ABSTRACT
Verticillium wilt of olive (VWO), caused by Verticillium dahliae, is a major concern in many olive-growing countries. An efficient VWO control measure is the use of tolerant/resistant cultivars. Low information is available about olive secondary metabolites and its relationship with VWO tolerance. In this study, a comprehensive metabolic profiling of the roots of six olive cultivars differing in their level of tolerance/susceptibility to VWO was addressed. Potential changes in the metabolite profiles due to the presence of the pathogen were also assessed. A strong relationship between the quantitative basal composition of the root secondary metabolic profile and VWO tolerance/susceptibility of olive varieties was found. Tolerant cultivars showed higher content of secoiridoids, while the susceptible ones presented greater amounts of verbascoside and methoxypinoresinol glucoside. The presence of V. dahliae only caused few significant variations mostly restricted to the earliest times after inoculation. Thus, a rapid activation of biochemical-based root defense mechanisms was observed.
Key policy highlights
Quantitative differences of secondary metabolites in roots contribute to explain the tolerance/susceptibility of olive cultivars to Verticillium dahliae.
Higher basal content of secoiridoids correlate with tolerance, while greater concentration of verbascoside and methoxypinoresinol glucoside seem to be linked to susceptibility.
Few alterations are observed in the olive root metabolic profiles in the presence of the pathogen.
Changes in the root metabolic profile occur at early times after pathogen inoculation which suggests a rapid activation of a biochemical-based defense response against V. dahliae.
1. Introduction
Verticillium wilt of olive (VWO), caused by the soil-borne fungus Verticillium dahliae Kleb., is one of the most devastating diseases affecting olive (Olea europaea L. subsp. europaea var. europaea) cultivation worldwide (Montes-Osuna and Mercado-Blanco Citation2020). The consequences for this relevant crop range from the partial loss of production on affected branches to the total destruction of the tree (López-Escudero and Mercado-Blanco Citation2011). A number of contributing factors explain the difficulty to control the disease (Tsror Citation2011). Therefore, the implementation of an integrated management strategy is the best way to confront this vascular pathogen (López-Escudero and Mercado-Blanco Citation2011). Following this approach, the use of resistant/tolerant cultivars is the most sustainable and economically viable measure to reduce the severity and the spread of VWO (Valverde et al. Citation2021, Citation2023; Serrano et al. Citation2023). During the last decades, a considerable body of knowledge about screening, evaluation, and breeding for new genotypes/cultivars showing tolerance to VWO has been generated. However, no olive cultivar has so far been reported as fully resistant to VWO (Bubici and Cirulli Citation2012; Trapero et al. Citation2012, Citation2015; García-Ruiz et al. Citation2014; Montes-Osuna and Mercado-Blanco Citation2020).
Plant tolerance is determined by a combination of constitutive (prior to infection) and inducible (during pathogen attack) defense mechanisms (Thatcher et al. Citation2005). In the case of VWO several studies have demonstrated that the first defense mechanisms against V. dahliae take place in the roots (Gómez-Lama Cabanás et al. Citation2015; Gharbi et al. Citation2017; Leyva-Pérez et al. Citation2018). Basal defenses operating in olive roots are related with the morphology/architecture of the root system and with the composition of the root cell wall. Our previous studies showed that specific root structural traits increase the tolerance to V. dahliae in olive plants (Cardoni et al. Citation2021, Citation2022a). In addition, the layer composed of suberin and lignin deposition in the root cell wall is an important mechanical defense barrier preventing the entry of soil-borne pathogens (Ferreira et al. Citation2007; Sabella et al. Citation2018; Cardoni et al. Citation2022a). Other components of the cell wall that contribute to plant defense are phenolic compounds and, to a lesser extent, pentacyclic triterpenes, both classified as plant secondary metabolites (Uccella Citation2000). They may act as defense (against herbivores, microbes, viruses, or competing plants) and signal (to attract pollinating or seed-dispersing animals) compounds as well as protectors from ultraviolet radiation and oxidants (Kutchan Citation2001). Due to their antioxidant, antimicrobial, antifungal, antiviral, and anti-inflammatory activities, the majority of the studies carried out on these compounds are related to their pharmacological activities and therapeutic implications (Dzubak et al. Citation2006; Li et al. Citation2022; Liu et al. Citation2022). Unfortunately, little is known about their quantitative distribution in plants and even less about their role in plant’s defense. Regarding olive plants, the studies on pentacyclic triterpenoids are limited to leaf and fruit tissues and on the changes of these compounds during the olive ontogeny (Jäger et al. Citation2009; Guinda et al. Citation2010; Olmo-García et al. Citation2016, Citation2018).
Phenolic compounds constitute one of the most common and widespread groups of substances in plants (Ryan and Robards Citation1998). They are secondary metabolites derived from the shikimate-phenylpropanoids-flavonoids pathway, producing monomeric and polymeric phenols and polyphenols (Lattanzio and Cardinali Citation2006). They comprise secoiridoids, simple phenols, phenolic acids, flavonoids, and lignans. Plant phenols may be divided into two classes: (i) preformed phenols, synthesized during the normal development of plant tissues, and (ii) induced phenols, produced by plants in response to physical damage, infection, or when stressed by elicitors (i.e. heavy metal-salts, UV-irradiation, temperature, pathogens). It has been shown that preformed phenols and polyphenols, stored in the plant cell as inactive bound forms, can be readily converted into biologically active antibiotics by plant hydrolyzing enzymes (glycosidases) in response to pathogen attack (Link and Walker Citation1933; Johnson and Schaal Citation1952; Ruiz-Barba et al. Citation1991; Marsilio and Lanza Citation1998; Lattanzio and Cardinali Citation2006). If pre-existing antimicrobial phenols are not sufficient to stop the development of the infectious process, plant cells usually respond by increasing the level of those compounds at the infection site (inducible defense mechanisms), inhibiting pathogen enzymes involved in cell wall degradation (Kunej et al. Citation2020). Moreover, the increased level of phenols provides an adequate substrate to oxidative reactions catalyzed by polyphenol oxidase (PPO) and peroxidase (POD) that, consuming oxygen and producing fungitoxic quinones, make the medium unfavorable to the further development of pathogens (Pichersky and Gang Citation2000).
Induced phenols may also be synthesized constitutively but, additionally, their synthesis is often boosted under biotic or abiotic stress. In fact, if the basal defenses are overcome, elicitors produced by phytopathogenic microorganisms induce a cascade of defense responses in the cells of their host plants. Regarding olive plants, the studies on phenolic compounds are mainly focused on their antioxidant and organoleptic properties, and mostly on olive oil, drupes, and leaves (Kanakis et al. Citation2013; Termentzi et al. Citation2015). Very little is known about the composition of phenols in olive roots. For instance, a significant decrease in oleuropein and hydroxytyrosol concentrations during olive ripening has been reported in roots of the cultivar (cv.) Picual (Ortega-García and Peragón Citation2010). The olive root phenolic composition under water deficit conditions was also investigated by Mechri and co-workers, who reported that roots of water-stressed plants had high levels of total phenols, oleuropein being the predominant compound (Mechri et al. Citation2019). Skodra and co-authors found that phenolics such as hydroxytyrosol, vanillin, and acetoxypinoresinol were reduced in roots under salinity conditions (Skodra et al. Citation2021).
Regarding the role of phenols in olive defense mechanisms our knowledge is also scant, particularly in the case of V. dahliae. Baídez and co-workers showed that olive plants responded to V. dahliae infection by a rapid accumulation of phenols in the stem (e.g. phloem and xylem tissues), thereby restricting or slowing down the growth of the pathogen. These authors also tested the antifungal activity of eight different phenolic compounds against V. dahliae, showing that rutin, oleuropein, and luteolin-7-glucoside were the most toxic metabolites (Báidez et al. Citation2007). A negative association between the amount of V. dahliae DNA and total phenols and oleuropein content in olive root tissues has been reported (Markakis et al. Citation2010). No information is available about the relationship between the olive root metabolic profile and tolerance/susceptibility to V. dahliae. Furthermore, most of the studies performed on the role that olive root secondary metabolites can play in defense mechanisms against pathogens focused on the detection of specific compounds typically present in olive oil or drupes (e.g. oleuropein, verbascoside, quercetin, luteolin, hydroxytyrosol, and luteolin 7-O-glucoside) through HPLC analysis (Del Río et al. Citation2003; Báidez et al. Citation2006; Markakis et al. Citation2010). This type of targeted analysis does not provide a broad coverage of the olive root metabolome. Only one work dealing with a comprehensive metabolic profiling of olive roots is available so far (Michel et al. Citation2015). In this study, the authors carried out the qualitative characterization of different plant organs (leaf, stem, and root) of cvs. Koroneiki and Chetoui by liquid chromatography coupled to high-resolution mass spectrometry (LC-HRMS). However, to the best of our knowledge, the quantitative determination of most of the secondary metabolites present in olive roots has not yet been accomplished, partly due to the analytical challenges related to the quantification of compounds belonging to several chemical classes that, in addition, are found at different concentration ranges in this organ.
Therefore, the objectives of this study (and the hypotheses to-be-tested) were: (I) to explore potential differences in the composition of secondary metabolites present in roots of olive varieties differing in their tolerance level to VWO (the presence of high variability in the metabolic profiles of the different olive varieties would be expected); and (II) to investigate the involvement of secondary metabolites in root olive defense mechanisms in relation to the presence/absence of V. dahliae (the tolerance level to VWO could be related to the presence of specific compounds). To that end, a powerful multi-class LC-MS method capable of monitoring compounds from different chemical classes within a single run and using just one sample treatment was implemented.
