ABSTRACT
Interaction between cancerous, non-transformed cells, and non-cellular components within the tumor microenvironment plays a key role in response to treatment. However, short-term culture or xenotransplantation of cancer specimens in immunodeficient animals results in dramatic modifications of the tumor microenvironment, thus preventing reliable assessment of compounds or biologicals of potential therapeutic relevance.
We used a perfusion-based bioreactor developed for tissue engineering purposes to successfully maintain the tumor microenvironment of freshly excised breast cancer tissue obtained from 27 breast cancer patients and used this platform to test the therapeutic effect of antiestrogens as well as checkpoint-inhibitors on the cancer cells.
Viability and functions of tumor and immune cells could be maintained for over 2 weeks in perfused bioreactors. Next generation sequencing authenticated cultured tissue specimens as closely matching the original clinical samples. Anti-estrogen treatment of cultured estrogen receptor positive breast cancer tissue as well as administration of pertuzumab to a Her2 positive breast cancer both had an anti-proliferative effect. Treatment with anti-programmed-death-Ligand (PD-L)-1 and anti-cytotoxic T lymphocyte-associated protein (CTLA)-4 antibodies lead to immune activation, evidenced by increased lymphocyte proliferation, increased expression of IFNγ, and decreased expression of IL10, accompanied by a massive cancer cell death in ex vivo triple negative breast cancer specimens.
In the era of personalized medicine, the ex vivo culture of breast cancer tissue represents a promising approach for the pre-clinical evaluation of conventional and immune-mediated treatments and provides a platform for testing of innovative treatments.
Introduction
Breast cancer is one of the most frequent cancer types and a leading cause of cancer-related death in women, and its incidence is increasing worldwide.Citation1 While for particular subtypes of breast cancer, targeted therapies such as endocrine blockade therapies and anti-HER2 treatment are effective, there are currently no targeted therapies for triple negative breast cancers (TNBC), representing the most aggressive type of breast cancer.Citation2 Therefore, especially for this cancer subtype, the translation of research findings into new, clinically effective therapies is crucial and requires innovative culture models reliably mirroring the complex biology of breast cancer.
Preliminary clinical studies suggest that a subpopulation of TNBC patients may profit from anti-PD-1 or anti-PD-L1 treatment,Citation3-5 however, more than 80% of these patients are currently treated unnecessarily with a potentially toxic antibody. The possibility of testing the effectiveness of these checkpoint inhibitors on patients' own tumor tissues could help in the selection of responsive patients.
Cancer research and development of treatment protocols are traditionally based on the use of established tumor cell lines and animal models for in vitro and in vivo assays. These approaches have led to a large number of clinically significant achievements.Citation6 However, limitations and disadvantages have also emerged. Rapid growth and minimal needs of care of established cancer cell lines are obviously advantageous. However, their in vitro behavior might fail to mirror in vivo features, since selective pressure only allows thriving of the most aggressive cells, resulting in loss of cancer heterogeneity.Citation6,7 In vivo testing of therapeutic compounds is also performed on patient-derived tumor xenografts (PDTX), based on direct implantation of human cancers in immunodeficient mice.Citation2 While this system certainly better reflects cancer heterogeneity and apparently retains gene expression profiles of the original tumor,Citation8 it is still hampered by several limitations. In particular, PDTX models fail to capture the human tumor microenvironment (TME) including the interactions between cancer and immune system, and cancer with the surrounding extracellular matrix which are increasingly being recognized as fundamental for cancer progression and metastasis formation,Citation7,9-11 and for the response of cancer cells to treatment.Citation12
Bioreactor technology has been used for more than a decade in the field of musculoskeletal tissue regenerationCitation11,13-17 and has led to the generation of autologous cartilage tissues which may be used for tissue reconstruction.Citation14,18 Early studies investigating the use of bioreactor systems in the field of 3D cancer models have shown encouraging results.Citation19-22 However, the potential of these systems for maintenance and culture of primary cancer tissue samples preserving the TME has not been explored so far.
In our study, we show that viability and functions of breast cancer cells and infiltrating immune cells can be maintained for over 2 weeks in perfusion-based bioreactors, thus allowing the in vitro assessment of the effectiveness of immune-therapeutic compounds such as checkpoint-inhibitors. This model represents a promising approach for the evaluation of immune-mediated treatments in a personalized fashion immediately after surgery, thus allowing selection of the most effective treatment of each individual patient in a timely fashion, while sparing unnecessary toxicity to the others.
Results
Establishment of a primary perfused culture of breast cancer specimens
Previous studies have shown that freshly excised tumor tissues from colorectal cancer and head and neck squamous cell carcinoma may be successfully maintained in static cultures for up to 72 h.Citation23 We used a perfusion-based bioreactor developed for tissue engineering purposesCitation19 to investigate the possibility of prolonged culture of surgically excised breast cancer tissues. In initial studies, to assess the role of perfusion, we comparatively analyzed tumor cell viability in static and perfused cultures from the same surgical specimens. After 1 and 2 weeks, tumor fragments cultured in static conditions were characterized by significantly lower percentages of viable tumor cells (vtc), as compared with their perfused counterparts ( and ).
Figure 1. Comparative analysis of breast cancer tissue specimens upon culture in static conditions or under perfusion. (A) Fragments from a representative surgically excised breast cancer specimen were cultured for the indicated time points in static or perfused culture conditions. Samples were then fixed, paraffin embedded, and sections were HE stained (Magnification 100× (Static) and 200× (Perfusion)). (B) Comparative analysis of percentages of vtc in fragments from at least seven different breast cancer surgical specimens cultured in static or perfused conditions for the indicated time points (*p ≤ 0.05; **p ≤ 0.01).
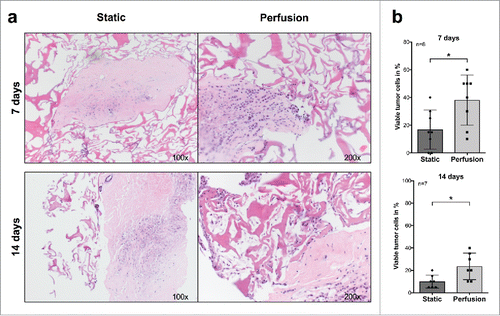
Prompted by these results, we cultured 30 freshly excised fragments from breast cancer specimens. Clinical-pathological parameters related to these tumors are reported in . In 27 cases, tumor tissue culture could be successfully performed in perfusion-based bioreactors. In two cases, there was no cancer in the cultured tissue fragments, and in another one, the culture got contaminated, so that the experiment had to be aborted. High numbers of viable cancer cells and infiltrating lymphocytes were detectable on day 7 cultures, with marked reductions on days 14 and 21 ( and ). Interestingly, following 7 d culture in perfused conditions, we also observed an infiltration of vtc and lymphocytes from the tumor fragment into the collagen scaffold, reaching its maximum after 14 d and declining thereafter ( and ). CK22 immunohistochemical staining confirmed the epithelial nature of cultured cancer cells and the infiltration by immune cells including CD68+ macrophages, CD3+ T, and CD20+ B lymphocytes ( and ). Remarkably, evidence of proliferation of cancer and stromal cells, as indicated by Ki-67 staining could also be observed (). In estrogen receptor (ER) positive cases (), ER expression was maintained up to 14 d in the expanding cancer tissue. Metastatic breast cancer tissue from two lymph node metastases and one brain metastasis could also be successfully cultured (Fig. S2).
Table 1. Clinico-pathological parameters of all included breast cancer patients.
