment of the phagocytic ability of raMoDCs toward FITC-labeled glucans. The data reflect % abundance for positive populations. Brightness, contrast, or color balance adjustments were uniformly applied to all microscopy images.
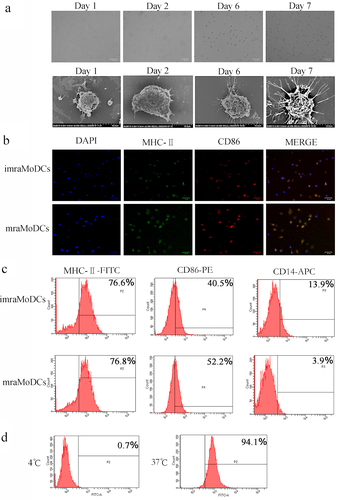
To further characterize raMoDCs, the expression of surface marker molecules was assessed using fluorescence microscopy and flow cytometry. As expected, both imraMoDCs and mraMoDCs expressed MHC-II, CD86, and CD14. The expression of CD86 was notably higher in mraMoDCs compared to imraMoDCs (). Additionally, the phagocytic ability of raMoDCs toward FITC-labeled glucans was confirmed using flow cytometry, indicating their functional capacity (). These results demonstrate that raMoDCs derived from PBMCs exhibit typical DC characteristics and are suitable for further experimentation.
Generation and characterization of raMoDC-targeting peptide
To identify peptide ligands specifically targeting raMoDCs, four rounds of phage display biopanning were conducted. Phages unbound to PBMCs were added to raMoDCs in each round to minimize non-specific binding. The stability of phage recovery rates across rounds () suggests effective screening.
Table 3. Determination of phage recovery rate.
In the fourth round, 200 randomly selected phages were sequenced (), revealing five frequently occurring phage isolates (). This indicates that these five peptides exhibit enhanced binding to raMoDCs. To validate this, the binding ability of these phages was assessed using ELISA (). The selected phages demonstrated significant binding to raMoDCs, with minimal binding to PBMCs. Among them, the clone HS exhibited the highest binding affinity (p < 0.01).
Figure 3. Analysis of phage binding ability to raMoDCs by ELISA. (a) Sequences of 12-mer peptides. (b) Cell-ELISA assessing the binding selectivity to raMoDCs of eight phage clones from the last round of biopanning. M13 wild-type phage without any displayed peptide served as a negative control. Data are presented as the mean ± SD of three independent experiments. Significant differences are denoted by different letters (a vs. b, a vs. c, b vs. c) at the same time point (p < 0.01).
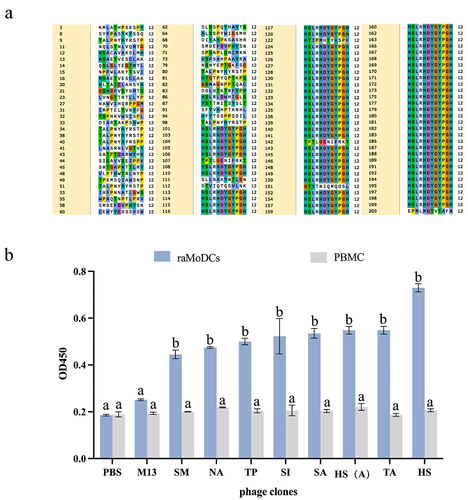
Table 4. Sequences and frequencies of peptides.
Porcine DC-targeting peptide effectively binds to RaModcs
In the pursuit of a high-affinity targeting peptide for raMoDCs, porcine DC-targeting peptides, namely KC1 (unpublished) and MY, were compared with HS. Utilizing fluorescence microscopy, HS-FITC, KC1-FITC, MY-FITC, and NC-FITC were incubated with raMoDCs labeled with the CD86-PE antibody. Green fluorescence signals were clearly observed in the HS-FITC, KC1-FITC, and MY-FITC incubated groups, while no fluorescence signal was detected in the NC-FITC incubated group ().
Figure 4. Binding ability of dc-targeting peptides to raMoDCs. (a) Fluorescence microscopy images showing the binding of FITC-labeled HS, KC1, MY, and negative control (NC) peptides to raMoDCs. (b) Flow cytometry analysis of the binding ability of the peptides to raMoDCs. Red indicates rabbit DCs expressing CD86, green represents FITC-labeled peptides, and blue represents nuclei stained with DAPI. Changes in brightness, contrast, or color balance were applied uniformly to all pixels in the microscopy image.