2. Materials and methods
2.1. Chemicals and standards
LC mobile phases were prepared with deionized water produced by a Millipore Milli-Q system (Bedford, MA, USA), LC-MS grade acetonitrile purchased from Prolabo (Paris, France) and acetic acid from Sigma-Aldrich (St. Louis, MO, USA). Gradient grade ethanol (EtOH), used for analytes extraction, was supplied by Prolabo. Analytical standards of tyrosol (CAS: 501-94-0), hydroxytyrosol (CAS: 10597-60-1), verbascoside (CAS: 61276-17-3), oleuropein (CAS: 32619-42-4), maslinic acid (CAS: 4373-41-5), oleanolic acid (CAS: 508-02-1), and betulinic acid (CAS: 472-15-1) were acquired from Sigma-Aldrich. Standards of oleuropein aglycone (CAS: 31773-95-2) and ligstroside (CAS: 35897-92-8) were provided by Toronto Research Chemicals (Toronto, Canada). Stock solutions of pure standards were prepared in ethanol/water (80:20, v/v) and serially diluted to cover a concentration range from 0.1 to 500 mg L-1 for the external calibration curves. All the standards and samples were filtered through 0.22 μm nylon syringe filters (Clarinert, Agela Technologies, Torrace, CA, USA) and stored in dark flasks at −20°C until analysis. Mobile phases were filtered through a 0.45 μm nylon membrane filter (Nylaflo, Pall Corporation, Michigan, MI, USA).
2.2. Plant material, inoculation with V. dahliae, and root sampling
Five-month-old, self-rooted olive plants, purchased in a commercial nursery in Córdoba province (Southern Spain), were used in this study. Three of the olive cultivars (‘Frantoio,’ ‘Empeltre,’ and ‘Changlot Real’) are classified as tolerant to VWO while another three (‘Picual,’ ‘Hojiblanca,’ and ‘Lechín de Sevilla’) are reported as susceptible to V. dahliae (López-Escudero et al. Citation2004; Gómez-Lama Cabanás et al. Citation2015; Trapero et al. Citation2015, Citation2018; Valverde et al. Citation2021; Citation2023). This set of plants was used in a parallel bioassay to determine the root lignin content upon inoculation with V. dahliae (Cardoni et al. Citation2022a). Before the inoculation with the pathogen, plants were kept for two months in the greenhouse in order to acclimatize them to the experimental conditions of light, temperature, and relative humidity described in Cardoni and co-workers (Citation2022a). Then, 72 plants (12 for each variety) were inoculated with V. dahliae V937I (an isolate representative of the defoliating pathotype) by adding to each pot 150 mL of a conidia suspension in water (5·106 conidial/mL). These conidia were obtained by filtering through sterile cheesecloth V937I cultures grown in potato dextrose broth during seven days in the dark at 27°C and with a constant shaking (190 rpm). A set of 90 additional plants (15 for each variety) were watered just with 150 mL of tap water and used as control (non-inoculated). At time-point 0, and at 1, 2, 7, and 15 days after inoculation (DAI), six plants per variety (i.e. three V. dahliae-inoculated and three control plants) were sampled. The roots of each plant were uprooted, gently cleaned under tap water, rapidly frozen in liquid nitrogen, and stored at −80°C until further processing. Additionally, seven V. dahliae-inoculated and three control (non-inoculated) plants per variety were kept under the same greenhouse conditions up to 100 DAI to evaluate differences in the disease development. Results in this regard were earlier reported (Cardoni et al. Citation2022a).
2.3. Processing of root samples
Each root sample was lyophilized and ground to a fine powder in a 50 mL stainless steel mill jar with two tungsten beads (Ø 1.2 cm) using a MM 301 mixer mill (Retsch GmbH, Haan, Germany). To maximize the number of extracted compounds (belonging to different chemical classes) from the root matrix a solid-liquid extraction procedure was adopted according to Serrano-García and co-authors (Citation2022). Root tissues powder (0.1 g) was weighed in a conical centrifuge tube and vortexed for 2 minutes with 5 mL of EtOH/H2O mixture (60:40 v/v). After using ultrasound for 30 minutes to assist the release of the metabolites from root tissues, the tube was centrifuged at 8500 rpm for 6 min. These steps were repeated two times, the first one with EtOH/H2O (80:20, v/v) as extracting agent, and the last one with pure EtOH. All supernatants were combined, filtered through nylon membrane filter (see above) and stored at −20°C until use.
2.4. LC-MS methodology
Two LC-MS systems were used for sample analysis: a Waters Acquity UPLC H–Class system coupled to a Q-TOF SYNAPT G2 mass spectrometer (Waters, Manchester, UK) for qualitative purposes (acquisition of high-resolution MS data); and an Agilent 1260 LC system (Agilent Technologies, Waldbronn, Germany) coupled to a Bruker Daltonics Esquire 2000 IT mass spectrometer (Bruker Daltonik, Bremen, Germany) to carry out the quantification of the targeted analytes. In both cases, metabolites separation was done at 40°C on a Zorbax Extend C18 column (4.6 × 100 mm, 1.8 μm particle size, Agilent Technologies), according to the procedure earlier described (Serrano-García et al. Citation2022). A mobile phase gradient of acidified water (1% acetic acid, v/v) (Phase A) and acidified acetonitrile (1% acetic acid, v/v) (Phase B) was applied at a flow rate of 1 mL min-1. Solvent gradient was as follows: 10–25% Phase B from 0 to 10 min; 25–60% Phase B from 10 to 12 min; 60–80% Phase B from 12 to 14 min; 80–100% Phase B from 14 to 18 min, kept for 2 min; then return to initial conditions in 2 min and 3 min of re-equilibration time. MS data were acquired in negative polarity from 50 to 1000 m/z; the electrospray ionization source parameters were selected in accordance to the LC flow and the particular configuration of the instrument used in each case (Serrano-García et al. Citation2022). MassLynx (Waters), Agilent ChemStation (Agilent Technologies), together with Esquire Control and Data Analysis 4.0 (Bruker Daltonik) were the software used for instrument control and MS data treatment.
2.5. Statistical analysis
To analyze the basal concentration of the compounds under study a heatmap (R package pheatmap) (Kolde Citation2018) was generated considering only the control (non-inoculated) plants at each sampling time. To assess possible differences among these compounds due to the olive genotype, the sampling times, the VWO tolerance level (VWO-tolerant vs. VWO-susceptible cultivars) or the presence/absence of V. dahliae (V. dahliae-treated vs. control plants), data were analyzed with one-way ANOVA (R function aov) considering separately the factors ‘variety,’ ‘time,’ ‘tolerance,’ and ‘treatment.’ The Tukey honestly-significant-difference with a p-level of 0.05 (R package agricolae) (De Mendiburu and Simon Citation2015) was used as post hoc test. Finally, to evaluate which compounds mostly influenced the differences among the four factors listed above a Principal Component Analysis (PCA) was carried out considering separately ‘variety,’ ‘time,’ ‘tolerance,’ and ‘treatment’ (R package factoextra) (Kassambara Citation2016).
3. Results
3.1. Qualitative characterization of olive roots metabolic profiles
From a qualitative point of view, the metabolic profiles obtained were similar for all root samples analyzed, regardless of the cultivar, the sampling time, the VWO tolerance level, and the presence/absence of the pathogen. Significant differences were found only at the quantitative level. The LC-MS chromatogram of a representative root sample is presented in Figure S1. The 31 most abundant compounds accounting for roughly 95% of the LC-MS chromatogram area are listed in . The acquired MS data (accurate mass of the main m/z signal corresponding to the pseudomolecular ion [M-H]-, as well as MS/MS main fragments for most of the compounds) and the information retrieved from MassLynx for molecular formulae calculation (theoretical mass, difference to the experimental mass and reliability of the mass shift and isotopic ratio of the proposed formula, i-FIT) are shown. When possible, the identity of the compound assigned to each chromatographic peak is displayed, together with the level of confidence in the annotation (Sumner et al. Citation2007).
Table 1. Secondary metabolites detected in olive root extracts by LC-ESI-QTOF MS.
Several phenolic glycosides were detected in the olive roots, namely hydroxytyrosol glucoside and three isomers of verbascoside, a caffeoyl phenylethanoid glycoside that has hydroxytyrosol and caffeic acid moieties in its structure, whose identity was confirmed with the corresponding pure standard. As far as secoiridoids are concerned, oleuropein, ligstroside, and oleuropein aglycone were unequivocally annotated by comparison of retention times and MS spectra with their authentic standards. The rest of the compounds belonging to this family have annotated thanks to the chromatographic and MS data (HRMS and MS/MS) and previous reports on secondary metabolites from olive tissues. This was the case of nuzhenide, dimethyl ligstroside, elenolic acid glucoside, acyclodihydroelenolic acid hexoside, and the compound with formula C16H22O11, which can be either oleoside or secologanoside (Michel et al. Citation2015; Olmo-García et al. Citation2018). Regarding lignans, five compounds were annotated based on the acquired MS information, relative retention times, spectral library searches, and available literature: acetoxypinoresinol, two isomeric peaks of C26H34O12, which had been already assigned to cycloolivil glucoside (Michel et al. Citation2015), together with 7,9ʹ:7ʹ,9-diepoxy-8,8ʹ-lignan-3,3ʹ,4,40,8-pentol, 3,3ʹ-Di-Me ether,4-O-ꞵ-D-glucopyranoside (hydroxypinoresinol glucoside), 7,9ʹ:7ʹ,9-diepoxy-8,8ʹ-lignan-3,3ʹ,4,4ʹ,5,8-hexol, 3,3ʹ,5-tri-Me ether,8-O-ꞵ-D-glucopyranoside (methoxypinoresinol glucoside) and 3,3ʹ,4,4ʹ,8-pentahydroxy-7,9ʹ:7ʹ,9-diepoxylignan,3,3ʹ-di-Me ether, 8-Ac,4-O-ꞵ-D-glucopyranoside (acetoxypinoresinol glucoside) (Al-Warhi et al. Citation2022). Three triterpenic acids were also identified by comparison with the analyzed pure standards: maslinic, betulinic, and oleanolic acids.