Figure 2. Culture of breast cancer fragments in perfused bioreactors: preservation of TME cellular components. (A) Fragments from two representative surgically excised breast cancer specimens were inserted between collagen type I scaffolds and cultured in perfused bioreactors for the indicated time. Samples were then collected, fixed, and paraffin embedded. HE-stained sections from the original surgical specimens, cultured fragments and infiltrated scaffolds were then comparatively evaluated (Magnification 100× or 200× as indicated). (B) Quantitative analysis of tumor cell and lymphocyte numbers within cultured tumor fragments and collagen scaffolds at the indicated time points. Displayed results summarize data from 27 different breast cancer specimens. In one cultured specimen, the initial tissue fragment did not contain cancer, and in two additional specimens, counting of tumor cells and lymphocytes could not be performed due to lack of material, and thus these specimens were omitted for data analysis. Non-parametric Mann–Whitney test (****p ≤ 0.0001).
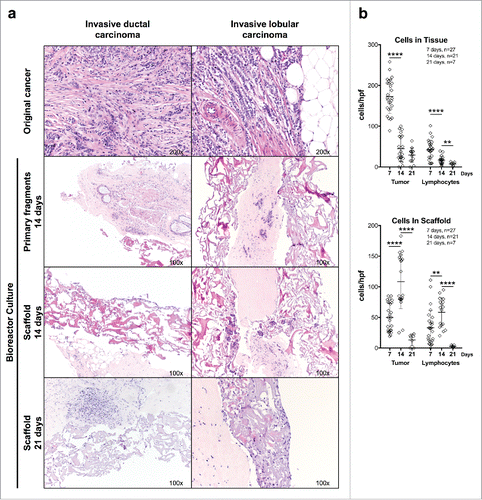
Figure 3. Immunohistochemical evaluation of breast cancer tissue fragments cultured in perfused bioreactors. (A) Fragments from the indicated representative surgically excised breast cancer specimen were cultured for 14 d in a perfused bioreactor. Samples were then collected fixed and paraffin embedded. Sections were stained with serological reagents recognizing the indicated markers (Magnification 200×). (B) Quantitative analysis of numbers of cells expressing the indicated markers in fragments from one breast cancer sample cultured for the indicated time points. The low lymphocyte count in the treated sample on day 7 is most probably due to tumor heterogeneity.
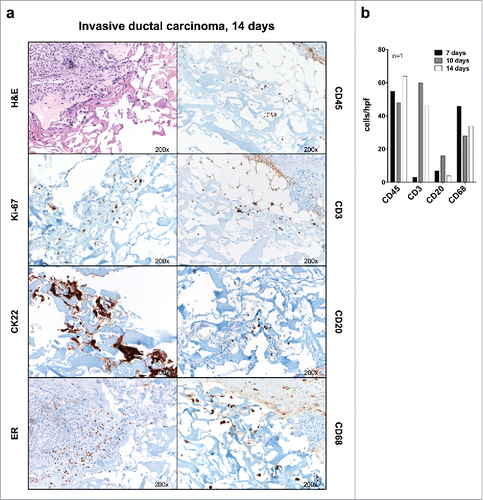
Authentication of cultured breast cancer tissue specimens by next generation sequencing (NGS) and phosphoproteome analysis
To evaluate the level of genetic identity between the primary tumor and the matching breast cancers growing into the scaffolds after 14 d of culture, we performed targeted-NGS on both types of specimens from two different TNBC patients. None of the tested samples displayed any known BRCA1 or BRCA2 pathological variants. A comprehensive list of BRCA1 and BRCA2 missense mutations detected in the analyzed samples is reported in Table S2. Of note, BRCA1 and BRCA2 mutation profiles were mostly overlapping in the first matched samples (F722), with only a few minor (n = 3 and n = 8 single nucleotide variation (SNV) in BRCA1/2, in F722TU and Bio-F722TU, respectively) private mutations (Table S2). The second matched pair of samples (F724) displayed an identical mutational profile (Table S2).
Subsequently, using the AmpliSeq™ Cancer Hotspot Panel v2 panel, selected regions of the 50 most frequently mutated genes in cancer were sequenced. At first, we profiled all the sequenced single nucleotide polymorphisms (SNPs) covered by this panel and compared them in matched samples. This analysis revealed that 95% of tested SNPs (21 out of 22 SNPs) were identical in both matched samples. A few private missense mutations were present in F722 original and F722 cultured specimen (n = 1 and n = 7 all unknown SNV, respectively), and one non-pathogenic mutation was identical in the two specimens. In contrast, no missense mutations were detectable in the F724 original and n = 2 (unknown) in the F724 cultured specimen (Table S2). Importantly, none of the reported mutations is known to be pathogenic. Thus, based on a targeted NGS approach, these results suggest that overall no key alterations in cancer driving genes occurred in cancer cells while growing into the perfused bioreactor.
To further reinforce the authentication of cultured tissue specimens by assessing the retention of the phosphorylated status of distinct proteins, we stained the original cancer and the matched cultured tissues (after 7, 14, and 21 d of culture) of two specimens with antibodies against phosphorylated (p)-STAT 1 and pSTAT 3 and phosphohistone 3. Remarkably, we did not observe any change in expression for pSTAT 1 and phosphohistone 3, whereas the expression of pSTAT 3 was slightly decreased in the cultured breast cancer tissue after 21 d, while still being maintained (Fig. S3). Taken together, these data authenticate tissue specimens grown in culture as closely matching the original clinical samples.
Anti-proliferative effect of anti-estrogen treatment on cultured estrogen receptor (ER)+ breast cancer tissue
To evaluate the possibility of in vitro testing of drug effectiveness on cultured breast cancer tissues, we added the ER-antagonist Fulvestrant (100 nM) to cultured ER+ breast cancer samples (n = 6). Exposure to Fulvestrant led to a significantly decreased number of vtc/hpf of both normal and malignant epithelial cells after 21 d, as compared with untreated cultures of the identical breast cancers (). In contrast, the number of lymphocytes/hpf in the tissue remained stable (data not shown), thus suggesting that the loss of epithelial cells was specifically due to the inhibitory activity of Fulvestrant.
Figure 4. Effects of anti-estrogen treatment on ER+ breast cancer fragments cultured in perfused bioreactors. (A) Fragments from a representative ER+ surgically excised breast cancer were cultured for 21 d in the presence or absence of Fulvestrant (100 nM). Samples were then fixed and paraffin embedded, sections were stained with HE (upper 2 and central 2 panels, magnification 100× and 200×, respectively) or immunohistochemically stained by using a CK22 specific reagent (lower two panels) (Magnification 200×). (B) Cumulative analysis of vtc per high-power field in fragments from six different ER+ breast cancers following culture in perfused conditions in the presence or absence of Fulvestrant. Data represented as ratio compared with day 0 (*p ≤ 0.05).
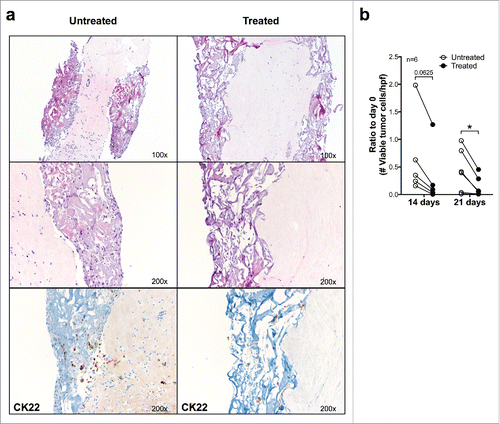
Treatment with phytohaemagglutinin (PHA) leads to increased numbers of T cells in cultured breast cancer specimens
To verify the possibility of actively conditioning the TME in vitro, and to evaluate the responsiveness of tumor-infiltrating lymphocytes (TILs), we added PHA (1 µg/mL) to cultured breast cancer samples (n = 4). Significantly increased numbers of T lymphocytes could be found in the scaffolds (p = 0.0445) and a corresponding trend could also be observed in the cultured primary fragments and by CD3-specific staining (Fig. S4) upon 7 d PHA stimulation, as compared with untreated samples. Taken together, these data suggest that immune competent cells within breast cancer TME may be successfully activated during culture in bioreactors.