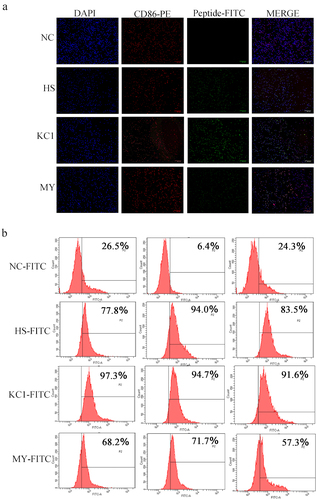
For precise quantitative analysis, flow cytometry was employed to assess the binding ability. The results revealed that the positivity rate of raMoDCs incubated with KC1-FITC was remarkably high at 91.6%, significantly surpassing those of the NC-FITC, HS-FITC, and MY-FITC groups (). These findings underscore the superior binding performance of the porcine DC-targeting peptide KC1 to raMoDCs, establishing it as the most effective among the tested DC-targeting peptides.
Construction and verification of recombinant Lactobacillus co-expressing RaMoDC-targeting peptides and RHDV VP60 antigen
To validate the enhancement of antigen binding to DCs through the fusion of DC-targeting peptides, recombinant Lactobacillus strains expressing DC-targeting peptides HS, KC1, and MY along with the RHDV protective antigen VP60 were constructed following the depicted strategy (). The expression of HS-VP60/L. reuteri, KC1-VP60/L. reuteri, MY-VP60/L. reuteri, and the vector/L. reuteri (negative control) was assessed using western blotting () and immunofluorescence analysis (IFA) ().
Figure 5. Characterization of expressed proteins in L. reuteri. (a) Schematic representation of the construction of recombinant plasmids: pPG-HS-VP60, pPG-KC1-VP60, pPG-MY-VP60, and pPG-VP60. (b) Verification of expressed VP60 protein in L. reuteri through western blotting. The primary antibody used was mouse anti-Flag monoclonal antibody. (c) Visualization of expressed proteins in Lactobacillus via fluorescence microscopy. The fluorescence signals confirm the presence of HS-VP60/L. reuteri, KC1-VP60/L. reuteri, and MY-VP60/L. reuteri.
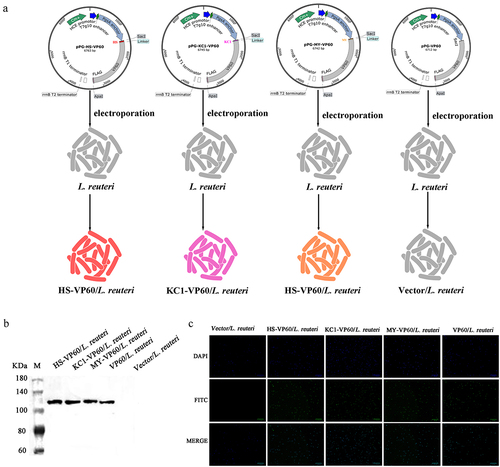
In , the bands corresponding to the expressed fusion proteins aligned with the theoretical size values, including the pgsA anchor (approximately 63 kD) and VP60 (approximately 60 kD). As expected, the vector/L. reuteri control did not exhibit immunoreactive bands. The IFA results () concurred with the western blotting data, as green fluorescence signals were distinctly observed in HS-VP60/L. reuteri, KC1-VP60/L. reuteri, and MY-VP60/L. reuteri. Thus, this confirms the successful construction of recombinant Lactobacillus co-expressing raMoDC-targeting peptides and the RHDV protective antigen VP60, denoted as HS-VP60/L. reuteri, KC1-VP60/L. reuteri, and MY-VP60/L. reuteri.
DC-targeting peptides enhance antigen recognition and capture efficiency of RaMoDCs
In assessing whether recombinant Lactobacillus expressing DC-targeting peptides were more effectively captured by raMoDCs, VP60/L. reuteri, HS-VP60/L. reuteri, KC1-VP60/L. reuteri, and MY-VP60/L. reuteri were incubated with raMoDCs. Scanning electron microscopy at various time points revealed the successful capture of all Lactobacillus strains by raMoDCs (). Toll-like receptors (TLRs) expressed by DCs play a crucial role in antigen presentation and immune responses. The qRT-PCR results indicated significantly higher levels of TLR-2, TNF-α, and IL-4 in raMoDCs stimulated by KC1-VP60/L. reuteri compared to other strains (p < 0.01), while TLR-4 expression did not markedly differ among the recombinant Lactobacillus strains (). These findings demonstrate that the DC-targeting peptides tested, particularly KC1, effectively enhance the capture and recognition of recombinant Lactobacillus by raMoDCs.