Besides the compounds mentioned above, ten additional peaks were annotated as ‘unknown’ (). Unknown_1, with molecular formula C25H38O18 must likely correspond to an elenolic acid diglucoside derivative since it showed two main fragments in its MS2 spectra: [M-H-C2O2H4]- (m/z 562.1767, C23H34O16) corresponding to elenolic acid diglucoside and [M-H-C2O2H4-glucose]- (m/z 403.1238, C17H24O11), which is the monoglycosidic form of elenolic acid. Unknown_2 (C28H44O9) and 3 (C28H44O8) could be related pregnane glycosides that have a C21 steroidal scaffold and a seco-pregnane-type glycoside. Unknown_4 and 5, with molecular formulas C21H30O13 and C21H30O12, respectively, are also related compounds only differing in the number of O atoms. The calculated formulas for unknown_6 and 8 matched eukovoside, a verbascoside derivate, and martynoside, a hydroxytyrosol derivative, respectively (Torres-Vega et al. Citation2021). Taking into account the analytical window in which they eluted (after triterpenic acids), both unknown_9 (C14H28O2) and 10 (C23H32O2) might belong to the fatty acids family and could be annotated as myristic acid and behenic acid, respectively. Contrariwise, a pseudo molecular formula containing just CHO atoms could not be calculated for unknown_7 since it presented an even m/z (504.1566). This indicated that the compound has an odd molecular mass (i.e. an odd number of nitrogen atoms in its molecular formula). Consequently, no tentative identity could be suggested for this compound.
3.2. Quantitative characterization of olive roots basal metabolic profiles
The quantification of the main detected metabolites was addressed in a subsequent step. Compounds lacking pure standards were quantified by using the external calibration curve of a reference compound belonging to the same metabolite subfamily or presenting a related chemical structure. This is a standard approach when the goal is not to find the absolute amount of the analytes but to compare samples or study the evolution of some compounds of interest (Olmo-García and Carrasco-Pancorbo Citation2021).
The basal composition of the control (non-inoculated) plant roots, calculated as the average of values scored for all replicates and at all sampling times, was mainly constituted by verbascoside, oleuropein, and elenolic acid glucoside (). As mentioned above (section 3.1) VWO-susceptible and VWO-tolerant cultivars showed similar metabolites composition. Nevertheless, quantitative differences between the two groups of varieties are worth mentioning. The first ones (‘Picual,’ ‘Lechín de Sevilla,’ and ‘Hojiblanca’) showed a significant higher concentration of acyclodihydroelenolic acid hexoside (p = 2.87·10−16 for ‘Picual’ and ‘Lechín de Sevilla’), maslinic acid (p = 3.32·10−10 for ‘Picual’ and ‘Lechín de Sevilla’), methoxypinoresinol glucoside (p = 2·10−16), oleanolic acid (p = 1.7510−06 for ‘Picual’ and ‘Lechín de Sevilla’), unknown_7 (p = 2.75·10−14 for ‘Hojiblanca’ and ‘Lechín de Sevilla’), and verbascoside (p = 1.38·10−11) compared with the VWO-tolerant cultivars (‘Changlot Real’, ‘Empeltre,’ and ‘Frantoio’). In contrast, ‘Frantoio’ and ‘Empeltre’ showed significantly higher concentration of elenolic acid glucoside, oleuropein, and oleuropein aglycone (p = 8.57·10−14, 1.77·10−7, and 3.64·10−7, respectively) ().
Table 2. Basal composition of metabolites scored for control (non-inoculated) olive plant roots.
Considering each sampling time (0, 1, 2, 7, and 15 DAI) the dataset of control plants could be grouped by ‘variety’ and ‘tolerance.’ Indeed, basal concentration values of all sampling time points clustered together for each variety, except for ‘Hojiblanca’ at 0 DAI and ‘Empeltre’ at 2 DAI (). Furthermore, VWO-susceptible (‘Lechín de Sevilla,’ ‘Hojiblanca,’ and ‘Picual’) and VWO-tolerant (‘Frantoio’ and ‘Empeltre’) varieties grouped separately, the three susceptible cultivars displaying more similar heatmaps among them than those showed by tolerant varieties. Interestingly enough, the constitutive metabolic profile of ‘Changlot Real’ plants appeared to be quite different to that scored for the rest of cultivars, including the other two tolerant varieties examined in this study. When carefully examining the clusters formed according to the analyzed compounds (), some interesting trends can be highlighted. The central cluster was mostly composed of secoiridoids and related compounds (unknown_1, oleuropein aglycone, nuzhenide, elenolic acid glucoside, and ligstroside). Overall, these metabolites showed higher concentrations in ‘Frantoio’ and ‘Empeltre’ roots compared with VWO-susceptible cultivars. In contrast, the pentacyclic triterpenes (maslinic and oleanolic acids), phenolic glycosides (unknown_4, 5, and 6 and verbascoside) and lignans (acetoxypinoresinol, acetoxypinoresinol glucoside, and methoxypinoresinol glucoside) grouped in the lower cluster () and showed overall higher concentrations in VWO-susceptible cultivars. It is also worth mentioning that some metabolites grouped in the upper cluster, such as oleuropein and unknown_8, showed higher abundances in ‘Empeltre’ and ‘Frantoio’ compared with the VWO-susceptible varieties.
Figure 1. Hierarchical clustering heatmap showing the quantitative basal metabolites composition present in roots of control (non-inoculated) olive plants at different sampling time points (t0, 1, 2, 7, 15 days). The concentration of each compound is the average value of three replicates (n = 3) per variety.
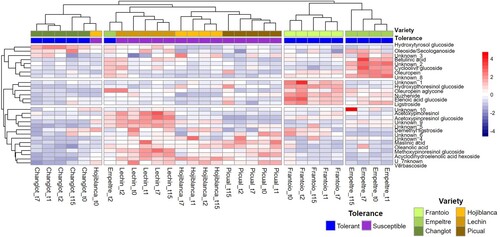
3.3. Metabolic profile variations over time
Most of the compounds found in olive roots did not show consistent significant variations either over time (Table S1) or between control and inoculated plants (Table S2). However, two groups of metabolites showing significant differences considering the factor ‘treatment’ can be highlighted (ANOVA p < 0.05). The first one was composed of metabolites showing significant variations between control and inoculated plants over time in VWO-tolerant cultivars. The second group was constituted by compounds exhibiting significant differences over time for the factor ‘treatment’ in VWO-susceptible varieties.
3.3.1. Time-course change of metabolite concentrations in VWO-tolerant cultivars
Overall, control plants of cvs. Empeltre and Frantoio showed higher concentrations of the secoridoid nuzhenide along time. Furthermore, this compound decreased significantly (p < 0.05) in the V. dahliae-inoculated plants of these varieties at 1 DAI ((A)). A similar trend was observed for unknown_3, that showed a significant decrease (p < 0.05) in inoculated plants of the VWO-tolerant varieties compared with the susceptible ones ((B)). Contrariwise, the pentacyclic triterpene oleanolic acid showed a significant increase (p < 0.05) in the same plants at 7 DAI. The same trend was detected for the VWO-susceptible varieties at 15 DAI ((C)). Finally, a significant increase (p < 0.05) of the unknown_5 metabolite was also detected in V. dahliae-inoculated plants of VWO-tolerant cultivars at early sampling times (between 1 and 2 DAI) ((D)).
Figure 2. Time course variation in the concentration of nuzhenide (A), unknown_3 (B), oleanolic acid (C), and unknown_5 (D). Green and ocher bars represent VWO-tolerant and VWO susceptible control (non-inoculated) plants, respectively, while black bars correspond to Verticillium dahliae-inoculated plants. Error bars show the standard deviation of three biological replicates (n = 3). Lowercase letters indicate Tukey’s post hoc test differences (p < 0.05) among control (black) and inoculated (red) plants. Statistical differences resulting from the ANOVA analysis between the control and inoculated plants are represented by asterisks (level of significance p < 0.05). DAI, days after inoculation.
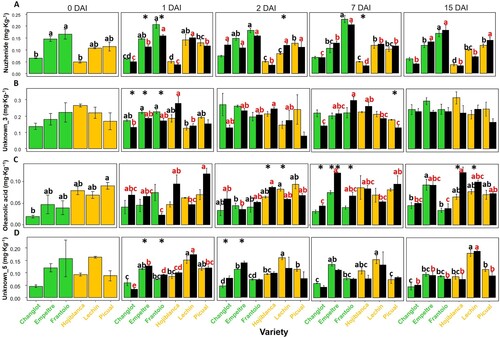
3.3.2. Time-course change of metabolite concentrations in VWO-susceptible cultivars
The unknown_7 metabolite showed an overall higher concentration in control plants of the VWO-susceptible cultivars during the whole experimental time, compared with the tolerant varieties ( and (A)). Moreover, this compound further increased its concentration at 1 DAI in the V. dahliae-inoculated plants of the former cultivars (significantly in the case of ‘Hojiblanca’ and ‘Lechín de Sevilla’ plants). This trend was not observed in VWO-tolerant varieties ((A)). Similarly, the secoridoid oleoside/secologanoside showed an increase only in the inoculated plants of VWO-susceptible cultivars at 7 DAI ((B)). In contrast, the unknown_2 metabolite decreased in this set of plants at 2 DAI ((C)). Likewise, at 1 DAI, the unknow_8 metabolite showed a lower concentration in V. dahliae-inoculated plants of the VWO-susceptible cultivars at 1 DAI (p < 0.05) ((D)). Finally, the unknown_9 metabolite also decreased between 2 and 15 DAI in ‘Hojiblanca’ and ‘Lechín de Sevilla’ inoculated plants (p < 0.05) ((E)).