Treatment with Pertuzumab in a Her2+ breast cancer specimen leads to reduced numbers of viable tumor cells
To evaluate the possibility of in vitro testing of targeted drug effectiveness on cultured breast cancer tissues, we added the monoclonal antibody Pertuzumab (255 µg/mL equal to CMax) to a Her2+ breast cancer specimen (confirmed by Her2 IHC and FISH). Administration of Pertuzumab for 7 d reduced the number of viable tumor cells from 55 to 11 (vtc/hpf). In contrast, the number of lymphocytes/hpf in the tissue increased over the same period of time from 18 lymphocytes/hpf to 190 lymphocytes/hpf after treatment of 7 d, thus suggesting that the loss of epithelial cells was specifically due to the inhibitory activity of Pertuzumab. Thus, we could show that the bioreactor systems can also be used to assess the effectiveness of targeted anti-Her2 therapy in Her2+ breast cancer specimens.
Treatment with anti-programmed-death-ligand (PD-L)-1 and anti-cytotoxic T lymphocyte-associated protein (CTLA)-4 antibodies leads to increased cancer cell death in TNBC specimens
Urged by the increasing clinical relevance of therapeutic administration of monoclonal antibodies (mAb) targeting “immunological checkpoints,” we evaluated the impact of treatment with anti-PD-L1 and anti-CTLA-4 therapeutic antibodies on cultured breast cancer tissues from eight patients.
Three patients had TNBC with Ki-67 positivity ranging between 25% and 90% of tumor cells (data not shown). In the first patient, cancer cells were strongly positive for PD-L1, with abundant PD-1+ tumor infiltrating lymphocytes (TILs). In the second patient, cancer cells were moderately positive for PD-L1, with abundant PD-1+ lymphocytes, while in the third patient, cancer cells were negative for PD-L1, but abundant numbers of PD-L1+ and PD-1+ lymphocytes were detectable (Figs. S5A–C).
Treatment with anti-PD-L1 or anti-CTLA-4 for 4–7 d induced dramatic decreases of tumor cell numbers in cultured specimens. Indeed, after treatment with anti-PD-L1 for 7 d, no vtc were left in tissue fragments of the first two patients, while the numbers of lymphocytes remained stable, and adjacent normal breast tissue was unaffected (). In the tissue of the third patient, treatment with anti-PD-L1 for 7 d reduced number of viable cancer cells from 57/hpf to 3 and 7/hpf, respectively, and no infiltration into the scaffold could be detected, while lymphocyte numbers remained stable or increased. summarizes data from the three independent experiments with different surgical specimens. Treatment with anti-CTLA-4 showed similar effects, although less pronounced ().
Figure 5. Culture of breast cancers in the presence of therapeutic mAb recognizing immunological checkpoints. (A) Fragments from a representative, untreated TNB cancer were cultured for the indicated time points in perfused bioreactors in the presence or absence of anti-PD-L1 therapeutic mAb (10 μg/mL). Samples were then fixed and paraffin embedded. Sections were stained with HE- and anti CK22-specific reagents (Magnification 100×). (B) Fragments from three different untreated TNB cancers were cultured in perfused bioreactors in the presence or absence of anti PD-L1 or anti CTLA-4 therapeutic mAbs (10 μg/mL) for the indicated time points. Numbers of vtc and lymphocytes in cultured tissue were then comparatively evaluated. Two-way ANOVA, matched values with Dunnett's multiple comparison test (*p ≤ 0.05; **p ≤ 0.01; ***p ≤ 0.001; ****p ≤ 0.0001). (C) Triplicate fragments of a luminal A breast cancer were treated for 7 d in perfused bioreactors, in the presence of the indicated therapeutic mAbs. Total cellular RNA was then extracted and reverse transcribed. The expression of the indicated genes was evaluated by qRT-PCR. Data are reported as fold increases or decreases, as compared with the expression of the same genes in untreated samples. IFNγ/IL-10 gene expression ratios are also shown.
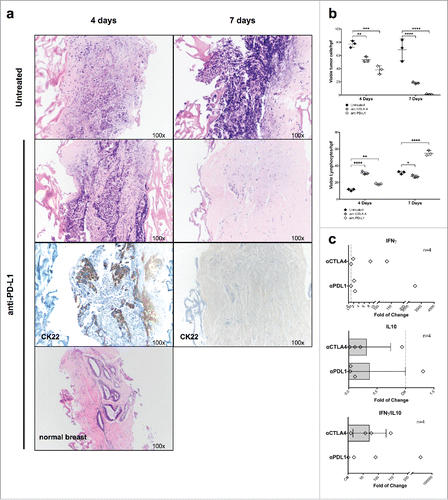
Additionally, we treated 4 Luminal A breast cancer subtype specimens (ER+, PR+, HER2−, Ki-67 <20%), one Luminal B (ER+, PR+, HER2−, Ki-67 25%), and one Her2 subtype (ER−, PR−, HER2+, Ki-67 60%) with checkpoint inhibitors. In one of the Luminal A specimens, most of the cultured primary tissue fragments (8 out of 13) did not contain cancer cells, since the macroscopically visible and sampled area contained a large fibrotic region and did not correspond with tumor size. Specifically, all the control samples (n = 3) did not contain cancer cells, so that we could not evaluate the effect of the checkpoint inhibitors on the tumor cells. Thus, we decided to not include this sample into the analysis. One of the Luminal A type specimens, the Luminal B specimen, and the Her 2 specimen showed complete absence of PD-L1 staining in the tumor cells, and only a few PD-L1 and PD-1 positive TILs. Interestingly, in the PD-L1 negative Luminal A tumor sample, treatment with anti-CTLA-4 and anti-PD-L1 did not show any effect on tumor cell count (95 vtc/hpf in the control versus 234 and 200 vtc/hpf after treatment with anti-CLTA-4 and anti-PD-L1, respectively), while in the PD-L1 negative Luminal B sample, there was a clear effect of anti-CTLA-4 and anti-PD-L1 treatment (100 vtc/hpf in the control vs. 60 and 24 vtc/hpf after treatment with anti-CLTA-4 and anti-PD-L1, respectively), and in the Her2 specimen, administration of anti-CTLA-4 for 7 d led to a total absence of viable tumor cells (55 vs. 0 vtc/hpf), whereas administration of anti-PD-L1 did not have any effect (55 vs. 58 vtc/hpf).
In the two remaining Luminal A specimens, tumor cells showed a clear albeit faintly positive IHC staining for PD-L1, with slightly stronger PD-L1 positivity in TILs as well as PD-1 positive TILs (Fig. S6). In one of these tumors, administration of anti-CTLA-4 or anti-PD-L1 for 7 d led to a marked decrease of viable tumor cells (60 vtc/hpf in the control vs. 22 and 15 vtc/hpf after treatment with anti-CLTA-4 and anti-PD-L1, respectively [average cell count of three samples per treatment]). In the last Luminal A specimen, individual treatment with anti-CLTA-4 and anti-PD-L1 did not decrease viable tumor cell count, whereas combined administration of anti-CTLA-4 and anti-PD-L1 antibodies for 7 d led to a marked decrease in numbers of viable tumor cells as compared with untreated tissue (155 vs. 28 vtc/hpf respectively), and no cancer cell infiltration into the scaffold was detectable (Fig. S7). Moreover, compared with untreated samples, we observed a reduction of FoxP3 positive cells in four out of five cases treated with anti-CTLA-4 (data not shown). This was, however, not statistically significant. IgG control antibody was tested in two cases and did not show any difference with the untreated matched samples (data not shown).