Figure 6. The ability of raMoDCs to recognize and capture recombinant Lactobacillus was evaluated using scanning electron microscopy and the analysis of toll-like receptor and cytokine mRNA levels in raMoDCs (106 cells/mL) in response to Vector/L. reuteri, VP60/L. reuteri, HS-VP60/L. reuteri, KC1-VP60/L. reuteri, MY-VP60/L. reuteri, and LPS (2 µg/mL) stimulation. (a) Scanning electron microscopy images showing the morphology of raMoDCs capturing recombinant Lactobacillus at 30, 60, 120 min. (b) Recombinant Lactobacillus-incubated raMoDCs at a ratio of 1:10 (DCs: recombinant Lactobacillus). RaMoDCs were stimulated by recombinant Lactobacillus and LPS for 12 h. Unstimulated raMoDCs were used as a control. Different letters (a vs. b, a vs. c, b vs. (c) indicate significant differences (p < 0.01) at the same time point.
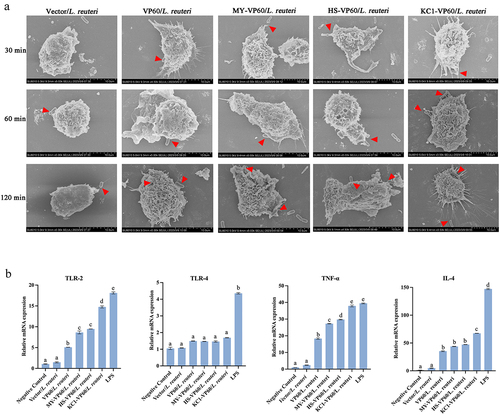
Induction of immune responses and protection against RHDV by oral administration of recombinant Lactobacillus in rabbits
The effectiveness of recombinant Lactobacillus in inducing immunogenicity and providing protection against RHDV challenge after oral immunization is illustrated in . Anti-RHDV-specific SIgA and IgG levels in all immunization groups were significantly elevated compared to the control group on the 14th and 21st days post-vaccination, respectively (p < 0.01) (). Notably, the levels of SIgA and IgG antibodies induced by KC1-VP60/L. reuteri were the highest among the immunization groups (). For the challenge experiment, rabbits orally administered KC1-VP60/L. reuteri were selected, while rabbits administered PBS were designated as the control group; after 14 days, RHDV-infected rabbits were cohabitated in the same cage (). The outcomes revealed that all rabbits in the control group succumbed at approximately 91–139 h, while immunized rabbits perished at approximately 147–223 h after cohabitation with infected rabbits (). RT-qPCR results indicated significantly lower RHDV copy numbers in the liver, spleen, lungs, and kidneys of immunized rabbits compared to the control group (). These findings suggest that recombinant Lactobacillus expressing KC1 induces robust mucosal and systemic immune responses, reducing RHDV copy numbers and extending the survival time of infected rabbits.
Figure 7. Detection of anti-RHDV-specific IgG/sIgA antibody levels and rabbit survival after challenge. (a) Specific anti-RHDV IgG and sIgA antibody levels in rabbits orally immunized with PBS, VP60/L. reuteri, HS-VP60/L. reuteri, KC1-VP60/L. reuteri, and MY-VP60/L. reuteri. Measurement of a specific anti-RHDV IgG antibody in the antisera from immunized rabbits by ELISA using RHDV as the coating antigen. Measurement of specific anti-RHDV SIgA antibody levels in the feces and nasal cavity by ELISA using RHDV as the coating antigen. (b) Rabbit survival after challenge. (c) RHDV load in the liver, spleen, lungs, and kidneys of infected dead rabbits. Different letters (a vs. b, a vs. c, b vs. c) indicate significant differences (p < 0.01) at the same time point.