Figure 3. Time course variation in the concentration of unknown_7 (A), oleoside/secologanoside (B), unknown_2 (C), unknown_8 (D), and unknown_9 (E). Green and ocher bars represent VWO-tolerant and VWO susceptible control (non-inoculated) plants, respectively, while black bars correspond to Verticillium dahliae-inoculated plants. Error bars show the standard deviation of three biological replicates (n = 3). Lower case letters indicate Tukey’s post hoc test differences (p < 0.05) among control (black) and inoculated (red) plants. Statistical differences resulting from the ANOVA analysis between the control and inoculated plants are represented by asterisks (level of significance p < 0.05). DAI, days after inoculation.
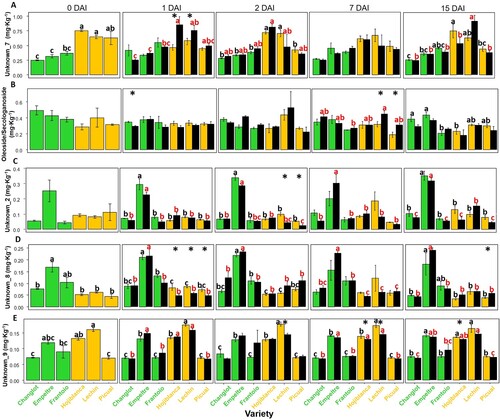
3.4. Contribution of root metabolites to explain differences between VWO-tolerant and VWO-susceptible cultivars
The PCA performed on the root metabolites explained more than 60% of the total variance (Dim. 1 = 31.9%, Dim. 2 = 28.4%), with a major contribution of unknown_5 (19.9%) and unknown_9 (16.6%) for the first axis, and unknown_ 2 (22%) and oleuropein (21.6%) for the second one. This statistical approach considered four different factors, namely ‘variety,’ ‘tolerance,’ ‘time,’ and ‘treatment.’ However, significant differences were observed only for the first two. In fact, the PCA showed no variations either for ‘time’ or for ‘treatment’ (Figure S2). The PCA separated VWO-tolerant and VWO-susceptible cultivars in two different groups on the first dimension ((A)), with a major influence of oleuropein, unknown_2, cycloolivil glucoside, and oleuropein aglycone for the first group, and maslinic acid, acyclodihydroelenolic acid hexoside, unknown_7, and methoxypinoresinol glucoside for the susceptible varieties, thus further supporting results shown in . Considering the factor ‘variety’ it is interesting to note that ‘Picual’ and ‘Hojiblanca’ clustered together on the left-lower quadrant, close to ‘Lechín de Sevilla’ (right-lower quadrant). On the other hand, the tolerant cultivars were more separated among them and grouped on the upper half of the plot ((B)).
Figure 4. Principal Component Analysis (PCA) of olive root metabolites performed with ‘tolerance’ and ‘variety’ as factors. Compounds with a contribution to the two dimensions higher than 4% were considered for the analysis. Verbascoside, acetoxypinoresinol glucoside, ligstroside, and unknown_8 were not considered because they were highly correlated with other metabolites that had a higher percentage of explanation (acyclodihydroelenolic acid hexoside, unknown_5, and oleuropein).
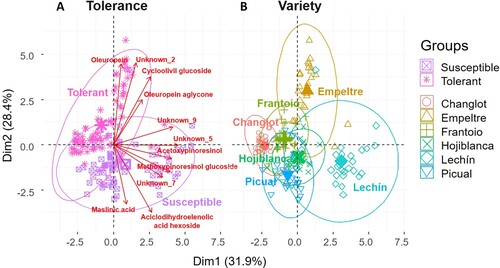
4. Discussion
In contrast to other olive-derived matrices such as fruit, oil, or leaf, olive roots have been scarcely studied from a compositional point of view (Báidez et al. Citation2006; Michel et al. Citation2015; Mechri et al. Citation2019). Therefore, in this study, the metabolic profile of roots from both control and V. dahliae-inoculated plants was exhaustively investigated by means of a multiclass LC-MS method capable of determining metabolites belonging to several chemical classes, including simple phenols, glycosides, lignans, secoiridoids, pentacyclic triterpenes, fatty acids, etc. Nine out of the 31 metabolites reported in were unequivocally identified (annotation confidence level 1) by comparison with their authentic standards, including a phenolic glycoside, three secoiridoids and three pentacyclic triterpenes. A group of 11 compounds were putatively annotated (confidence level 2) on the basis of their relative retention times, HRMS and MS/MS information retrieved from public spectral libraries and previous reports on olive-related matrices (Kanakis et al. Citation2013; Michel et al. Citation2015; Olmo-García et al. Citation2018; Al-Warhi et al. Citation2022). With regard to compounds annotated with a confidence level 3 (putatively characterized compound classes), it is worth mentioning that the compound named oleoside or secologanoside has been previously described in olive matrices. However, since both molecules are mass isomers sharing a very similar skeleton with a glucose moiety attached, we were unable to distinguish them without an available pure standard. In addition, tentative identities were proposed for 9 ‘unknowns’ as phytochemicals described in other plant tissues that have plausible chemical structures and belong to families of compounds already present in olive-related matrices. Thus, unknown_1 was classified as an elenolic acid diglucoside derivative thanks to the MS/MS data, as described in section 3.1. Unknowns_2 and 3 could be pregnane glycosides such as seco-illustrol-3-O-β-L-diginopyranosyl (dardanol D) isolated from Mandevilla dardanoi roots (Lins et al. Citation2022). Although the molecular formula C21H30O13 had been assigned to acetylbarlerin in olive fruit and leaf wastes (Cádiz-Gurrea et al. Citation2021), unknown_4, together with unknown_5, could also be benzoic acid allopyranosides such as Keteleeroside A and C, respectively, which had been previously found in twigs of Keteleeria evelyniana Mast (Wen et al. Citation2011). As far as unknown_6 is concerned, it could correspond to eukovoside, a verbascoside derivative previously isolated from Buddleja globosa Hope (Torres-Vega et al. Citation2021). These authors also reported martynoside in the same matrix, which is another hydroxytyrosol derivative that could match unknown_8 in the present study. Finally, unknown_9 and 10, were tentatively designated as saturated fatty acids previously found in trace amounts in olive oil (Boskou Citation2006). Just one compound (unknown_7) was labeled as Level 4 (unclassified) (). While no plausible identity was suggested for this one, differentiation and quantification of this metabolite were accomplished in this present study. Interestingly, eight of the determined compounds (i.e. hydroxytyrosol glucoside, verbascoside, oleoside or secologanoside, oleuropein, ligstroside, oleuropein aglycone, hydroxypinoresinol glucoside, and maslinic acid) were previously detected in roots of two Greek olive cultivars (Koroneki and Chetoui) using a similar analytical method (LC-HRMS) (Michel et al. Citation2015). However, cycloolivil glucoside, acetoxypynoresinol glucoside, and oleanolic acid were not detected in root tissues in that work, although their presence was reported in leaves and/or stems. Finally, it is important to note that no flavonoids were found in the roots of the cultivars assessed, what is in agreement with previous findings (Michel et al. Citation2015; Skodra et al. Citation2021).
While from the qualitative point of view no differences were detected, tolerant and susceptible cultivars here examined did show significant quantitative differences for several compounds. Indeed, considering either the entire time-course of the experiment or individual sampling times ( and ), ‘Frantoio’ and ‘Empeltre’ (VWO-tolerant varieties) showed a significantly higher constitutive content of oleuropein, oleuropein aglycone and elenolic acid glucoside. It is noteworthy that these compounds also mostly influenced the cluster formed by tolerant plants in the PCA analysis (). Oleuropein aglycone and elenolic acid glucoside are products of the enzymatic hydrolysis of oleuropein. The first step is the hydrolysis of the glycosidic linkage by β-glucosidase with the formation of oleuropein aglycone that, in a second step, is hydrolyzed to elenolic acid and hydroxytyrosol by esterase activity (Marsilio and Lanza Citation1998; Kanakis et al. Citation2013; Termentzi et al. Citation2015). Oleuropein and its aglycone, extracted from the fruit, showed in vitro antimicrobial activity against bacteria (Lactobacillus plantarum and Leuconostoc mesenteroides) and fungi (Rhizopus sp., Rhizoctonia solani, Geotrichum candidum, and Penicillium cyclopium) (Juven and Henis Citation1970). The antimicrobial proprieties of oleuropein, extracted from olive fruit and fermented brines, were also tested against Lactobacillus planyarum (Ruiz-Barba et al. Citation1991; Marsilio and Lanza Citation1998) and Staphylococcus aureus (Tassou and Nychas Citation1994). Furthermore, the same compound extracted from olive roots showed inhibitory activity in vitro against Phytophthora spp. (Del Río et al. Citation2003). These authors affirmed that the content of oleuropein in olive roots, together with tyrosol and catechin, contribute to impede the access of this oomycete, inhibiting mycelial growth and preventing its proliferation to the rest of the plant. In previous studies about olive response to cold (Ortega-García and Peragón Citation2009) and salt (Petridis et al. Citation2012) stresses in leaves and roots, respectively, oleuropein was described as a stress-tolerant agent. Olive plants accumulated this compound in different organs to protect themselves from oxidative damage (Ortega-García and Peragón Citation2009). Thus, it is plausible to think that the higher basal content of oleuropein and its derivatives present in the roots of VWO-tolerant plants, compared with that scored in the susceptible varieties, may be linked to the ability of the former group to overcome the disease. Considering individual sampling times (), other secoridoids such as ligstroside, nuzhenide, and unknown_1 (an elenolic acid derivative) also showed significant higher basal concentrations in root tissues of VWO-tolerant cultivars. Therefore, it can also be argued the existence of a link between a higher basal content of secoiridoids in olive roots and tolerance to V. dahliae. Nevertheless, further evidence is needed to confirm these hypotheses.