Gene signatures in breast cancer specimens following treatment with therapeutic mAbs
To obtain insights into the nature of immune-responses detectable in breast cancer samples upon treatment with therapeutic mAbs, we addressed the expression of genes associated with immune cell activation or inhibition following 7 d treatment with anti-PDL-1 or anti-CTLA-4 (see above) of three fragments from a luminal A breast cancers as well as one fragment of TNBC by qRT-PCR (). We observed an increase of IFNγ gene expression after treatment with anti-PDL-1 and anti-CTLA-4 (1.9-fold and 36.11-fold, respectively), and a decreased expression of IL10 gene after treatment with anti-PD-L1 (2.7-fold), and correspondingly also after treatment with anti-CTLA-4 (3.14-fold). Moreover, the pro- and anti-inflammatory IFNγ: IL-10 cytokine gene ratio suggested that both treatments were indeed favoring local immune-activation (37.2 and 71.2, respectively, for anti-PD-L1 and anti-CTL-4) (). By calculating the fold of change, two outliers for anti-PD-L1 were excluded.
Discussion
The interaction between cancerous and non-transformed cells within the TME plays a critical role in cancer biology and response to treatment.Citation23-25 However, the development of in vitro systems effectively mirroring the complexity of the TME and allowing its functional analysis in controlled conditions is highly challenging. By capitalizing on advances in tissue engineering,Citation20-22 we have used a perfusion-based bioreactor to culture entire fragments of breast cancer tissue for up to 3 weeks, preserving tumor cells and the autologous TME in terms of cellular composition and interaction.
Our results show that perfusion-based culture overcomes a major limitation of static culture: limited mass transportation, resulting in reduced and uncontrolled diffusion of oxygen and nutrients inside the tissue, compromising tissue growth and cellular viability.Citation14,26 The perfusion flow pushes the culture media through porous scaffolds, providing more nutrients and oxygen to the tissue, which results in an increased survival of all cells comprised in the TME, including tumor cells, lymphocytes, and additional stromal cells.Citation13,14,19,27 Importantly, during culture, breast cancer specimens appear to maintain the genetic profile of the original tissue. Indeed, NGS did not reveal major variations between original and cultured samples. These results are particularly encouraging in view of a possible use of this technology for drug testing, since they indicate that the cultured tissue is sufficiently genetically stable to extents allowing testing of therapeutic substances in a personalized treatment approach. Nevertheless, single nucleotide variations were observed. These results are not surprising. Recently, it has been demonstrated that intra-tumor genetic heterogeneity is present in almost all breast cancer lesions.Citation28 In fact, while tumors contain clonal genetic aberrations, inevitably, subclones harboring distinct mutations do exist.Citation29-32 Of note, the F724-matched samples, which presented with an identical BRCA1 and BRCA2 mutation profile, also displayed a lower degree of heterogeneity for the tested genes. Conversely, the F722-matched samples, characterized by the presence of some private variations for BRCA1 and BRCA2, also exhibited a few additional private missense mutations in the tested genes, consistent with a higher level of preexisting heterogeneity. Furthermore, the maintained phosphorylation status of STAT 1, STAT 3, and phosphohistone 3 suggests that culture of cancer tissue in the bioreactor does not alter the phosphorylation status and thus the function of at least these selected proteins involved in cell signaling.
The possibility of culturing entire tumor fragments in controlled conditions provides unique opportunities for the assessment of the effects of defined treatment in the context of a relatively intact TME. To support this notion, we first treated breast cancer tissues with anti-estrogens, supposedly targeting only ER+ breast cancer cells. Indeed, treatment with Fulvestrant resulted in decreased viability of cancer cells and reduced infiltration of viable cancer cells into collagen scaffolds. We then also treated a Her2+ breast cancer specimen with Pertuzumab (Perjeta®), a monoclonal antibody which blocks the dimerization of the Her2-receptor,Citation33 and could see a clear reduction of viable tumor cells after treatment of 7 d in the bioreactor. In sharp contrast, as expected, in both treatment conditions, interstitial cells were completely unaffected.
Major advances in cancer immunotherapy underline the need of innovative in vitro models recapitulating the complex interactions between cancer cells and the immune microenvironment.Citation26 While PDTX may allow in a fraction of cases the expansion of tumor cells, it does not allow pre-clinical evaluation of biologicals of potential use for cancer immunotherapy,Citation8 since human immune cells are rapidly replaced by murine interstitial cells. Therapeutic mAbs recognizing “immunological checkpoints” are increasingly being used for the treatment of advanced malignancies, based on the rationale of releasing the brakes limiting the effectiveness of endogenous antitumor immune response. However, response to treatment is typically observed in a minority of patients bearing tumors of diverse histological origin,Citation34,35 and adverse effects, possibly due to the activation of endogenous auto-reactive responses are frequently observed.Citation34 Therefore, the possibility of testing the effectiveness of these checkpoint inhibitors on patients' own tumor tissues could help in the selection of potentially responsive patients, while sparing unnecessary toxicity to the others. A prerequisite for these studies may be represented by the evaluation of the functional abilities of TILs as directly assessed within TME. With this in mind, we first tested responsiveness of TILs in breast cancer specimens to mitogens. Treatment with PHA increased the number of lymphocytes in primary tissues as well as in scaffolds, proving that the cultured lymphocytes are functionally competent and proliferating, and may be influenced by addition of specific stimuli.
Since the use of checkpoint inhibitors has been proposed in TNBC,Citation3,4,36 we investigated their in vitro effectiveness in cultured TNBC tissues from three previously untreated patients. In all of them, treatment with anti-PD-L1 or anti-CTLA-4 was successful with marked reduction in number or absence of viable cancer cells after 7 d, whereas lymphocytes and normal breast tissue remained viable. Interestingly, cancer cells from one patient were strongly positive for PD-L1, which has been suggested as a predictive biomarker to select patients for anti PD-1/PD-L1 therapies,Citation34 while the cancer cells of the other two patients showed only focal or no PD-L1 positivity, respectively. Additionally, the three completely negative breast cancer samples of the Luminal and Her2 subtype showed diverse response to treatment with anti-PD-L1, with two of the specimens showing no effect, while the third showed a clear effect. This could be an indication that expression of PD-L1 by breast cancer cells might not be an accurate predictive marker for response to anti-PD-L1 therapy. Preliminary results of a phase 1a trial using an anti-PD-L1 antibody (MPDL3280A) in patients with PD-L1 positive, metastatic TNBC show objective responses in 24% and complete response in 10% of patients (n = 21).Citation3 It is tempting to speculate that the innovative bioreactor-based culture technology described here could be useful in the selection of patients who potentially benefit from these treatments. Additionally, we analyzed three of the treated specimens (one Luminal A and two TNBCs) for expression of pSTAT 1, and could observe a consistent upregulation of pSTAT1 on tumor cells under treatment with anti-PD-L1 for 7 d, compared with the untreated control (Fig. S8). This finding indicates that blocking of PD-L1 induces upregulation of pSTA1 on the tumor cells via the T cells and proves that we are able to conserve a reactive and functioning inflammatory TME in the bioreactor.