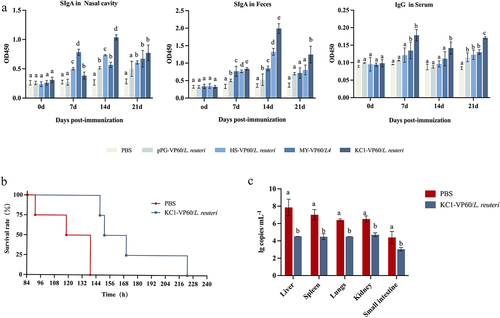
Discussion
The quest for safe, enduring, and potent vaccines remains a significant challenge in the field of immunization. In recent years, a novel strategy targeting antigens directly to DCs has emerged as a promising avenue for enhancing immune responses post-vaccination [Citation50–52]. Building upon our previous findings demonstrating that recombinant Lactobacillus expressing VP60 could elicit mucosal and systemic immune responses in rabbits [Citation21], this study aimed to elevate the immune response levels against RHDV by constructing another recombinant Lactobacillus strain co-expressing a DC-targeting peptide and VP60.
While DC-targeting peptides have been extensively employed in vaccines [Citation53–55], the lack of reports on rabbit-derived DC-targeting peptides prompted an investigation into their effectiveness. DC-targeting peptides from different species, including a rabbit DC-targeting peptide (HS), a porcine DC-targeting peptide (KC1) identified in our laboratory (unpublished), and a modified DC-targeting peptide (MY) based on the LYPPY motif of porcine CTLA4 [Citation47], were selected for analysis. The evaluation revealed that the DC-targeting peptide KC1 exhibited superior performance in targeting raMoDCs. This suggests that the source of DC-targeting peptides does not significantly impact their ability to target DCs.
Antigens are captured and processed by DCs before being presented to T cells. DCs gradually mature, with increased expression of surface molecules, and augmented cytokine secretion are characteristics of matured DCs [Citation56]. TLRs on the surface of DCs can also mediate their maturation and play an important role in initiating innate immune responses [Citation57]. Our study found an elevation in the expression of TLR-2, TNF-α, and IL-4 in raMoDCs following incubation with recombinant Lactobacillus expressing DC-targeting peptides, with KC1-VP60/L. reuteri demonstrating the highest performance. However, no significant difference was observed in TLR-4 expression in raMoDCs after incubation with different recombinant Lactobacillus expressing DC-targeting peptides. This discrepancy may be attributed to TLR-2 forming a heterodimer with TLR-1 or TLR-6, recognizing various microbial ligands, while TLR-4 primarily mediates the response to endotoxins/LPS [Citation58]. These findings underscore that the origin of DC-targeting peptides does not play a pivotal role in their ability to effectively target DCs.
An effective oral vaccine based on recombinant Lactobacillus has the potential to elicit both systemic and mucosal immune responses [Citation59,Citation60]. Therefore, we assessed the immune response efficacy in rabbits induced by VP60/L. reuteri, HS-VP60/L. reuteri, KC1-VP60/L. reuteri, and MY-VP60/L. reuteri through oral immunization. IgG and IgA are mainly involved in the systemic immune and mucosal immune of the body, respectively [Citation59,Citation61]. In this study, we detected that recombinant Lactobacillus expressing DC-targeting peptides could significantly increase the anti-RHDV-specific SIgA and IgG of rabbits after oral immunization, comparing the VP60/L. reuteri group, furthermore the KC1-VP60/L. reuteri performs best in the groups which expressing DC-targeting peptides. The results recommended that the KC1-VP60/L. reuteri expressing the VP60 of RHDV fused with KC1 could effectively induce immune responses, including systemic immune and mucosal immune responses. Consequently, KC1-VP60/L. reuteri was selected to evaluate its protective effects against the RHDV challenge. Contact with infected and dead rabbits is a very important direct route of RHDV transmission, so, cohabitied RHDV-infected rabbits with healthy rabbits is a good way to simulate natural infections [Citation62,Citation63]. The immunoprotective efficacy was assessed by cohabiting and raising RHDV-infected rabbits with immunized ones. The outcomes demonstrated that oral immunization with KC1-VP60/L. reuteri reduced RHDV copy numbers and prolonged the survival time of infected rabbits, albeit without complete prevention of rabbit death. In a previous study, rabbits that received oral immunization with recombinant Lactobacillus once a day for three consecutive days and were boosted twice at 2-week intervals were found to be completely protected against RHDV challenge [Citation21]. In the current study, rabbits were immunized with KC1-VP60/L. reuteri only once to assess the DC-targeting peptide’s efficacy in targeting DCs. It is essential to note that future studies should focus on refining optimal vaccination programs. The collective findings affirm that recombinant Lactobacillus expressing KC1 exhibited enhanced capture by rabbit DCs and promoted DC maturation more effectively. Additionally, the DC-targeting peptide KC1, when expressed by recombinant Lactobacillus, robustly induced both systemic and mucosal immune responses against RHDV. This study underscores the promising strategy of antigen targeting to DCs using Lactobacillus, providing a foundation for the potential development of rabbit vaccines against RHDV infection.