Regarding VWO-susceptible plants, three compounds were predominant in their basal root tissue composition, both considering single sampling times and the whole elapsed experimental: methoxypinoresinol glucoside, maslinic acid, and verbascoside ( and ). Furthermore, these compounds mostly influenced the cluster formed by the susceptible cultivars in the PCA analysis (). No information about the first compound is available in the literature. Concerning maslinic acid, most of the studies so far performed focused on clinical tests (antitumoral, antidiabetic, antioxidant, cardioprotective, and neuroprotective activities) (Deng et al. Citation2021). Moderate antifungal activity against Aspergillus flavus, Ustilago maydis, and A. niger has also been reported using in silico PASS (Prediction of Activity Spectra for Substances) analysis, although no inhibitory effect against different bacteria was found (Jamkhande et al. Citation2016). Conversely, maslinic acid extracted from olive leaves showed antimicrobial activity against oral streptococci and anaerobic pathogenic bacteria (Porphyromonas gingivalis, Fusobacterium nucleatum, Parvimonas micra) (Karygianni et al. Citation2019). So far, only one study has reported antimicrobial activity of this metabolite against plant pathogens. Indeed, in vitro antifungal activity of maslinic acid extracted from leaves of Cobretum erythrophyllum (Burch.) Sond. against six different pathogenic species of Fusarium has been demonstrated (Seepe et al. Citation2021). In this present study no significant variation in the concentrations of methoxypinoresinol glucoside and maslinic acid after V. dahliae inoculation was detected. Therefore, it is unlikely to ascribe any antifungal role (at least against V. dahliae) to these compounds. Furthermore, the significantly higher concentration of these metabolites in the roots of non-inoculated VWO-susceptible cultivars compared with that in the tolerant varieties may suggest a possible role, yet to be identified, in susceptibility to V. dahliae.
Verbascoside was the metabolite detected at the highest concentration in all olive roots here analyzed, what agrees with previous studies on the same matrix (Mechri et al. Citation2019, Citation2020). In olive plants infected by Pseudomonas savastanoi pv. savastanoi, the etiological agent of the olive knot disease, increased biosynthesis of this compound in the lesions (tumors) caused by this bacterium in the tree was associated with a plant defense mechanism (Cayuela et al. Citation2006). Contrariwise, no significant changes were found in our study for this compound after V. dahliae inoculation. A recent study has shown a reduction of verbascoside content in roots of water-stressed olive plants compared with well-watered plants (Mechri et al. Citation2019). It is worth mentioning that Verticillium wilt causes symptoms similar to those produced by water stress. Indeed, the invasion of the vascular system by V. dahliae hinders the water flow, triggering the plant physiological responses resembling those caused by drought stress (Ayele et al. Citation2020; Zarco-Tejada et al. Citation2021; Cardoni et al. Citation2022b). Our results may suggest a negative association between the content of verbascoside in olive roots and the tolerance level against this vascular pathogen. In fact, as for the previous two metabolites, its higher concentration in the basal metabolic profiles of the VWO-susceptible cultivars () suggested a correlation between verbascoside content and V. dahliae susceptibility. Considering the metabolic profile of non-inoculated plants at each sampling time, unknown_4, unknown_7, and acyclodihydroelenolic acid hexose also exhibited higher concentrations in VWO-susceptible cultivars compared with the tolerant ones. Therefore, a greater content of these metabolites may be linked to the susceptibility of cvs. Picual, Hojiblanca and Lechín de Sevilla to V. dahliae. Further studies about the role of these compounds in VWO tolerance/susceptibility would be convenient.
It is worth noticing that the basal metabolic profile of ‘Changlot Real’ was not only different from that of the VWO-susceptible cultivars but also from the profiles displayed by the other two VWO-tolerant varieties here assessed. While compounds found at significantly higher concentrations in the VWO-susceptible cultivars (i.e. methoxypinoresinol glucoside, maslinic acid, verbascoside, acyclodihydroelenolic acid hexose, unknown_4, and unknown_7) showed lower contents in ‘Changlot Real,’ metabolites with significantly higher values in the other tolerant cultivars (i.e. hydroxypinoresinol glucoside, oleuropein, oleuropein aglycone, elenolic acid glucoside, ligstroside, nuzhenide, and unknown_1) were also found at low concentration in the roots of this cultivar. An ‘intermediate’ or ‘discrepant’ behavior for this cultivar was earlier reported when examining the expression pattern of some defense-response genes (Gómez-Lama Cabanás et al. Citation2015). In this study, ‘Changlot Real’ plants exhibited some genes expression trends similar to those showed by cv. Frantoio while other genes displayed expression patterns alike to ‘Picual’ plants. Moreover, in another study, progenies obtained from ‘Frantoio’ and ‘Changlot Real’ parents yielded different results. Indeed, while the first cultivar produced a large number of VWO-tolerant seedlings, even when crossed with ‘Picual,’ ‘Changlot Real’ generated many susceptible descendants (Trapero et al. Citation2015). These results, together with the findings here reported, point to the fact that the level of tolerance of ‘Changlot Real’ to V. dahliae is lower compared to that displayed by ‘Empeltre’ and ‘Frantoio,’ and that an accurate qualification of this phenotype for the former variety still remains to be done (Serrano et al. Citation2020).
The impact that the inoculation with V. dahliae had on the composition (and changes over time) of the olive root metabolites analyzed in this study seemed to be minor (Table S2). This result was confirmed by the PCA analysis carried out with the factor ‘treatment’ that showed no differences among control and V. dahliae-inoculated plants (Figure S2). Similarly, no differences were detected considering the factor ‘time’ (Table S1 and Figure S2). The metabolites nuzhenide and unknown_3 exhibited a significant decrease in VWO-tolerant cultivars at the earliest sampling time point (1 DAI), suggesting an involvement of these compounds in defense mechanisms ((A,B)). Indeed, there are many phenolic compounds present in plant tissues with no antimicrobial activity per se. However, the oxidation products of these pre-existing phenols might have antimicrobial activity through inhibition of the cell wall degradation by extracellular enzymes produced by pathogens (Lattanzio and Cardinali Citation2006). Unfortunately, no information about the role of these metabolites in plant defense is available. The opposite trend was found for oleanolic acid and unknown_5 that showed an increase in the same cultivars upon inoculation with V. dahliae ((C,D)). Since in vitro antibacterial activity has been previously demonstrated for oleanolic acid (Chung et al. Citation2011), it is tempting to conjecture that these compounds may also have antagonist activity against V. dahliae. Interestingly, a late increase (15 DAI) was detected for this compound in VWO-susceptible cultivars. A faster and more effective upregulation of genes involved in lignin biosynthesis and activation of innate immunity was previously reported for VWO-tolerant cultivars compared with susceptible varieties (Cardoni et al. Citation2022a). Results here reported pointed to a similar scenario; that is, a rapid activation of biochemical-based defense mechanisms in VWO-tolerant plants but a delayed response in the susceptible varieties.
Five different metabolites showed significant alterations at early times after pathogen inoculation in the VWO-susceptible cultivars. Unknown_7 and oleoside/secologanoside showed an increase in the V. dahliae-inoculated plants compared with the controls ((A,B)). Instead, unknown compounds 2, 8, and 9 exhibited the opposite trend ((C–E)). While it is tempting to assign some role for these metabolites (and their contrasting variations over time upon V. dahliae interaction with the host plant) in the higher susceptibility displayed by these cultivars, additional evidence will be needed to confirm such hypothesis.
The great dissimilarity in the concentration of phenols and triterpenes found between the two groups of olive cultivars, as well as the almost null impact of the presence of the pathogen along time, suggested that the root basal metabolic profile is key to determine the VWO tolerance/susceptibility, at least under our experimental conditions. However, more factors (e.g. genetic, physiological, structural, and microbiological) must be taken into account to explain the tolerance/susceptibility level to V. dahliae of a given olive cultivar (Cardoni et al. Citation2022a). Furthermore, the few significant alterations detected after pathogen inoculation confirmed that the qualitative and quantitative metabolic composition of olive root is strongly related to pathogen tolerance, determining the efficiency of the plant to respond to the V. dahliae attack, as suggested in previous studies focused only on a limited number of compounds (Báidez et al. Citation2007; Markakis et al. Citation2010). Finally, it must be emphasized that many of the metabolites showing high concentrations in the basal metabolic profiles and significant changes after pathogen inoculation could not be fully identified (annotated as unknowns), although the chemical class has been proposed for some of them. This underlines the important gap of knowledge about secondary metabolites in olive roots in general, and on their role in defense mechanisms against soil-borne pathogens in particular.
5. Conclusions
A significant association between VWO tolerance level and the olive root constitutive metabolic profile has been established. Therefore, the first hypothesis to-be-tested in this study has been confirmed: there is variability in the root metabolic profiles of the olive varieties here examined, although this variability was quantitative and not qualitative. Besides, the presence of V. dahliae only caused minor significant changes in the composition of the secondary metabolites present in the roots. Consequently, the second hypothesis to-be-tested has been also confirmed: the VWO tolerance level of olive cultivars could be related to differences in the amount of specific compounds. The few significant alterations were all detected at early times after the inoculation with the pathogen (between 1 and 7 DAI), suggesting a rapid activation of a biochemical-based defense response. On the one hand, our findings stress the need to deepen our knowledge about the biochemical composition of olive roots, aiming at understanding the underlying mechanisms of resistance/tolerance to V. dahliae, and perhaps of other soil-borne pathogens. On the other hand, the results gathered could be relevant to be considered as additional selection criteria in breeding programs for VWO resistance.
Supplemental Material
Download MS Word (339.6 KB)Disclosure statement
No potential conflict of interest was reported by the author(s).