In conclusion, the perfusion-based bioreactor presented here allows for immediate testing of different treatments in a personalized approach, providing rapid evaluation of their effectiveness on individual patient's cancer tissues within 1–3 weeks from initial surgery. Furthermore, it might represent a powerful tool for the study of basic aspects of cancer biology and immunobiology, for the pre-clinical evaluation of therapeutic compounds and for the selection of patients eligible for clinical trials. Importantly, this system might also allow for animal-free assessment of the effectiveness of innovative treatments, thus potentially reducing the burden of animal use in cancer research.Citation37
Materials and methods
Breast tissue fragment preparation
Informed written consent from all patients to provide anonymized breast cancer tissue for research uses, as approved by the responsible Ethical Commission (EKNZ proposal Nr. 2014–046 and 2016–00067) was obtained at the University Hospital of Basel. All breast cancer patients were older than 18 y and treated in the breast cancer center at the University Hospital Basel. For patients with language barriers, a translator was provided. Patients were informed about their right to receive upon request the data created with their sample as well as to have their tissue samples eliminated. Patients acknowledged that they cannot be identified via the published results and that they will be fully anonymized by giving each sample an ID study number. Immediately after surgery tissue specimens were transferred to the Institute of Pathology, where they were anatomically orientated, inked, and then cut open. Small tissue specimens, measuring up to 1 × 1 cm, were removed from the macroscopically identifiable cancer, placed in isotonic NaCl solution and taken to the laboratory. Upon arrival at the laboratory, tumor tissue was rinsed twice in cold PBS and then chopped using a tissue chopper device (McIlwain Tissue Chopper; Ted Pella Inc.) to obtain 2 × 2 × 2 mm fragments.
Perfusion-based culture in bioreactor
Breast cancer tissue fragments were installed between two discs (8-mm diameter) of shaped collagen type 1 porous scaffold pre-wetted with culture medium for 1 h inside a humidified incubator (37°C, 5% CO2). Scaffolds were discs punched from sheets of Ultrafoam Collagen Hemostat (Avitene), a water-insoluble, partial HCl salt of purified bovine dermal (corium) collagen formed as a sponge with interconnected pores.Citation17 The disk size (8 mm) was chosen to compensate between load capacity and tissue availability, and to keep the system tight enough to induce the culture medium to perfuse the tissue. Silicon adaptors and grids were arranged on top and bottom of the sandwich scaffold tissue assembly and placed into the U-CUP perfusion chamber (Cellec Biotek AG) following manufacturer's instructions. A U-CUP is defined as a U-shaped bioreactor system for 3D, scaffold-based, cell and tissue Culture Under Perfusion as described in Wendt et al. (2006).Citation19,38 The perfusion flow rate was set at 0.3 mL/min to guarantee a superficial velocity of 100 µm/sec.Citation39 Due to the high variability and low availability of tumor specimens, we choose a flow rate that was previously used in our laboratory to generate normal and tumor tissue construct and that has also been reported in literature to provide benefit in terms of cells survival and proliferation.Citation19,21,39-41 Fig. S1 shows a schematic representation of the system and experimental procedure.
Culture medium
Breast cancer tissue fragments were cultured in 8 mL per U-CUP of DMEM/F12 (Gibco) containing 10% autologous human serum (AHS), 1% GlutaMAX-I 100x (Gibco), 1% Kanamycin sulfate (Gibco), 1% HEPES 1M (Gibco), N-Acetyl-Cysteine 1 mM (Sigma-Aldrich), Nicotinamide 10 mM (Sigma-Aldrich), Epidermal Growth Factor (25 ng/mL, Stem Cell Technologies), thereafter referred to as complete medium.
Treatment with fulvestrant
To evaluate the possibility to perform ex-vivo chemotherapeutic treatments, breast luminal-A type cancer tissue fragments were cultured in perfused conditions for 7, 14, and 21 d in the presence of ER antagonist Fulvestrant (100 nM, Sigma-Aldrich) or the appropriate vehicle control.Citation42 All experiments were performed at least two times.
Treatment with pertuzumab
To evaluate the possibility to assess ex-vivo targeted drug effectiveness treatment, breast Her2 type cancer tissue fragments were cultured in perfused conditions for 7 d in the presence of anti Her2 monoclonal antibody Pertuzumab (255 µg/mL, Perjeta®) or the appropriate vehicle control.
In vitro stimulation of tumor-infiltrating lymphocytes
To assess the functionality of TILs in U-CUP cultured breast cancer, tissue fragments were treated with PHA 1 µg/mL at day 0. Histomorphological analyses were performed after 4, 7, and 10 d of culture. All experiments were performed at least two times.
In vitro treatment with therapeutic monoclonal antibodies recognizing immunological checkpoints
At day 0, U-CUP cultured breast cancer tissue fragments were treated with either anti-PD-L1 (10 µg/mL, MDX-1105/BMS-936559, Bristol Myers Squibb) or anti-CTL4 (10 µg/mL MDX-010/BMS-734016, Bristol Myers Squibb), or a combination of both. Histomorphological analyses and RT-qPCR were performed after 4 and 7 d of treatment. All experiments were performed at least two times.
Histomorphological assessment and immunohistochemistry
Following culture, sandwich scaffolds containing breast cancer tissues were fixed in formalin 4% for at least 24 h, cut in half and embedded in paraffin. 4-μm sections were cut and stained for hematoxylin and eosin (HE). Immunohistochemical analysis was performed by using reagents specific for CK22 (Clone AE1/AE3/PCK26, prediluted, Ventana), CD3 (Clone 2GV6, prediluted, Ventana), CD20 (Clone L26, prediluted, Ventana), CD68 (Clone PG-M1, prediluted, Dako), Ki-67 (Clone Mib-1, prediluted, Dako), ERα (Clone SP1, prediluted, Ventana), PD-1 (Clone NAT105, prediluted, Ventana), PD-L1 (Clone E1L3N, dilution 1:50, CellSignaling), Caspase 3 (Clone DR6Y, CellSignaling), FoxP3 (Clone SP97, Abcam), Stat1-phospho (Clone 58D6, dilution 1:50, Cellsignaling), Stat3-phospho (Clone D3A7, dilution 1:50, Cellsignaling), and Phosphohistone H3 (polyclonal, prediluted, Ventana). For all immunohistochemical stainings, DAB was used as a chromogen.
Assessment of vtc and lymphocytes
Histologic slides were assessed by a board certified breast pathologist (S.M.). Viable cancer cells, identified by negative staining for cleaved Caspase 3, were counted per high-power field (hpf) (field diameter 0.625 mm) in the primary tissue as well as in the scaffold. Because of the small size of the tissue areas, automated counting by an analysis software was not used, since it would not have added any further information. If the cancer cells in the primary tissue were evenly distributed, one representative hpf was counted. If the distribution was uneven, with large necrotic areas next to viable cancer tissue, the number of viable cells was averaged across the number of hpf. For the assessment of infiltration of cancer cells into the scaffold, the most densely infiltrated area was counted. If there was doubt about the epithelial nature of the cells, CK22 staining was performed, and only positive cells were counted. Additionally, lymphocytes were counted per hpf in the primary tissue as well as in the scaffold. Again, to assess scaffold infiltration by lymphocytes, the most densely infiltrated area was counted. Additional immunohistochemical staining for CD3 and CD20 was performed, to distinguish between T- and B-lymphocytes.