Author contributions
L.W. and Y.L. conceived and designed the study. T.X., X.L., D.K., and T.G. performed most of the experiments. Y.G., L.X., Y.J., X.W., Z.S., J.L., H.Z., W.C., X.Q., and L.T. participated in the discussion. T.X. supervised the work and revised the final version of the manuscript. All the authors have read and approved the final manuscript.
Supplemental Material
Download PDF (290.8 KB)Disclosure statement
No potential conflict of interest was reported by the author(s).
Data availability statement
The data that support the findings of this study are openly available in Mendeley Data at http://doi.org/10.17632/s9x7z3v6df.1
Supplemental material
Supplemental data for this article can be accessed online at https://doi.org/10.1080/21505594.2024.2368080
Additional information
Funding
References
- Marcato PS, Benazzi C, Vecchi G, et al. Clinical and pathological features of viral haemorrhagic disease of rabbits and the European brown hare syndrome. Rev Sci Tech. 1991;10(2):371–15. doi: 10.20506/rst.10.2.560
- Abrantes J, van der Loo W, Le Pendu J, et al. Rabbit haemorrhagic disease (RHD) and rabbit haemorrhagic disease virus (RHDV): a review. Vet Res. 2012;43(1):12. doi: 10.1186/1297-9716-43-12
- Oem JK, Lee KN, Roh IS, et al. Identification and characterization of rabbit hemorrhagic disease virus genetic variants isolated in Korea. J Vet Med Sci. 2009;71(11):1519–1523. doi: 10.1292/jvms.001519
- Parra F, Prieto M. Purification and characterization of a calicivirus as the causative agent of a lethal hemorrhagic disease in rabbits. J Virol. 1990;64(8):4013–4015. doi: 10.1128/jvi.64.8.4013-4015.1990
- Boga JA, Marín MS, Casais R, et al. In vitro translation of a subgenomic mRNA from purified virions of the Spanish field isolate AST/89 of rabbit hemorrhagic disease virus (RHDV). Virus Res. 1992;26(1):33–40. doi: 10.1016/0168-1702(92)90144-X
- Torres JM, Ramírez MA, Morales M, et al. Safety evaluation of a recombinant myxoma-RHDV virus inducing horizontal transmissible protection against myxomatosis and rabbit haemorrhagic disease. Vaccine. 2000;19(2–3):174–182. doi: 10.1016/S0264-410X(00)00183-3
- Zhu J, Miao Q, Tan Y, et al. Inclusion of an arg-Gly-asp receptor-recognition motif into the capsid protein of rabbit hemorrhagic disease virus enables culture of the virus in vitro. J Biol Chem. 2017;292(21):8605–8615. doi: 10.1074/jbc.M117.780924
- Argüello Villares JL. Viral haemorrhagic disease of rabbits: vaccination and immune response. Rev Sci Tech. 1991;10(2):459–480. doi: 10.20506/rst.10.2.554
- Boga JA, Casais R, Marin MS, et al. Molecular cloning, sequencing and expression in Escherichia coli of the capsid protein gene from rabbit haemorrhagic disease virus (Spanish isolate AST/89). J Gen Virol. 1994;75(Pt 9):2409–2413. doi: 10.1099/0022-1317-75-9-2409
- Boga JA, Martín Alonso JM, Casais R, et al. A single dose immunization with rabbit haemorrhagic disease virus major capsid protein produced in Saccharomyces cerevisiae induces protection. J Gen Virol. 1997;78(Pt 9):2315–2318. doi: 10.1099/0022-1317-78-9-2315
- Farnós O, Boué O, Parra F, et al. High-level expression and immunogenic properties of the recombinant rabbit hemorrhagic disease virus VP60 capsid protein obtained in Pichia pastoris. J Biotechnol. 2005;117(3):215–224. doi: 10.1016/j.jbiotec.2005.01.013
- Castanon S, Marin MS, Martin-Alonso JM, et al. Immunization with potato plants expressing VP60 protein protects against rabbit hemorrhagic disease virus. J Virol. 1999;73(5):4452–4455. doi: 10.1128/JVI.73.5.4452-4455.1999