Additional information
Funding
Notes on contributors
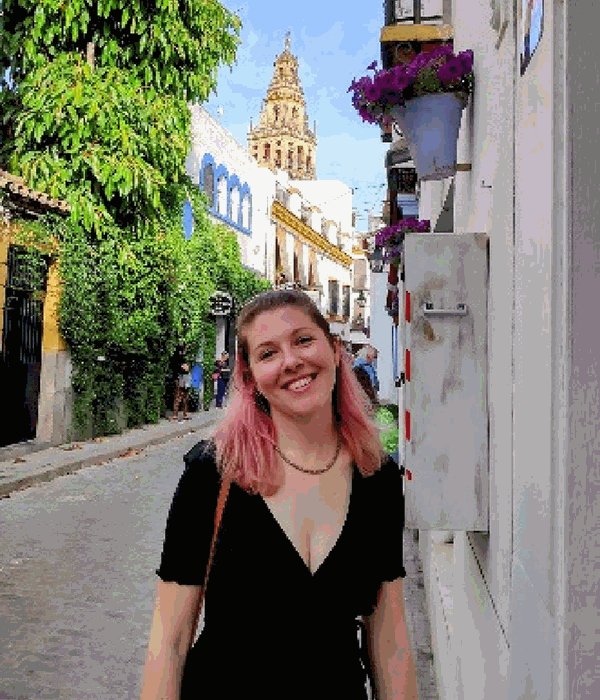
Martina Cardoni
Martina Cardoni is graduated in Environmental Science from the University of Tuscia (Viterbo, Italy) and has a MSc in Ecology from the University of “La Sapienza” (Rome, Italy). She is currently doing a PhD in Agricultural Engineering with the Plant-Microorganisms Interactions research group of the Institute for Sustainable Agriculture, Spanish National Research Council (IAS-CSIC). Her research is focused on the genetic and molecular mechanisms underlying the susceptibility/tolerance level of olive cultivars to Verticillium wilt of olive (VWO). Likewise, the characterization of the olive root microbiomes, to evaluate their possible implication in the susceptibility/tolerance level that olive genotypes show against VWO attacks.
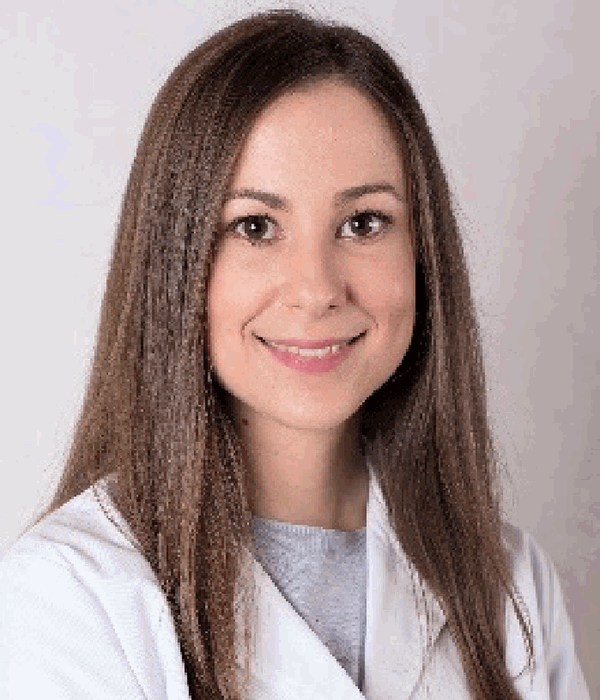
Lucía Olmo-García
Lucía Olmo-García is a doctor in Chemistry, with a strong background in Analytical Chemistry, including separative techniques (gas and liquid chromatography), mass spectrometry, and chemometrics. Her field of expertise comprises the application of different metabolomic strategies to the study of secondary metabolites in plants and food, as well as in biological fluids. She currently holds a Ramón y Cajal contract at the University of Granada.
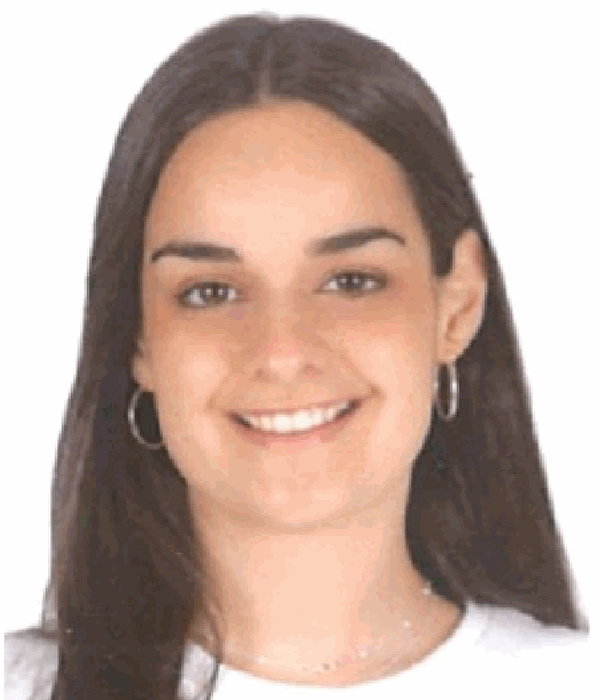
Irene Serrano-García
Irene Serrano-García has a Degree in Food Science and Technology from the University of Barcelona and a MSc in Chemical Technologies from the University of Granada, where she is currently doing a PhD in Food Metabolomics at the Department of Analytical Chemistry. She is working on the study and characterization of several olive tree derived matrices, vegetable oils, and avocado tissues by liquid chromatography coupled to mass spectrometry.
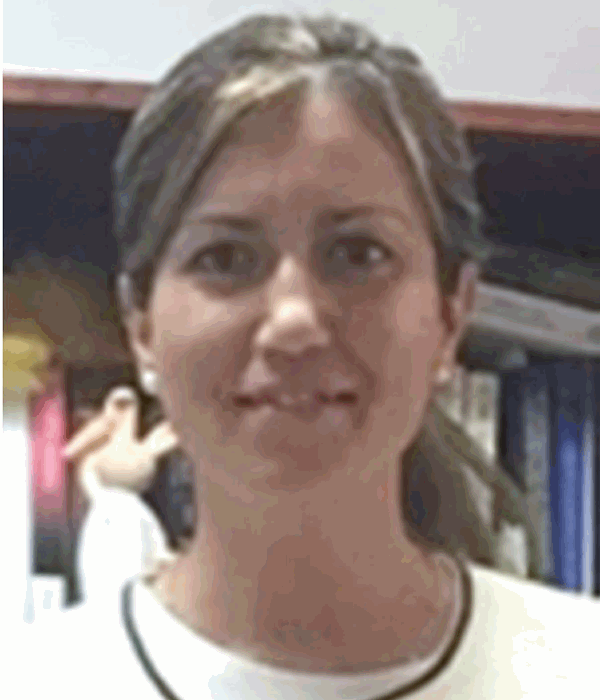
Alegría Carrasco-Pancorbo
Alegría Carrasco-Pancorbo is full Professor of the Department of Analytical Chemistry at the University of Granada since 2018. Her scientific contributions have mainly focused on the extraction of minor compounds of interest from food matrices (olive oil, tropical fruits, etc.) and their subsequent characterization using innovative analytical methodologies based on both targeted and non-targeted Metabolomics approaches.
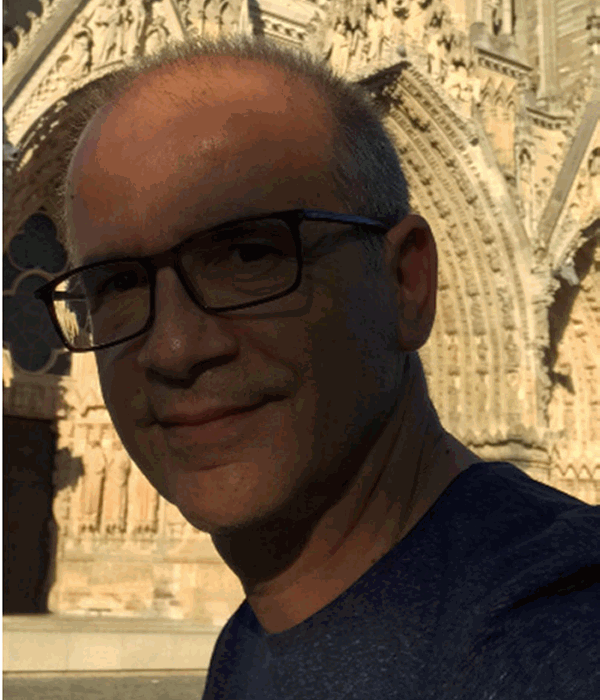
Jesús Mercado-Blanco
Jesús Mercado-Blanco has a PhD in Biological Sciences (University of Granada, Spain). He is senior researcher currently affiliated to the Zaidín Experimental Station (EEZ), belonging to the Spanish National Research Council (CSIC). His main research interests focus on agricultural microbiology, agro-biotechnology and the development of control tools within integrated disease management strategies, using Verticillium wilt of olive and Fusarium wilt of banana as study models. Specific research topics are the bases underlying plant-microbe/microbiome interactions using molecular and ‘-omic’ approaches and the identification, characterization, and use of microbiological control agents, with emphasis on bacterial endophytes.
References
- Al-Warhi T, Elmaidomy AH, Maher SA, Abu-Baih DH, Selim S, Albqmi M, et al. 2022. The wound-healing potential of Olea europaea L. cv. Arbequina leaves extract: an integrated in vitro, in silico, and in vivo investigation. Metabolites. 12:791. doi:10.3390/metabo12090791.
- Ayele AG, Wheeler TA, Dever JK. 2020. Impacts of Verticillium wilt on photosynthesis rate, lint production, and fiber quality of greenhouse-grown cotton (Gossypium hirsutum). Plants. 9:857. doi:10.3390/plants9070857.