RNA extraction and quantitative real-time PCR
Sandwich scaffolds containing chunks of breast cancer specimens were harvested after 7 d of culture in perfused bioreactor. Total cellular RNA was extracted using the Trizol reagent (Invitrogen) according to the manufacturer's protocol, following mechanical homogenization in TissueLyser II system (Qiagen) using stainless steel beads (Qiagen). RNA concentration and purity were determined using NanoDrop1 ND-1000 Spectrophotometer (NanoDrop Technologies). RNA (1 μg) was reverse transcribed using M-MLV reverse transcriptase (Invitrogen) following manufacturer's protocol, and cDNA were amplified and analyzed by quantitative real-time PCR (qRT-PCR) using ABI Prism 7300 (Applied Biosystems). Primers used to evaluate the expression by qRT-PCR are listed in Table S1. Specific gene expression was normalized by using human glyceraldehyde-3-phosphate dehydrogenase (GAPDH) housekeeping gene as reference.
Next generation sequencing
H&E stained FFPE tissue sections of tumor biopsy samples and corresponding cultured tumor samples were reviewed by an experienced pathologist (S.M.) to mark the area of interest and to assess tumor content. Specimens with a minimum of 20% tumor cells and no signs of extensive necrosis were used in the study. From such samples, unstained sections of 5 μM thickness were freshly cut, deparaffinized, and microdissected using H&E staining as a guide. DNA extraction and purification were performed using the fully automated Maxwell system (Promega) and quantified with the Qubit DNA HS assay kit (Invitrogen).
Libraries preparation using the Ion AmpliSeq™ Cancer Hotspot Panel v2 panel and the Ion AmpliSeq™BRCA1 and BRCA2 Panel (both from Life Technologies) was performed following the manufacturer's instructions, as described previously.Citation43,44 Briefly, the Ion AmpliSeq™ Cancer Hotspot Panel v2 panel was designed to allow amplification-based capture and sequencing of coding regions of 50 cancer-related genes (http://tools.thermofisher.com/content/sfs/brochures/Ion-AmpliSeq-CancerHotspot-Panel Flyer.pdf). The Ion AmpliSeq™ BRCA1 and BRCA2 Panel (http://tools.thermofisher.com/content/sfs/brochures/BRCA12_Panel_Flyer.pdf) was designed to sequence the entire coding regions of both BRCA1 and BRCA2. Targets enrichment was performed using genomic DNA mixed with primer pools and the Ion AmpliSeq™ HiFi master mix (Ion AmpliSeq™ Library Kit 2.0, Life Technologies) for 2 min at 99°C, followed by 22 cycles of 99°C for 15 sec and 60°C for 4 min and holding at 10°C. The PCR products were treated with 2 μL FuPa reagent (Ion AmpliSeq™ Library Kit 2.0, Life Technologies) to partially digest the primer sequences. Phosphorylation at 50°C for 10 min was followed by 10 min incubation at 55°C, and, finally, 20 min incubation at 60°C. The generated amplicons were ligated to Ion beads adapters and sample-specific barcodes from the Ion Xpress™ Barcode Adapters kit (Life Technologies) for 30 min at 22°C and then at 72°C for 20 min. Afterwards, barcoded libraries were purified using Agencourt® AMPure® XP reagents (Beckman Coulter). The library concentration was determined using an Ion Library Quantitation Kit (Life Technologies) and the Bioanalyzer 2100 (Agilent). 30 pM for each library were loaded into the IonChef System using the IC Hi-Q sequencing Kit. 318v2 were used for the Cancer Hotspot Panel and PIv3 for the BRCA1 and BRCA2 Panel). Afterwards, loaded chips were sequenced using the Ion PGM for the Cancer Hotspot Panel v2 (500 flows) and the Ion Proton for the BRCA1/2 panel (520 flows), respectively.
Raw data (BAM files) for each sample were processed for the alignment of sequencing reads with the human genome reference (hg19, tmap-f3) using the Torrent Suite software v4.6.11–1 (Life Technologies). The alignment pipeline also included signaling processing, base calling, quality score assignment, adaptor trimming, PCR duplicate removal, and control of mapping quality. Local re-alignment, duplicate removal, and quality base score recalibration were as well performed using the Genome Analysis Toolkit (GATK). Coverage metrics for each amplicon (minimal acceptable coverage threshold was set at 100×) was obtained by running the Coverage Analysis Plugin software v4.6.0.3 (Life Technologies). Base calling was performed using the IonReporter v4.6 (Life Technologies). In additions, also MuTectCitation45 and Varscan246 algorithms were used to call somatic single nucleotide variants (SNVs) and Varscan2,Citation46 Strelka,Citation47 and ScalpelCitation48 for insertions and deletions (indels). These specific mutations callers were chosen as they are designed to identify somatic mutations down to 1–2% mutant allele fraction (MAF) (i.e., 1–2% of the reads harboring a given mutation). Finally, all candidate mutations were manually reviewed using the Integrative Genomics Viewer.Citation49 Additionally, the Breast Cancer Information Core (BIC) (https://research.nhgri.nih.gov/projects/bic/index.shtml) and ClinVar database (http://www.ncbi.nlm.nih.gov/clinvar/) were used as reference to identify clinically revenant alterations.
Statistical analysis
Statistical analysis was performed using GraphPad Prism version 7.0c, GraphPad Software, La Jolla California USA, www.graphpad.com. To compare groups, the Wilcoxon matched-pairs signed rank test was applied when possible. When pairing match was not possible, Mann–Whitney test was used. Two sided p-values were considered significant if <0.05 (*p≤ 0.05; **p≤ 0.01; ***p≤ 0.001; ****p≤ 0.0001;). Because of the small cohorts' sizes no correction for exact significance was performed. Data are presented as mean ± SD.
Disclosure of potential conflicts of interest
Manuele G. Muraro and Giulio C. Spagnoli are Cellec Biotek AG share-holders.
Author contributions
M.G.M. set up, planned, and performed all the bioreactor experiments, provided technical support for this technology, contributed to the figures, and wrote part of the manuscript. S.M. did the histologic analyses, provided the histological images for the figures, and wrote part of the manuscript. S.D.S. planned, sponsored, coordinated, and supervised the experiments, provided breast cancer samples, and wrote part of the manuscript. A.T. did the statistical analysis, wrote the statistics part of the manuscript, and critically revised the manuscript. V.M. performed PCR, and ELISA analysis, wrote part of the manuscript, and critically revised it. L.Q. performed NGS, wrote part of the manuscript, and critically revised it. W.P.W. provided breast cancer samples and critically revised the manuscript. G.I. had previously helped to establish the culture system. G.S. contributed to the planning of the experiments and critically revised the manuscript.
Supplementary_materials.zip
Download Zip (10 MB)Acknowledgments
We thank Christian Hirt for his help in setting up the method of U-CUP culture. We further thank David Blattner for his help with the specimen handling. We also thank Michèle Baumann for the embedding, processing and staining of the slides, Petra Hirschmann for the IHC staining, and the collaboration of Elisabeth Adams for her support with the Pertuzumab experiments. This work was supported by the Holcim Foundation for the Advancement of Scientific Research, the Claudia von Schilling Foundation for Breast Cancer Research, the Freiwillge Akademische Gesellschaft Basel and the Huggenberger-Bischoff Foundation.