- Fernández-Fernández MR, Mouriño M, Rivera J, et al. Protection of rabbits against rabbit hemorrhagic disease virus by immunization with the VP60 protein expressed in plants with a potyvirus-based vector. Virology. 2001;280(2):283–291. doi: 10.1006/viro.2000.0762
- Martín-Alonso JM, Castañón S, Alonso P, et al. Oral immunization using tuber extracts from transgenic potato plants expressing rabbit hemorrhagic disease virus capsid protein. Transgenic Res. 2003;12(1):127–130. doi: 10.1023/A:1022112717331
- Gil F, Titarenko E, Terrada E, et al. Successful oral prime-immunization with VP60 from rabbit haemorrhagic disease virus produced in transgenic plants using different fusion strategies. Plant Biotechnol J. 2006;4(1):135–143. doi: 10.1111/j.1467-7652.2005.00172.x
- Bertagnoli S, Gelfi J, Le Gall G, et al. Protection against myxomatosis and rabbit viral hemorrhagic disease with recombinant myxoma viruses expressing rabbit hemorrhagic disease virus capsid protein. J Virol. 1996;70(8):5061–5066. doi: 10.1128/jvi.70.8.5061-5066.1996
- Fischer L, Le Gros FX, Mason PW, et al. A recombinant canarypox virus protects rabbits against a lethal rabbit hemorrhagic disease virus (RHDV) challenge. Vaccine. 1997;15(1):90–96. doi: 10.1016/S0264-410X(96)00102-8
- Nagesha HS, Wang LF, Hyatt AD, et al. Self-assembly, antigenicity, and immunogenicity of the rabbit haemorrhagic disease virus (Czechoslovakian strain V-351) capsid protein expressed in baculovirus. Arch Virol. 1995;140(6):1095–1108. doi: 10.1007/BF01315418
- Plana-Duran J, Bastons M, Rodriguez MJ, et al. Oral immunization of rabbits with VP60 particles confers protection against rabbit hemorrhagic disease. Arch Virol. 1996;141(8):1423–1436. doi: 10.1007/BF01718245
- Gromadzka B, Szewczyk B, Konopa G, et al. Recombinant VP60 in the form of virion-like particles as a potential vaccine against rabbit hemorrhagic disease virus. Acta Biochim Pol. 2006;53(2):371–376. doi: 10.18388/abp.2006_3351
- Wang L, Xia T, Guo T, et al. Recombinant lactobacillus casei expressing capsid protein VP60 can serve as vaccine against rabbit hemorrhagic disease virus in rabbits. Vaccines (Basel). 2019;7(4):7. doi: 10.3390/vaccines7040172
- Pfeiler EA, Azcarate-Peril MA, Klaenhammer TR. Characterization of a novel bile-inducible operon encoding a two-component regulatory system in Lactobacillus acidophilus. J Bacteriol. 2007;189(13):4624–4634. doi: 10.1128/JB.00337-07
- Daniel C, Roussel Y, Kleerebezem M, et al. Recombinant lactic acid bacteria as mucosal biotherapeutic agents. Trends Biotechnol. 2011;29(10):499–508. doi: 10.1016/j.tibtech.2011.05.002
- Martín V, Maldonado A, Fernández L, et al. Inhibition of human immunodeficiency virus type 1 by lactic acid bacteria from human breastmilk. Breastfeed Med. 2010;5(4):153–158. doi: 10.1089/bfm.2010.0001
- Mahe MM, Aihara E, Schumacher MA, et al. Establishment of gastrointestinal epithelial organoids. Curr Protoc Mouse Biol. 2013;3(4):217–240. doi: 10.1002/9780470942390.mo130179
- Wu H, Xie S, Miao J, et al. Lactobacillus reuteri maintains intestinal epithelial regeneration and repairs damaged intestinal mucosa. Gut Microbes. 2020;11(4):997–1014. doi: 10.1080/19490976.2020.1734423
- Craig K, Dai X, Li A, et al. A lactic acid bacteria (LAB)-based vaccine Candidate for human norovirus. Viruses. 2019;11(3):11. doi: 10.3390/v11030213
- Eisenbarth SC. Dendritic cell subsets in T cell programming: location dictates function. Nat Rev Immunol. 2019;19(2):89–103. doi: 10.1038/s41577-018-0088-1
- Ma S, Qiao X, Xu Y, et al. Screening and identification of a chicken dendritic cell binding peptide by using a phage display library. Front Immunol. 2019;10:1853. doi: 10.3389/fimmu.2019.01853