- Báidez AG, Gómez P, Del Río JA, Ortuño A. 2006. Antifungal capacity of major phenolic compounds of Olea europaea L. against Phytophthora megasperma Drechsler and Cylindrocarpon destructans (Zinssm.) Scholten. Physiol Mol Plant Pathol. 69:224–229. doi:10.1016/j.pmpp.2007.05.001.
- Báidez AG, Gómez P, Del Río JA, Ortuño A. 2007. Dysfunctionality of the xylem in Olea europaea L. plants associated with the infection process by Verticillium dahliae Kleb. role of phenolic compounds in plant defense mechanism. J Agric Food Chem. 55:3373–3377. doi:10.1021/jf063166d.
- Boskou D. 2006. Chemistry and technology. In: Dimitrios Boskou editor. Olive oil. 2nd ed. New York: AOCS Publishing; p. 288. doi:10.4324/9781003040217.
- Bubici G, Cirulli M. 2012. Control of Verticillium wilt of olive by resistant rootstocks. Plant Soil. 352:363–376. doi:10.1007/s11104-011-1002-9.
- Cádiz-Gurrea MdlL, Pinto D, Delerue-Matos C, Rodrigues F. 2021. Olive fruit and leaf wastes as bioactive ingredients for cosmetics: a preliminary study. Antioxidants. 10:1–18. doi:10.3390/antiox10020245.
- Cardoni M, Gómez-Lama Cabanás C, Valverde-Corredor A, Villar R, Mercado-Blanco J. 2022a. Unveiling differences in root defense mechanisms between tolerant and susceptible olive cultivars to Verticillium dahliae. Front Plant Sci. 13:1–19. doi:10.3389/fpls.2022.863055.
- Cardoni M, Mercado-Blanco J, Villar R. 2021. Functional traits of olive varieties and their relationship with the tolerance level towards Verticillium wilt. Plants. 10: 1079. doi:10.3390/plants10061079.
- Cardoni M, Quero JL, Villar R, Mercado-Blanco J. 2022b. Physiological and structural responses of olive leaves related totolerance/susceptibility to Verticillium dahliae. Plants. 11:2302. doi:10.3390/plants11172302.
- Cayuela JA, Rada M, Rios JJ, Albi T, Guinda A. 2006. Changes in phenolic composition induced by Pseudomonas savastanoi pv. savastanoi infection in olive tree: presence of large amounts of verbascoside in nodules of tuberculosis disease. J Agric Food Chem. 54:5363–5368. doi:10.1021/jf060807w.
- Chung PY, Navaratnam P, Chung LY. 2011. Synergistic antimicrobial activity between pentacyclic triterpenoids and antibiotics against Staphylococcus aureus strains. Ann Clin Microbiol Antimicrob. 10:1–6. doi:10.1186/1476-0711-10-25.
- Del Río JA, Báidez AG, Botía JM, Ortuño A. 2003. Enhancement of phenolic compounds in olive plants (Olea europaea L.) and their influence on resistance against Phytophthora sp. Food Chem. 83:75–78. doi:10.1016/S0308-8146(03)00051-7.
- De Mendiburu F, Simon R. 2015. Agricolae – Ten years of an open source statistical tool for experiments in breeding, agriculture and biology. PeerJ Pre Prints. 3:0–17. doi:10.7287/peerj.preprints.1404.
- Deng J, Wang H, Mu X, He X, Zhao F, Meng Q. 2021. Advances in research on the preparation and biological activity of maslinic acid, in Mini reviews in medicinal chemistry. Sharjah, UAE: Bentham Science Publishers, 79–89.
- Dzubak P, Hajduch M, Vydra D, Hustova A, Kvasnica M, Biedermann D, et al. 2006. Pharmacological activities of natural triterpenoids and their therapeutic implications. Nat Prod Rep. 23:394–411. doi:10.1039/b515312n.
- Ferreira RB, Monteiro S, Freitas R, Santos CN, Chen Z, Batista LM, et al. 2007. The role of plant defence proteins in fungal pathogenesis. Mol Plant Pathol. 8:677–700. doi:10.1111/j.1364-3703.2007.00419.x.
- García-Ruiz GM, Trapero C, Del Río C, López-Escudero FJ. 2014. Evaluation of resistance of Spanish olive cultivars to Verticillium dahliae in inoculations conducted in greenhouse. Phytoparasitica. 42:205–212. doi:10.1007/s12600-013-0353-6.
- Gharbi Y, Barkallah M, Bouazizi E, Gdoura R, Triki MA. 2017. Differential biochemical and physiological responses of two olive cultivars differing by their susceptibility to the hemibiotrophic pathogen Verticillium dahliae. Physiol Mol Plant Pathol. 97:30–39. doi:10.1016/j.pmpp.2016.12.001.
- Gómez-Lama Cabanás C, Schilirò E, Valverde-Corredor A, Mercado-Blanco J. 2015. Systemic responses in a tolerant olive (Olea europaea L.) cultivar upon root colonization by the vascular pathogen Verticillium dahliae. Front Microbiol. 6:1–13. doi:10.3389/fmicb.2015.00928.
- Guinda Á, Rada M, Delgado T, Gutiérrez-Adánez P, Castellano JM. 2010. Pentacyclic triterpenoids from olive fruit and leaf. J Agric Food Chem. 58:9685–9691. doi:10.1021/jf102039t.
- Jäger S, Trojan H, Kopp T, Laszczyk MN, Scheffler A. 2009. Pentacyclic triterpene distribution in various plants, rich sources for a new group of multi-potent plant extracts. Molecules. 14:2016–2031. doi:10.3390/molecules14062016.
- Jamkhande PG, Pathan SK, Wadher SJ. 2016. In silico PASS analysis and determination of antimycobacterial, antifungal, and antioxidant efficacies of maslinic acid in an extract rich in pentacyclic triterpenoids. Int J Mycobacteriology. 5:417–425. doi:10.1016/j.ijmyco.2016.06.020.
- Johnson G, Schaal LA. 1952. Relation of chlorogenic acid to scab resistance in potatoes. Science. 80(115):627–629. doi:10.1126/science.115.2997.627.
- Juven B, Henis Y. 1970. Studies on the antimicrobial activity of ovotransferrin. J. Appl Bacteriol. 33:721–732.
- Kanakis P, Termentzi A, Michel T, Gikas E, Halabalaki M, Skaltsounis AL. 2013. From olive drupes to olive oil. An HPLC-orbitrap-based qualitative and quantitative exploration of olive key metabolites. Planta Med. 79:1576–1587. doi:10.1055/s-0033-1350823.
- Karygianni L, Cecere M, Argyropoulou A, Hellwig E, Skaltsounis AL, Wittmer A, et al. 2019. Compounds from Olea europaea and Pistacia lentiscus inhibit oral microbial growth. BMC Complement Altern Med. 19:1–10. doi:10.1186/s12906-019-2461-4.
- Kassambara A. 2016. Practical guide to principal component methods in R: PCA, M (CA), FAMD, MFA, HCPC, factoextra. Vol. 2. Sthda.
- Kolde R. 2018. Package pheatmap. R package 1.10.
- Kunej U, Mikulič-Petkovšek M, Radišek S, Štajner N. 2020. Changes in the phenolic compounds of hop (Humulus lupulus l.) induced by infection with Verticillium nonalfalfae, the causal agent of hop Verticillium wilt. Plants. 9:841. doi:10.3390/plants9070841.
- Kutchan T. 2001. Ecological arsenal and developmental dispatcher. The Paradigm of Secondary Metabolism. Plant Physiol. 125:58–60. doi:10.1104/pp.125.1.58
- Lattanzio V, Cardinali A. 2006. Role of phenolics in the resistance mechanisms of plants against fungal pathogens and insects. Phytochem Adv Res. 661:23–67.
- Leyva-Pérez MdlO, Jiménez-Ruiz J, Gómez-Lama Cabanás C, Valverde-Corredor A, Barroso JB, Luque F, et al. 2018. Tolerance of olive (Olea europaea) cv Frantoio to Verticillium dahliae relies on both basal and pathogen-induced differential transcriptomic responses. New Phytol. 217:671–686. doi:10.1111/nph.14833
- Li Y, Wang J, Li L, Song W, Li M, Hua X, et al. 2022. Natural products of pentacyclic triterpenoids: from discovery to heterologous biosynthesis. Nat Prod Rep. doi:10.1039/d2np00063f.
- Link K, Walker JC. 1933. The isolation of catechol from pigmented onion scales and its significance in relation to disease resistance in Onions. J Biol Chem. 100:2.
- Lins FSV, de Souza TA, Opretzka LCF, e Silva JPR, Pereira LCO, Abreu LS, et al. 2022. New pregnane glycosides from Mandevilla dardanoi and their anti-inflammatory activity. Molecules. 27:1–16. doi:10.3390/molecules27185992.
- Liu S, Liu H, Zhang L, Ma C, Abd El-Aty AM. 2022. Edible pentacyclic triterpenes: a review of their sources, bioactivities, bioavailability, self-assembly behavior, and emerging applications as functional delivery vehicles. Crit Rev Food Sci Nutr. 1–17. doi:10.1080/10408398.2022.2153238.
- López-Escudero FJ, Del Río C, Caballero JM, Blanco-López MA. 2004. Evaluation of olive cultivars for resistance to Verticillium dahliae. Eur J Plant Pathol. 110:79–85. doi:10.1023/B:EJPP.0000010150.08098.2d.
- López-Escudero FJ, Mercado-Blanco J. 2011. Verticillium wilt of olive: a case study to implement an integrated strategy to control a soil-borne pathogen. Plant Soil. 344:1–50. doi:10.1007/s11104-010-0629-2.
- Markakis EA, Tjamos SE, Antoniou PP, Roussos PA, Paplomatas EJ, Tjamos EC. 2010. Phenolic responses of resistant and susceptible olive cultivars induced by defoliating and non-defoliating Verticillium dahliae pathotypes. Plant Dis. 94:1156–1162. doi:10.1094/PDIS-94-9-1156.