References
- Parkin DM, Fernandez LM. Use of statistics to assess the global burden of breast cancer. Breast J 2006; 12(Suppl 1):S70-80; PMID:16430400; https://doi.org/10.1111/j.1075-122X.2006.00205.x
- DeRose YS, Wang G, Lin YC, Bernard PS, Buys SS, Ebbert MT, Factor R, Matsen C, Milash BA, Nelson E et al. Tumor grafts derived from women with breast cancer authentically reflect tumor pathology, growth, metastasis and disease outcomes. Nat Med 2011; 17:1514-20; PMID:22019887; https://doi.org/10.1038/nm.2454
- Gibson J. Anti-PD-L1 for metastatic triple-negative breast cancer. Lancet Oncol 2015; 16:e264; PMID:25936988; https://doi.org/10.1016/S1470-2045(15)70208-1
- Emens LA, Braiteh FS, Cassier P, Delord J-P, Eder JP, Fasso M, Xiao Y, Wang Y, Molinero L, Chen DS et al. Abstract 2859: Inhibition of PD-L1 by MPDL3280A leads to clinical activity in patients with metastatic triple-negative breast cancer (TNBC). Cancer Res 2015; 75:2859; https://doi.org/10.1158/1538-7445.AM2015-2859
- Nanda R, Chow LQ, Dees EC, Berger R, Gupta S, Geva R, Pusztai L, Pathiraja K, Aktan G, Cheng JD et al. Pembrolizumab in patients with advanced triple-negative breast cancer: Phase Ib KEYNOTE-012 study. J Clin Oncol 2016; 34:2460-7; PMID:27138582; https://doi.org/10.1200/JCO.2015.64.8931
- Lieu CH, Tan AC, Leong S, Diamond JR, Eckhardt SG. From bench to bedside: Lessons learned in translating preclinical studies in cancer drug development. J Natl Cancer Inst 2013; 105:1441-56; PMID:24052618; https://doi.org/10.1093/jnci/djt209
- Siolas D, Hannon GJ. Patient-derived tumor xenografts: Transforming clinical samples into mouse models. Cancer Res 2013; 73:5315-9; PMID:23733750; https://doi.org/10.1158/0008-5472.CAN-13-1069
- Gould SE, Junttila MR, de Sauvage FJ. Translational value of mouse models in oncology drug development. Nature Med 2015; 21:431-9; PMID:25951530; https://doi.org/10.1038/nm.3853
- Mele V, Muraro MG, Calabrese D, Pfaff D, Amatruda N, Amicarella F, Kvinlaug B, Bocelli-Tyndall C, Martin I, Resink TJ et al. Mesenchymal stromal cells induce epithelial-to-mesenchymal transition in human colorectal cancer cells through the expression of surface-bound TGF-beta. Int J Cancer 2014; 134:2583-94; PMID:24214914; https://doi.org/10.1002/ijc.28598
- Ingber DE. Cancer as a disease of epithelial-mesenchymal interactions and extracellular matrix regulation. Differentiation 2002; 70:547-60; PMID:12492496; https://doi.org/10.1046/j.1432-0436.2002.700908.x
- Correia AL, Bissell MJ. The tumor microenvironment is a dominant force in multidrug resistance. Drug Resist Updat 2012; 15:39-49; PMID:22335920; https://doi.org/10.1016/j.drup.2012.01.006
- Junttila MR, de Sauvage FJ. Influence of tumour micro-environment heterogeneity on therapeutic response. Nature 2013; 501:346-54; PMID:24048067; https://doi.org/10.1038/nature12626
- Cerino G, Gaudiello E, Grussenmeyer T, Melly L, Massai D, Banfi A, Martin I, Eckstein F, Grapow M, Marsano A. Three dimensional multi-cellular muscle-like tissue engineering in perfusion-based bioreactors. Biotechnol Bioeng 2015; 113(1):226-36; PMID:26126766; https://doi.org/10.1002/bit.25688
- Santoro R, Olivares AL, Brans G, Wirz D, Longinotti C, Lacroix D, Martin I, Wendt D. Bioreactor based engineering of large-scale human cartilage grafts for joint resurfacing. Biomaterials 2010; 31:8946-52; PMID:20800280; https://doi.org/10.1016/j.biomaterials.2010.08.009
- Scherberich A, Galli R, Jaquiery C, Farhadi J, Martin I. Three-dimensional perfusion culture of human adipose tissue-derived endothelial and osteoblastic progenitors generates osteogenic constructs with intrinsic vascularization capacity. Stem Cells 2007; 25:1823-9; PMID:17446558; https://doi.org/10.1634/stemcells.2007-0124
- Martin I, Wendt D, Heberer M. The role of bioreactors in tissue engineering. Trends Biotechnol 2004; 22:80-6; PMID:14757042; https://doi.org/10.1016/j.tibtech.2003.12.001
- Radisic M, Euloth M, Yang L, Langer R, Freed LE, Vunjak-Novakovic G. High-density seeding of myocyte cells for cardiac tissue engineering. Biotechnol Bioeng 2003; 82:403-14; PMID:12632397; https://doi.org/10.1002/bit.10594
- Sabatino MA, Santoro R, Gueven S, Jaquiery C, Wendt DJ, Martin I, Moretti M, Barbero A. Cartilage graft engineering by co-culturing primary human articular chondrocytes with human bone marrow stromal cells. J Tissue Eng Regen Med 2012; 9(12):1394-403; PMID:23225781; https://doi.org/10.1002/term.1661
- Hirt C, Papadimitropoulos A, Muraro MG, Mele V, Panopoulos E, Cremonesi E, Ivanek R, Schultz-Thater E, Droeser RA, Mengus C et al. Bioreactor-engineered cancer tissue-like structures mimic phenotypes, gene expression profiles and drug resistance patterns observed “in vivo.” Biomaterials 2015; 62:138-46; PMID:26051518; https://doi.org/10.1016/j.biomaterials.2015.05.037
- Fong EL, Santoro M, Farach-Carson MC, Kasper FK, Mikos AG. Tissue engineering perfusable cancer models. Curr Opin Chem Eng 2014; 3:112-7; PMID:24634812; https://doi.org/10.1016/j.coche.2013.12.008
- Santoro M, Lamhamedi-Cherradi SE, Menegaz BA, Ludwig JA, Mikos AG. Flow perfusion effects on three-dimensional culture and drug sensitivity of ewing sarcoma. Proc Natl Acad Sci U S A 2015; 112:10304-9; PMID:26240353; https://doi.org/10.1073/pnas.1506684112
- Hsieh CH, Chen YD, Huang SF, Wang HM, Wu MH. The effect of primary cancer cell culture models on the results of drug chemosensitivity assays: the application of perfusion microbioreactor system as cell culture vessel. Biomed Res Int 2015; 2015:470283; PMID:25654105; https://doi.org/10.1155/2015/470283
- Majumder B, Baraneedharan U, Thiyagarajan S, Radhakrishnan P, Narasimhan H, Dhandapani M, Brijwani N, Pinto DD, Prasath A, Shanthappa BU et al. Predicting clinical response to anticancer drugs using an ex vivo platform that captures tumour heterogeneity. Nat Commun 2015; 6:6169; PMID:25721094; https://doi.org/10.1038/ncomms7169
- Bissell MJ, Radisky D. Putting tumours in context. Nat Rev Cancer 2001; 1:46-54; PMID:11900251; https://doi.org/10.1038/35094059
- Weigelt B, Bissell MJ. Unraveling the microenvironmental influences on the normal mammary gland and breast cancer. Semin Cancer Biol 2008; 18:311-21; PMID:18455428; https://doi.org/10.1016/j.semcancer.2008.03.013