- Shimizu T, Kawaguchi Y, Ando H, et al. Development of an antigen delivery system for a B cell-targeted vaccine as an alternative to dendritic cell-targeted vaccines. Chem Pharm Bull (Tokyo). 2022;70(5):341–350. doi: 10.1248/cpb.c22-00047
- Embgenbroich M, Burgdorf S. Current concepts of antigen cross-presentation. Front Immunol. 2018;9:1643. doi: 10.3389/fimmu.2018.01643
- Théry C, Amigorena S. The cell biology of antigen presentation in dendritic cells. Curr Opin Immunol. 2001;13(1):45–51. doi: 10.1016/S0952-7915(00)00180-1
- Banchereau J, Briere F, Caux C, et al. Immunobiology of dendritic cells. Annu Rev Immunol. 2000;18(1):767–811. doi: 10.1146/annurev.immunol.18.1.767
- Lin J, Wang H, Liu C, et al. Dendritic cells: versatile players in renal transplantation. Front Immunol. 2021;12:654540. doi: 10.3389/fimmu.2021.654540
- Deliyannis G, Boyle JS, Brady JL, et al. A fusion DNA vaccine that targets antigen-presenting cells increases protection from viral challenge. Proc Natl Acad Sci USA. 2000;97(12):6676–6680. doi: 10.1073/pnas.120162497
- Lu M, Isogawa M, Xu Y, et al. Immunization with the gene expressing woodchuck hepatitis virus nucleocapsid protein fused to cytotoxic-T-lymphocyte-associated antigen 4 leads to enhanced specific immune responses in mice and woodchucks. J Virol. 2005;79(10):6368–6376. doi: 10.1128/JVI.79.10.6368-6376.2005
- Rohrbach F, Weth R, Kursar M, et al. Targeted delivery of the ErbB2/HER2 tumor antigen to professional APCs results in effective antitumor immunity. J Immunol. 2005;174(9):5481–5489. doi: 10.4049/jimmunol.174.9.5481
- Gerstmayer B, Pessara U, Wels W. Construction and expression in the yeast pichia pastoris of functionally active soluble forms of the human costimulatory molecules B7-1 and B7-2 and the B7 counter-receptor CTLA-4. FEBS Lett. 1997;407(1):63–68. doi: 10.1016/S0014-5793(97)00294-9
- Owen JL, Sahay B, Mohamadzadeh M. New generation of oral mucosal vaccines targeting dendritic cells. Curr Opin Chem Biol. 2013;17(6):918–924. doi: 10.1016/j.cbpa.2013.06.013
- Mohamadzadeh M, Duong T, Sandwick SJ, et al. Dendritic cell targeting of Bacillus anthracis protective antigen expressed by Lactobacillus acidophilus protects mice from lethal challenge. Proc Natl Acad Sci U S A. 2009;106(11):4331–4336. doi: 10.1073/pnas.0900029106
- Chen W, Ma C, Wang D, et al. Immune response and protective efficacy of recombinant Enterococcus faecalis displaying dendritic cell–targeting peptide fused with Eimeria tenella 3-1E protein. Poult Sci. 2020;99(6):2967–2975. doi: 10.1016/j.psj.2020.03.014
- Hou X, Jiang X, Jiang Y, et al. Oral immunization against PEDV with recombinant lactobacillus casei expressing dendritic cell-targeting peptide fusing COE protein of PEDV in Piglets. Viruses. 2018;10(3):10. doi: 10.3390/v10030106
- Jin YB, Yang WT, Shi CW, et al. Immune responses induced by recombinant Lactobacillus plantarum expressing the spike protein derived from transmissible gastroenteritis virus in piglets. Appl Microbiol Biotechnol. 2018;102(19):8403–8417. doi: 10.1007/s00253-018-9205-0
- Ma S, Wang L, Huang X, et al. Oral recombinant lactobacillus vaccine targeting the intestinal microfold cells and dendritic cells for delivering the core neutralizing epitope of porcine epidemic diarrhea virus. Microb Cell Fact. 2018;17(1):20. doi: 10.1186/s12934-018-0861-7
- Xia T, Yang H, Guo Y, et al. Human dendritic cell targeting peptide can be targeted to porcine dendritic cells to improve antigen capture efficiency to stimulate stronger immune response. Front Immunol. 2022;13:950597. doi: 10.3389/fimmu.2022.950597