- Marsilio V, Lanza B. 1998. Characterisation of an oleuropein degrading strain of Lactobacillus plantarum. Combined effects of compounds present in olive fermenting brines (phenols, glucose and NaCl) on bacterial activity. J Sci Food Agric. 76:520–524. doi:10.1002/(SICI)1097-0010(199804)76:4 <; 520::AID-JSFA982 > 3.0.CO;2-I.
- Mechri B, Tekaya M, Attia F, Hammami M, Chehab H. 2020. Drought stress improved the capacity of Rhizophagus irregularis for inducing the accumulation of oleuropein and mannitol in olive (Olea europaea) roots. Plant Physiol Biochem. 156:178–191. doi:10.1016/j.plaphy.2020.09.011.
- Mechri B, Tekaya M, Hammami M, Chehab H. 2019. Root verbascoside and oleuropein are potential indicators of drought resistance in olive trees (Olea europaea L.). Plant Physiol Biochem. 141:407–414. doi:10.1016/j.plaphy.2019.06.024.
- Michel T, Khlif I, Kanakis P, Termentzi A, Allouche N, Halabalaki M, et al. 2015. UHPLC-DAD-FLD and UHPLC-HRMS/MS based metabolic profiling and characterization of different Olea europaea organs of Koroneiki and Chetoui varieties. Phytochem Lett. 11:424–439. doi:10.1016/j.phytol.2014.12.020.
- Montes-Osuna N, Mercado-Blanco J. 2020. Verticillium wilt of olive and its control: what did we learn during the last decade? Plants. 9:735. doi:10.3390/plants9060735.
- Olmo-García L, Bajoub A, Fernández-Gutiérrez A, Carrasco-Pancorbo A. 2016. Evaluating the potential of LC coupled to three alternative detection systems (ESI-IT, APCI-TOF and DAD) for the targeted determination of triterpenic acids and dialcohols in olive tissues. Talanta. 150:355–366. doi:10.1016/j.talanta.2015.12.042.
- Olmo-García L, Carrasco-Pancorbo A. 2021. Chromatography-MS based metabolomics applied to the study of virgin olive oil bioactive compounds: characterization studies, agro-technological investigations and assessment of healthy properties. TrAC - Trends Anal Chem. 135:116153. doi:10.1016/j.trac.2020.116153.
- Olmo-García L, Kessler N, Neuweger H, Wendt K, Olmo-Peinado JM, Fernández-Gutiérrez A, et al. 2018. Unravelling the distribution of secondary metabolites in Olea europaea L.: exhaustive characterization of eight olive-tree derived matrices by complementary platforms (LC-ESI/APCI-MS and GC-APCI-MS). Molecules. 23:1–16. doi:10.3390/molecules23102419.
- Ortega-García F, Peragón J. 2009. The response of phenylalanine ammonia-lyase, polyphenol oxidase and phenols to cold stress in the olive tree (Olea europaea L. cv. Picual). J Sci Food Agric. 89:1565–1573. doi:10.1002/jsfa.3625.
- Ortega-García F, Peragón J. 2010. HPLC analysis of oleuropein, hydroxytyrosol, and tyrosol in stems and roots of Olea europaea L. cv. picual during ripening. J Sci Food Agric. 90:2295–2300. doi:10.1002/jsfa.4085.
- Petridis A, Therios I, Samouris G, Tananaki C. 2012. Salinity-induced changes in phenolic compounds in leaves and roots of four olive cultivars (Olea europaea L.) and their relationship to antioxidant activity. Environ Exp Bot. 79:37–43. doi:10.1016/j.envexpbot.2012.01.007.
- Pichersky E, Gang DR. 2000. Genetics and biochemistry of secondary metabolites in plants: An evolutionary perspective. Trends Plant Sci. 5:439–445. doi:10.1016/S1360-1385(00)01741-6.
- Ruiz-Barba JL, Garrido-Fernández A, Jiménez-Díaz R. 1991. Bactericidal action of oleuropein extracted from green olives against Lactobacillus plantarum. Lett Appl Microbiol. 12:65–68. doi:10.1111/j.1472-765X.1991.tb00505.x.
- Ryan D, Robards K. 1998. Phenolic compounds in olives. Analyst. 123:31–44. doi:10.1039/a708920a.
- Sabella E, Luvisi A, Aprile A, Negro C, Vergine M, Nicolì F, et al. 2018. Xylella fastidiosa induces differential expression of lignification related-genes and lignin accumulation in tolerant olive trees cv. Leccino. J Plant Physiol. 220:60–68. doi:10.1016/j.jplph.2017.10.007.
- Seepe HA, Ramakadi TG, Lebepe CM, Amoo SO, Nxumalo W. 2021. Antifungal activity of isolated compound from the leaves of Combretum erythrophyllum (Burch.) Sond. and Withania somnifera (L.) Dunal against Fusarium pathogens. Molecules. 26:4732.
- Serrano A, Rodríguez-Jurado D, López-Escudero FJ, Román B, Belaj A, De la Rosa R, Luque F, Ramírez-Tejero JA, León L. 2020. Verticillium wilt response of an olive core collection. Poster session presented at: The 7th international horticulture research conference; July 1–30; Northwest A&F University; China.
- Serrano A, Rodríguez-Jurado D, Ramírez-Tejero JA, Luque F, López-Escudero FJ, Belaj A, Román B, León L. 2023. Response to Verticillium dahliae infection in a genetically diverse set of olive cultivars. Sci Hortic. 316:112008. doi:10.1016/j.scienta.2023.112008
- Serrano-García I, Olmo-García L, Polo-Megías D, Serrano A, León L, de la Rosa R, et al. 2022. Fruit phenolic and triterpenic composition of progenies of Olea europaea subsp. cuspidata, an interesting phytochemical source to be included in olive breeding programs. Plants. 11:1791. doi:10.3390/plants11141791.
- Skodra C, Michailidis M, Dasenaki M, Ganopoulos I, Thomaidis NS, Tanou G, et al. 2021. Unraveling salt-responsive tissue-specific metabolic pathways in olive tree. Physiol Plant. 173:1643–1656.
- Sumner LW, Amberg A, Barrett D, Beale MH, Beger R, Daykin CA, et al. 2007. Proposed minimum reporting standards for chemical analysis: chemical analysis working group (CAWG) metabolomics standards initiative (MSI). Metabolomics. 3:211–221. doi:10.1007/s11306-007-0082-2.
- Tassou CC, Nychas GJE. 1994. Inhibition of Staphylococcus aureus by olive phenolics in broth and in a model food system. J Food Prot. 57:120–124. doi:10.4315/0362-028x-57.2.120.
- Termentzi A, Halabalaki M, Skaltsounis AL. 2015. From drupes to olive oil: an exploration of olive key metabolites. In: Olive and olive oil bioactive constituents. AOCS Press; p. 147–177. doi:10.1016/B978-1-63067-041-2.50012-4.
- Thatcher LF, Anderson JP, Singh KB. 2005. Plant defence responses: what have we learnt from Arabidopsis? Funct Plant Biol. 32:1–19. doi:10.1071/FP04135.
- Torres-Vega J, Gómez-Alonso S, Pérez-Navarro J, Alarcón-Enos J, Pastene-Navarrete E. 2021. Polyphenolic compounds extracted and purified from Buddleja globosa hope (Buddlejaceae) leaves using natural deep eutectic solvents and centrifugal partition chromatography. Molecules. 26:2192. doi:10.3390/molecules26082192.
- Trapero C, Alcántara E, Jiménez J, Amaro-Ventura MC, Romero J, Koopmann B, et al. 2018. Starch hydrolysis and vessel occlusion related to wilt symptoms in olive stems of susceptible cultivars infected by Verticillium dahliae. Front Plant Sci. 9:1–8. doi:10.3389/fpls.2018.00072.
- Trapero C, Rallo L, López-Escudero FJ, Barranco D, Díez CM. 2015. Variability and selection of Verticillium wilt resistant genotypes in cultivated olive and in the Olea genus. Plant Pathol. 64:890–900. doi:10.1111/ppa.12330.
- Trapero C, Serrano N, Arquero O, Del Río C, Trapero A, López-Escudero FJ. 2012. Field resistance to Verticillium wilt in selected olive cultivars grown in two naturally infested soils. Plant Dis. 97:668–674. doi:10.1094/PDIS-07-12-0654-RE.
- Tsror LL. 2011. Review: epidemiology and control of Verticillium wilt on olive. Isr J Plant Sci. 59:59–69. doi:10.1560/IJPS.59.1.59.
- Uccella N. 2000. Olive biophenols: biomolecular characterization, distribution and phytoalexin histochemical localization in the drupes. Trends Food Sci Technol. 11:9–10. doi: 10.1016/S0924-2244(01)00029-2
- Valverde P, Barranco D, López-Escudero FJ, Díez CM, Trapero C. 2023. Efficiency of breeding olives for resistance to Verticillium wilt. Front Plant Sci. 14:566.
- Valverde P, Trapero C, Arquero O, Serrano N, Barranco D, Muñoz Díez C, et al. 2021. Highly infested soils undermine the use of resistant olive rootstocks as a control method of Verticillium wilt. Plant Pathol. 70:144–153. doi:10.1111/ppa.13264.
- Wen JH, Zhao HF, Hong JH, He Y, Guang ZZ, Chang JJ, et al. 2011. Benzoic acid allopyranosides and lignan glycosides from the twigs of Keteleeria evelyniana. Zeitschrift fur Naturforsch. Sect B J Chem Sci. 66:733–739. doi:10.1515/znb-2011-0715.
- Zarco-Tejada PJ, Poblete T, Camino C, González-Dugo V, Calderón R, Hornero A, et al. 2021. Divergent abiotic spectral pathways unravel pathogen stress signals across species. Nat Commun. 12:1–11. doi:10.1038/s41467-021-26335-3.