- Hirt C, Papadimitropoulos A, Mele V, Muraro MG, Mengus C, Iezzi G, Terracciano L, Martin I, Spagnoli GC. “In vitro” 3D models of tumor-immune system interaction. Adv Drug Deliv Rev 2014; 79-80:145-54; PMID:24819215; https://doi.org/10.1016/j.addr.2014.05.003
- Guven S, Mehrkens A, Saxer F, Schaefer DJ, Martinetti R, Martin I, Scherberich A. Engineering of large osteogenic grafts with rapid engraftment capacity using mesenchymal and endothelial progenitors from human adipose tissue. Biomaterials 2011; 32:5801-9; PMID:21605897; https://doi.org/10.1016/j.biomaterials.2011.04.064
- Zardavas D, Irrthum A, Swanton C, Piccart M. Clinical management of breast cancer heterogeneity. Nat Rev Clin Oncol 2015; 12:381-94; PMID:25895611; https://doi.org/10.1038/nrclinonc.2015.73
- Gerlinger M, Rowan AJ, Horswell S, Larkin J, Endesfelder D, Gronroos E, Martinez P, Matthews N, Stewart A, Tarpey P et al. Intratumor heterogeneity and branched evolution revealed by multiregion sequencing. N Engl J Med 2012; 366:883-92; PMID:22397650; https://doi.org/10.1056/NEJMoa1113205
- Shah SP, Roth A, Goya R, Oloumi A, Ha G, Zhao Y, Turashvili G, Ding J, Tse K, Haffari G et al. The clonal and mutational evolution spectrum of primary triple-negative breast cancers. Nature 2012; 486:395-9; PMID:22495314; https://doi.org/10.1038/nature10933
- Navin N, Kendall J, Troge J, Andrews P, Rodgers L, McIndoo J, Cook K, Stepansky A, Levy D, Esposito D et al. Tumour evolution inferred by single-cell sequencing. Nature 2011; 472:90-4; PMID:21399628; https://doi.org/10.1038/nature09807
- Yachida S, Jones S, Bozic I, Antal T, Leary R, Fu B, Kamiyama M, Hruban RH, Eshleman JR, Nowak MA et al. Distant metastasis occurs late during the genetic evolution of pancreatic cancer. Nature 2010; 467:1114-7; PMID:20981102; https://doi.org/10.1038/nature09515
- Moya-Horno I, Cortes J. The expanding role of pertuzumab in the treatment of HER2-positive breast cancer. Breast Cancer (Dove Med Press) 2015; 7:125-32. PMID:26056489; https://doi.org/10.2147/BCTT.S61579
- Topalian SL, Hodi FS, Brahmer JR, Gettinger SN, Smith DC, McDermott DF, Powderly JD, Carvajal RD, Sosman JA, Atkins MB et al. Safety, activity, and immune correlates of anti-PD-1 antibody in cancer. N Engl J Med 2012; 366:2443-54; PMID:22658127; https://doi.org/10.1056/NEJMoa1200690
- Muenst S, Soysal SD, Tzankov A, Hoeller S. The PD-1/PD-L1 pathway: Biological background and clinical relevance of an emerging treatment target in immunotherapy. Expert Opin Ther Targets 2015; 19:201-11; PMID:25491730; https://doi.org/10.1517/14728222.2014.980235
- Nanda R CL, Dees E. A phase Ib study of pembrolizumab (MK-3475) in patients with advanced triple-negative breast cancer. Presented at 2014 San Antonio Breast Cancer Symposium 2014:Dec 9–13. Journal of Clinical Oncology 34, no. 21 (July 2016) 2460-2467; https://doi.org/10.1200/JCO.2015.64.8931
- Franco NH, Olsson IA. Scientists and the 3 Rs: Attitudes to animal use in biomedical research and the effect of mandatory training in laboratory animal science. Lab Anim 2014; 48:50-60; PMID:23940123; https://doi.org/10.1177/0023677213498717
- Wendt D, Stroebel S, Jakob M, John GT, Martin I. Uniform tissues engineered by seeding and culturing cells in 3D scaffolds under perfusion at defined oxygen tensions. Biorheology 2006; 43:481-8; PMID:16912419
- Cioffi M, Kuffer J, Strobel S, Dubini G, Martin I, Wendt D. Computational evaluation of oxygen and shear stress distributions in 3D perfusion culture systems: Macro-scale and micro-structured models. J Biomech 2008; 41:2918-25; PMID:18789444; https://doi.org/10.1016/j.jbiomech.2008.07.023
- Braccini A, Wendt D, Jaquiery C, Jakob M, Heberer M, Kenins L, Wodnar-Filipowicz A, Quarto R, Martin I. Three-dimensional perfusion culture of human bone marrow cells and generation of osteoinductive grafts. Stem Cells 2005; 23:1066-72; PMID:16002780; https://doi.org/10.1634/stemcells.2005-0002
- Radisic M, Deen W, Langer R, Vunjak-Novakovic G. Mathematical model of oxygen distribution in engineered cardiac tissue with parallel channel array perfused with culture medium containing oxygen carriers. Am J Physiol Heart Circ Physiol 2005; 288:H1278-89; PMID:15539422; https://doi.org/10.1152/ajpheart.00787.2004
- Hutcheson IR, Goddard L, Barrow D, McClelland RA, Francies HE, Knowlden JM, Nicholson RI, Gee JM. Fulvestrant-induced expression of ErbB3 and ErbB4 receptors sensitizes oestrogen receptor-positive breast cancer cells to heregulin beta1. Breast Cancer Res 2011; 13:R29; PMID:21396094; https://doi.org/10.1186/bcr2848
- Dacheva D, Dodova R, Popov I, Goranova T, Mitkova A, Mitev V, Kaneva R. Validation of an NGS approach for diagnostic BRCA1/BRCA2 mutation testing. Mol Diagn Ther 2015; 19:119-30; PMID:25893891; https://doi.org/10.1007/s40291-015-0136-5
- Simen BB, Yin L, Goswami CP, Davis KO, Bajaj R, Gong JZ, Peiper SC, Johnson ES, Wang ZX. Validation of a next-generation-sequencing cancer panel for use in the clinical laboratory. Arch Pathol Lab Med 2015; 139:508-17; PMID:25356985; https://doi.org/10.5858/arpa.2013-0710-OA
- Cibulskis K, Lawrence MS, Carter SL, Sivachenko A, Jaffe D, Sougnez C, Gabriel S, Meyerson M, Lander ES, Getz G. Sensitive detection of somatic point mutations in impure and heterogeneous cancer samples. Nat Biotechnol 2013; 31:213-9; PMID:23396013; https://doi.org/10.1038/nbt.2514
- Koboldt DC, Zhang Q, Larson DE, Shen D, McLellan MD, Lin L, Miller CA, Mardis ER, Ding L, Wilson RK. VarScan 2: Somatic mutation and copy number alteration discovery in cancer by exome sequencing. Genome Res 2012; 22:568-76; PMID:22300766; https://doi.org/10.1101/gr.129684.111
- Saunders CT, Wong WS, Swamy S, Becq J, Murray LJ, Cheetham RK. Strelka: Accurate somatic small-variant calling from sequenced tumor-normal sample pairs. Bioinformatics 2012; 28:1811-7; PMID:22581179; https://doi.org/10.1093/bioinformatics/bts271
- Narzisi G, O'Rawe JA, Iossifov I, Fang H, Lee YH, Wang Z, Wu Y, Lyon GJ, Wigler M, Schatz MC. Accurate de novo and transmitted indel detection in exome-capture data using microassembly. Nat Methods 2014; 11:1033-6; PMID:25128977; https://doi.org/10.1038/nmeth.3069
- Robinson JT, Thorvaldsdottir H, Winckler W, Guttman M, Lander ES, Getz G, Mesirov JP. Integrative genomics viewer. Nat Biotechnol 2011; 29:24-6; PMID:21221095; https://doi.org/10.1038/nbt.1754