- Wang Y, Feng B, Niu C, et al. Dendritic cell targeting of bovine viral diarrhea virus E2 protein expressed by lactobacillus casei effectively induces antigen-specific immune responses via oral vaccination. Viruses. 2019;11(6):11. doi: 10.3390/v11060575
- Xia T, Wang N, Tang Y, et al. Delivery of antigen to porcine dendritic cells by fusing antigen with porcine dendritic cells targeting peptide. Front Immunol. 2022;13:926279. doi: 10.3389/fimmu.2022.926279
- Percie du Sert N, Hurst V, Ahluwalia A, et al. The ARRIVE guidelines 2.0: Updated guidelines for reporting animal research. Br J Pharmacol. 2020;177:3617–3624. doi: 10.1111/bph.15193
- Guo T, Gao C, Hao J, et al. Strategy of developing oral vaccine candidates against co-infection of porcine diarrhea viruses based on a Lactobacillus delivery system. Front Microbiol. 2022;13:872550. doi: 10.3389/fmicb.2022.872550
- Reddy ST, Swartz MA, Hubbell JA. Targeting dendritic cells with biomaterials: developing the next generation of vaccines. Trends Immunol. 2006;27(12):573–579. doi: 10.1016/j.it.2006.10.005
- Macri C, Jenika D, Ouslinis C, et al. Targeting dendritic cells to advance cross-presentation and vaccination outcomes. Semin Immunol. 2023;68:101762. doi: 10.1016/j.smim.2023.101762
- Caminschi I, Lahoud MH, Shortman K. Enhancing immune responses by targeting antigen to DC. Eur J Immunol. 2009;39(4):931–938. doi: 10.1002/eji.200839035
- Wang Z, He Y, Wang W, et al. A novel “prime and pull” strategy mediated by the combination of two dendritic cell-targeting designs induced protective lung tissue-resident memory T cells against H1N1 influenza virus challenge. J Nanobiotechnology. 2023;21(1):479. doi: 10.1186/s12951-023-02229-y
- Jia F, Sun C, Ge C, et al. Chicken dendritic cell-targeting nanobodies mediated improved protective effects against H9N2 influenza virus challenge in a homologous sequential immunization study. Vet Microbiol. 2023;285:109875. doi: 10.1016/j.vetmic.2023.109875
- Kim SH, Shim EH, Kim DJ, et al. C5aR+ dendritic cells fine-tune the Peyer’s patch microenvironment to induce antigen-specific CD8+ T cells. NPJ Vaccines. 2023;8(1):120. doi: 10.1038/s41541-023-00720-z
- Liu X, Xia X, Wang X, et al. Tropomodulin1 expression increases upon maturation in dendritic cells and promotes their maturation and immune functions. Front Immunol. 2020;11:587441. doi: 10.3389/fimmu.2020.587441
- Ullah MO, Sweet MJ, Mansell A, et al. TRIF-dependent TLR signaling, its functions in host defense and inflammation, and its potential as a therapeutic target. J Leukocyte Biol. 2016;100(1):27–45. doi: 10.1189/jlb.2RI1115-531R
- Noreen M, Arshad M. Association of TLR1, TLR2, TLR4, TLR6, and TIRAP polymorphisms with disease susceptibility. Immunol Res. 2015;62(2):234–252. doi: 10.1007/s12026-015-8640-6
- Holmgren J, Czerkinsky C. Mucosal immunity and vaccines. Nat Med. 2005;11(S4):S45–53. doi: 10.1038/nm1213
- Mantis NJ, Rol N, Corthésy B. Secretory IgA’s complex roles in immunity and mucosal homeostasis in the gut. Mucosal Immunol. 2011;4(6):603–611. doi: 10.1038/mi.2011.41
- Kurashima Y, Kiyono H. Mucosal ecological network of epithelium and immune cells for gut homeostasis and tissue healing. Annu Rev Immunol. 2017;35(1):119–147. doi: 10.1146/annurev-immunol-051116-052424
- Perera KD, Johnson D, Lovell S, et al. Potent protease inhibitors of highly pathogenic lagoviruses: rabbit hemorrhagic disease virus and European brown hare syndrome virus. Microbiol Spectr. 2022;10(4):e0014222. doi: 10.1128/spectrum.00142-22
- Merchán T, Rocha G, Alda F, et al. Detection of rabbit haemorrhagic disease virus (RHDV) in nonspecific vertebrate hosts sympatric to the European wild rabbit (Oryctolagus cuniculus). Infect Genet Evol. 2011;11(6):1469–1474. doi: 10.1016/j.meegid.2011.05.001